ABSTRACT
Oxidative tissues such as brown adipose tissue and muscle internalize large amounts of circulating lipids and glucose as energy source. Endothelial cells (ECs) provide a platform for regulated transport and processing of blood-borne nutrients. Next to this role, it has become recognized that intercellular crosstalk between ECs and underlying parenchymal cells is indispensable for maintenance of tissue homoeostasis. Here, we comment on our recent observation that capillary ECs in thermogenic adipose tissues take up and metabolize entire triglyceride-rich lipoprotein (TRL) particles in response to cold exposure. This process is dependent on CD36, lipoprotein lipase (LPL) and lysosomal acid lipase (LAL). Remarkably, loss of LAL specifically in endothelial cells results in impaired endothelial proliferation and diminished thermogenic adaptation. Mechanistically, cell culture experiments indicate that LAL-mediated TRL processing leads to the generation of reactive oxygen species, which in turn activate hypoxia-induced factor (HIF)-mediated proliferative responses. In the current manuscript, we provide in vivo evidence that LAL-deficiency impairs proliferation of endothelial cells in thermogenic adipose tissue. In addition, we show uptake of nanoparticle-labelled TRL and LAL expression in cardiac endothelial cells, suggesting a physiological function of endothelial lipoprotein processing not only in thermogenic adipose tissue but also in cardiac muscle.
Thermogenic adipose tissue endothelial cells – controlling the fire
Brown adipocytes residing in brown adipose tissue (BAT) contribute to whole body energy expenditure and maintenance of euthermia. In addition, sustained cold exposure results in browning in white adipose tissues (WAT), a process characterized by the appearance of thermogenically active beige/brite adipocytes. For heat production, thermogenic brown and beige/brite adipocytes uncouple the electron transportation chain from oxidative phosphorylation using a unique mitochondrial protein, the uncoupling protein 1 (UCP1). Due to their high capacity for energy combustion, brown and beige/brite adipocytes can beneficially affect metabolic health in rodents and potentially in humans [Citation1]. Thermogenic adipocytes internalize substantial amounts of fuels – mainly lipids and glucose – to maintain tissue function and replenish intracellular energy stores [Citation2]. To ensure sufficient nutrient and oxygen supply, thermogenic adipose tissues are highly vascularized. In adipose tissue, the amount and density of ECs is crucial for tissue expansion and thermogenic adipose tissue browning [Citation3–6]. The continuous capillary endothelium forms a tight barrier between the circulation and parenchymal adipocytes, creating the first contact site for circulating nutrients. Therefore, fuelling of underlying cells requires efficient uptake, processing and potentially transendothelial transport of lipids and glucose by ECs. ECs express a variety of transporters, receptors, and lipases to take up and process nutrients. The shuttling of lipids to parenchymal cells of BAT is facilitated by a multistep process, in which the EC membrane acts as a platform for LPL-mediated processing of TRL. Subsequently, the liberated fatty acids (FAs) are taken up by transport proteins such as CD36 or FATP [Citation7–9]. In the classical view, the remaining TRL-remnants detach from the vascular endothelium, reach the liver via the circulation and are ultimately internalized by hepatocytes through lipoprotein receptor mediated endocytosis [Citation10]. Notably and in contrast to this classical pathway, substantial amounts of whole TRL remnant particles are cleared by activated thermogenic BAT and WAT [Citation2,Citation11–13], yet the physiological significance and regulation of whole-lipoprotein particle uptake remained elusive. Another source for FAs are intracellular triglycerides (TGs) stored in lipid droplets (LDs). In brown adipose tissue, cold exposure induces the hydrolysis of stored TGs by activation of lipases such as adipose triglyceride lipase (ATGL) and hormone-sensitive lipase (HSL) [Citation14]. BAT lipolysis was considered to be essential for regular thermogenic capacity and cold adaptation. In fact, whole body as well as adipose tissue-specific ATGL-deficiency in mice leads to impaired non-shivering thermogenesis [Citation15,Citation16]. However, recent studies showed that mice lacking ATGL or its co-activator comparative gene identification-58 (CGI-58) specifically in brown adipocytes displayed regular thermogenic function [Citation17,Citation18]. Similarly, HSL-deficient mice are not cold sensitive [Citation19,Citation20]. By brown adipocyte-specific deletion of acyl CoA:diacylglycerol acyltransferase (DGAT) enzymes, it was even shown recently, that TG storage in LDs generally is not required for regular non-shivering thermogenic adaptation [Citation21]. These data suggest that lipolysis of intracellular TG stores in brown adipocytes is dispensable for BAT-mediated cold-adaptation, as long as WAT-derived/circulating lipids or other fuels are available. This underlines an important role of whole TRL uptake in nutrient supply for thermogenic adipose tissues.
Lysosomal lipoprotein processing in endothelial cells determines thermogenic capacity of adipose tissue
Using nanoparticle labelling we recently discovered that entire TRL particles colocalize with endothelial cells in activated BAT [Citation22], pointing towards an active and regulated uptake process into these cells. Indeed, electron microscopy confirmed a sequential pathway, starting with initial attachment of TRL particles to the endothelial wall, followed by shrinkage and subsequently the appearance of the particles in intracellular vesicular organelles [Citation22]. These organelles were biochemically identified as representing the endosomal/lysosomal compartment [Citation22]. We thus hypothesized that entire TRL particles are hydrolysed in lysosomes to release lipid species that could in turn affect the metabolic activity of the underlying thermogenic adipocytes. The main triglyceride and cholesterol ester-hydrolysing enzyme in lysosomes is LAL [Citation23]. Magnetic isolation of endothelial cells confirmed high expression levels of LAL in endothelial cells of both BAT as well as WAT [Citation22]. Expression of LAL was found to be higher in ECs than in adipocytes and it was induced by cold activation, strengthening the hypothesis that endothelial LAL exerts an important physiological role in thermogenic adipose tissue. Another recent report described the uptake of chylomicrons into aortic ECs, in which the particles are internalized by ECs and subsequently hydrolysed in lysosomes under pathophysiological conditions. The authors of this paper propose a mechanism that contributes to lipid accumulation in ECs and co-cultured macrophages of mice lacking LPL [Citation24]. However, in contrast to our report this paper found CD36 and LPL to be dispensable for chylomicron uptake, and instead described a pathway involving the receptor SR-BI, a lipoprotein receptor best known for mediating transendothelial transport of high-density lipoproteins (HDL) into liver and adrenals [Citation25–28]. Generally, it is believed that EC forming large vessels are displaying different gene expression patterns than ECs lining small tissue-embedded capillaries [Citation29,Citation30]. In contrast to macrovascular VCAM1-positive ECs, microvascular cells express CD36, LPL and GPIHBP1 [Citation29–32]. Accordingly, differences in endothelial TRL uptake mechanisms are not surprising but might be necessary for homoeostatic tissue function. Both pathways, the one described in [Citation24] as well as in our recent report [Citation22] converge at the lysosome and imply an important functional role for lysosomal lipid processing.
We therefore generated inducible endothelial cell-specific LAL knockout mice and examined thermogenic adipose tissue function in these mice, which in response to cold exposure was significantly reduced [Citation22]. Depending on the duration of the acclimation protocol, we found both BAT as well as WAT thermogenic capacity to be strongly decreased. In addition to insufficient uptake of triglycerides as well as glucose, we observed lower levels of thermogenic marker expression as well as reduced appearance of beige/brite adipocytes. These findings implied a functional relationship between endothelial lipoprotein processing and the differentiation of the underlying parenchyma. Cold-induced recruitment of brown and beige/brite adipose tissues is accompanied by a strong induction of angiogenesis, the formation and sprouting of new blood vessels [Citation6]. We thus examined more closely the vascular bed in thermogenic adipose tissues of LAL-deficient mice in response to cold exposure. We found the expression of endothelial marker genes and proteins as well as vascular density to be significantly reduced [Citation22]. In line with this finding, we observed a strong induction of proliferation of cultured human HUVEC endothelial cells and adipocyte precursor cells in vitro following incubation with human TRL particles, an effect that was dependent on LAL action [Citation22]. Furthermore, we found that this effect was due to an activation of the HIF1a transcription factor through a beta oxidation-dependent stimulation of ROS production following TRL uptake. This TRL-LAL-ROS-HIF axis most likely contributes to the lipoprotein-mediated stimulation of proliferation.
LAL-deficiency impairs endothelial proliferation in vivo
In our recent paper, the question remained as to whether the proliferative effect of LAL-mediated TRL-processing in ECs is also relevant in vivo. Here we investigated endothelial cell proliferation in a dynamic manner during cold exposure using in vivo incorporation of the thymidine analogue EdU ()). Indeed, cold adaptation resulted in increased proliferation of EC. Moreover, in mice lacking LAL in endothelial cells we directly demonstrate that the proliferative responses in BAT endothelial cells were blunted in vivo ()). In line with our published data, we confirmed that LAL-mediated TRL processing is required for regular cold-induced endothelial proliferation. However, the direct link between endothelial proliferation and increased thermogenic recruitment is not fully understood. It is conceivable that endothelial proliferation and activity directly signals to adipocyte precursor cells residing in the vascular niche in mice [Citation33,Citation34] and humans [Citation35]. Moreover, endothelial cells have been shown to transdifferentiate into thermogenic adipocytes, providing another direct link between endothelial cell proliferation and the thermogenic recruitment of BAT and beige/brite WAT [Citation5]. Further lineage tracing and single-cell sequencing experiments will be needed to decipher which pathway is affected by LAL deficiency and how the endothelial lipid state is sensed by precursor cells residing in the same niche.
Figure 1. Endothelial cells proliferate in active BAT in a LAL-dependent manner
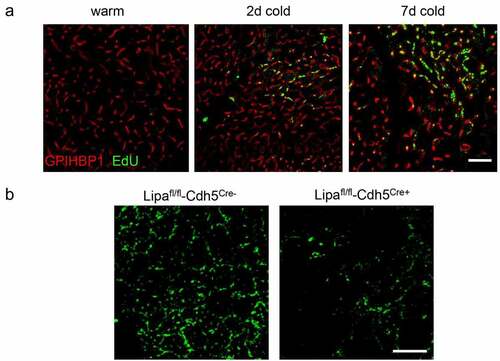
Future directions
We identified lysosomal TRL processing by endothelial cells as an important process for efficient activation and thermogenic adaptation of BAT and WAT. However, many other oxidative tissues also process TRLs to ensure sufficient fatty acid supply. Muscle tissues are highly vascularized and endothelial cells from these tissues show signatures of high nutrient clearance capacity [Citation36,Citation37]. This is illustrated by the high expression levels of lipid metabolism players such as GPIHBP1, LPL and CD36 in cardiac and skeletal muscles. Given that the uptake of entire TRL particles in BAT/WAT depends on LPL and CD36, it appeared likely that similar pathways might also be present in the skeletal and cardiac muscles. In our recent publication, it remained elusive whether ECs localized in other tissues than BAT/WAT contribute to reduced TRL particle uptake and impaired thermogenic capacity in endothelial-specific LAL-deficient mice [Citation22]. Here, using confocal microscopy following injection of QuantumDot-labelled TRL, we found the uptake of entire TRL particles both into endothelial cells of BAT ()) and heart ()). Furthermore, magnetic cell isolation experiments showed high enrichment of Gpihbp1 in CD31-positive fractions of BAT ()) and heart ()). Notably, expression of LAL-encoding Lipa mRNA was significantly higher in endothelial versus parenchymal cells isolated from BAT ()) and heart ()). While we did not find any obvious signs of cardiac impairment in endothelial cell-specific LAL knockout mice, their lower total energy expenditure and body temperature upon cold exposure could point towards metabolic abnormalities beyond BAT and beige/brite WAT function [Citation22]. Nevertheless, we found glucose and lipid uptake into heart and skeletal muscle to be unchanged in the endothelial cell-specific LAL knockout mice under conditions of warm or cold housing (unpublished results). Further studies using exercise regimens or models of cardiac impairment such as ischaemia-reperfusion may be used to shed light on the importance of TRL processing by endothelial LAL for (cardiac) muscle function.
Figure 2. Endothelial cells of BAT and cardiac muscle take up whole TRL particles, and ECs are equipped to process TRLs in lysosomes
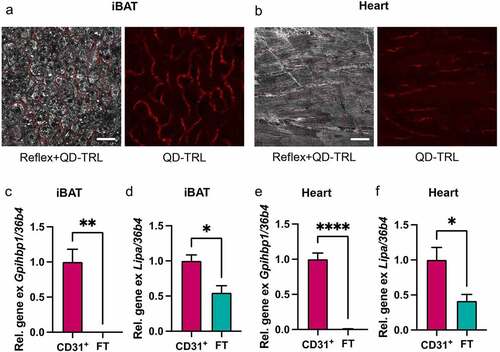
Furthermore, it remains to be established whether such pathway would be present and physiologically relevant in humans. Different levels of LAL deficiency result in distinct clinical manifestations [Citation38]. Direct analysis of transcriptional and functional changes in ECs of oxidative tissues from patients presenting with LAL-associated diseases may provide insight into the relevance of this pathway in the human disease context.
Author contribution
AWF and MYJ researched, analyzed data and prepared figures, all authors co-wrote the manuscript.
Disclosure statement
No potential conflict of interest was reported by the author(s).
Data availability statement
The data that support the findings of this study are openly available (DOI: 10.17632/xk9dtc7rw8.1) at Mendeley (https://data.mendeley.com).
Additional information
Funding
References
- Bartelt A, Heeren J. Adipose tissue browning and metabolic health. Nat Rev Endocrinol. 2014;10(1):24–36.
- Heine M, Fischer AW, Schlein C, et al. Lipolysis triggers a systemic insulin response essential for efficient energy replenishment of activated brown adipose tissue in mice. Cell Metab. 2018;28(4):644–655 e4.
- Han J, Lee JE, Jin J, et al. The spatiotemporal development of adipose tissue. Development. 2011;138(22):5027–5037.
- Luo X, Jia R, Luo XQ, et al. Cold exposure differentially stimulates angiogenesis in BAT and WAT of mice: implication in adrenergic activation. Cell Physiol Biochem. 2017;42(3):974–986.
- Tran KV, Gealekman O, Frontini A, et al. The vascular endothelium of the adipose tissue gives rise to both white and brown fat cells. Cell Metab. 2012;15(2):222–229.
- Xue Y, Petrovic N, Cao R, et al. Hypoxia-independent angiogenesis in adipose tissues during cold acclimation. Cell Metab. 2009;9(1):99–109.
- Abumrad N, Coburn C, Ibrahimi A. Membrane proteins implicated in long-chain fatty acid uptake by mammalian cells: CD36, FATP and FABPm. Biochim Biophys Acta. 1999;1441(1):4–13.
- Fischer AW, Cannon B, Nedergaard J. Leptin: is it thermogenic? Endocr Rev. 2020;41(2): 232–260.
- Hoeke G, Kooijman S, Boon MR, et al. Role of brown fat in lipoprotein metabolism and atherosclerosis. Circ Res. 2016;118(1):173–182.
- Heeren J, Scheja L. Metabolic-associated fatty liver disease and lipoprotein metabolism. Mol Metab. 2021;50:101238.
- Bartelt A, Bruns OT, Reimer R, et al. Brown adipose tissue activity controls triglyceride clearance. Nat Med. 2011;17(2):200–205.
- Khedoe PP, Hoeke G, Kooijman S, et al. Brown adipose tissue takes up plasma triglycerides mostly after lipolysis. J Lipid Res. 2015;56(1):51–59.
- Schlein C, Talukdar S, Heine M, et al. FGF21 lowers plasma triglycerides by accelerating lipoprotein catabolism in white and brown adipose tissues. Cell Metab. 2016;23(3):441–453.
- Zechner R, Zimmermann R, Eichmann TO, et al. FAT SIGNALS–lipases and lipolysis in lipid metabolism and signaling. Cell Metab. 2012;15(3):279–291.
- Ahmadian M, Abbott MJ, Tang T, et al. Desnutrin/ATGL is regulated by AMPK and is required for a brown adipose phenotype. Cell Metab. 2011;13(6):739–748.
- Haemmerle G, Lass A, Zimmermann R, et al. Defective lipolysis and altered energy metabolism in mice lacking adipose triglyceride lipase. Science. 2006;312(5774):734–737.
- Schreiber R, Diwoky C, Schoiswohl G, et al. Cold-induced thermogenesis depends on ATGL-mediated lipolysis in cardiac muscle, but not brown adipose tissue. Cell Metab. 2017;26(5):753–763 e7.
- Shin H, Ma Y, Chanturiya T, et al. Lipolysis in brown adipocytes is not essential for cold-induced thermogenesis in mice. Cell Metab. 2017;26(5):764–777 e5.
- Osuga J, Ishibashi S, Oka T, et al. Targeted disruption of hormone-sensitive lipase results in male sterility and adipocyte hypertrophy, but not in obesity. Proc Natl Acad Sci U S A. 2000;97(2):787–792.
- Wang SP, Laurin N, Himms-Hagen J, et al. The adipose tissue phenotype of hormone-sensitive lipase deficiency in mice. Obesity Res. 2001;9(2):119–128.
- Chitraju C, Fischer AW, Farese RV Jr., et al. Lipid droplets in brown adipose tissue are dispensable for cold-induced thermogenesis. Cell Rep. 2020;33(5):108348.
- Fischer AW, Jaeckstein MY, Gottschling K, et al. Lysosomal lipoprotein processing in endothelial cells stimulates adipose tissue thermogenic adaptation. Cell Metab. 2021;33(3):547–564 e7.
- Sheriff S, Du H, Grabowski GA. Characterization of lysosomal acid lipase by site-directed mutagenesis and heterologous expression. J Biol Chem. 1995;270(46):27766–27772.
- Cabodevilla AG, Tang S, Lee S, et al. Eruptive xanthoma model reveals endothelial cells internalize and metabolize chylomicrons, leading to extravascular triglyceride accumulation. J Clin Invest. 2021;131(12): e145800.
- Brundert M, Ewert A, Heeren J, et al. Scavenger receptor class B type I mediates the selective uptake of high-density lipoprotein-associated cholesteryl ester by the liver in mice. Arterioscler Thromb Vasc Biol. 2005;25(1):143–148.
- Jang E, Robert J, Rohrer L, et al. Transendothelial transport of lipoproteins. Atherosclerosis. 2020;315:111–125.
- Robert J, Osto E, Von Eckardstein A. The endothelium is both a target and a barrier of HDL’s protective functions. Cells. 2021;10(5):1041.
- Rohrer L, Ohnsorg PM, Lehner M, et al. High-density lipoprotein transport through aortic endothelial cells involves scavenger receptor BI and ATP-binding cassette transporter G1. Circ Res. 2009;104(10):1142–1150.
- Kalluri AS, Vellarikkal SK, Edelman ER, et al. Single-Cell analysis of the normal mouse aorta reveals functionally distinct endothelial cell populations. Circulation. 2019;140(2):147–163.
- Nolan DJ, Ginsberg M, Israely E, et al. Molecular signatures of tissue-specific microvascular endothelial cell heterogeneity in organ maintenance and regeneration. Dev Cell. 2013;26(2):204–219.
- Beigneux AP, Davies BS, Gin P, et al. Glycosylphosphatidylinositol-anchored high-density lipoprotein-binding protein 1 plays a critical role in the lipolytic processing of chylomicrons. Cell Metab. 2007;5(4):279–291.
- Davies BS, Beigneux AP, Barnes RH 2nd, et al. GPIHBP1 is responsible for the entry of lipoprotein lipase into capillaries. Cell Metab. 2010;12(1):42–52.
- Gupta RK, Mepani RJ, Kleiner S, et al. Zfp423 expression identifies committed preadipocytes and localizes to adipose endothelial and perivascular cells. Cell Metab. 2012;15(2):230–239.
- Tang W, Zeve D, Suh JM, et al. White fat progenitor cells reside in the adipose vasculature. Science. 2008;322(5901):583–586.
- Min SY, Kady J, Nam M, et al. Human ‘brite/beige’ adipocytes develop from capillary networks, and their implantation improves metabolic homeostasis in mice. Nat Med. 2016;22(3):312–318.
- Kalucka J, de Rooij L, Goveia J, et al. Single-Cell transcriptome atlas of murine endothelial cells. Cell. 2020;180(4):764–779 e20.
- Lother A, Bergemann S, Deng L, et al. Cardiac endothelial cell transcriptome. Arterioscler Thromb Vasc Biol. 2018;38(3):566–574.
- Pericleous M, Kelly C, Wang T, et al. Wolman’s disease and cholesteryl ester storage disorder: the phenotypic spectrum of lysosomal acid lipase deficiency. Lancet Gastroenterol Hepatol. 2017;2(9):670–679.
- Fischer AW, Schlein C, Cannon B, et al. Intact innervation is essential for diet-induced recruitment of brown adipose tissue. Am J Physiol Endocrinol Metab. 2019;316(3):E487–E503.
- Fischer AW, Shabalina IG, Mattsson CL, et al. UCP1 inhibition in Cidea-overexpressing mice is physiologically counteracted by brown adipose tissue hyperrecruitment. Am J Physiol Endocrinol Metab. 2017;312(1):E72–E87.