ABSTRACT
Aqueous zinc-based devices (AZDs) are considered as a promising candidate for next-generation energy storage due to high safety, economic benefit, and environmental benignity. However, the wide-spread application of AZD cathodes still faces challenges such as inferior energy density and cycling life. This manuscript focuses on the key design parameters for AZD cathodes and highlights the diverse functions of well-designed carbon architectures in AZD cathodes. The emerging opportunities are also presented to guide future endeavors towards high-performance AZD cathodes.
GRAPHICAL ABSTRACT
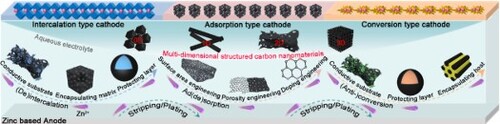
IMPACT STATEMENT
This manuscript is organized from the typical cathodic electrochemistry to discuss the progress and perspective of multi-dimensional structured nanocarbons for cathodes in Zinc-based energy devices.
1. Introduction
Employing aqueous rechargeable battery systems provides opportunities to eliminate the safety hazard and unaffordable cost issues associated with the commercial lithium-ion batteries (LIBs) [Citation1,Citation2]. The dominant aqueous solvent in the electrolyte effectively settles the security concern originating from the flammable and volatile aprotic electrolyte, while reducing the battery assembly cost. Additionally, the presence of water naturally roles out water- and air-sensitive raw alkaline metals as electrodes [Citation3,Citation4], and the multivalent metals emerge [Citation5]. Therefore, aqueous AZDs stand out from the numerous candidates. The motivation mainly stems from the high affordability (USD $2 kg−1 of Zn vs. $19 kg−1 of Li) [Citation6,Citation7], intrinsic abundance (almost five times as much as Li resources) [Citation8], low redox potential (−0.76 V vs. standard hydrogen electrode (SHE)) and high theoretical capacity (820 mAh g−1, 5851 mAh cm−3) of zinc metal [Citation5,Citation9–12]. The pure zinc metals directly acting as anode enables AZDs to eliminate the price of excess weight posed by electrochemically inactive components. However, the naked zinc in electrolytes suffers from heterogenous plating/stripping electrochemistry [Citation13,Citation14]. These are usually ascribed as the notorious dendrite formation and parasitic side reaction especially in the alkaline medium [Citation15]. At present, the near neutral or mild acidic (pH of 3.6–6.0) electrolytes (or with additives) optimized from the early alkaline ones are commonly used for AZDs owing to the suppressed byproducts and the alleviated dendrite formation [Citation16,Citation17].
The cathodes of AZDs determine holistic cathodic electrochemistry, thereby attracting even more attention. To date, there have been diverse but well-classified cathodes, including manganese- [Citation18–22], vanadium- [Citation23–27], prussian blue analogs (PBA)- [Citation28,Citation29], chalcogens- [Citation30–34], and carbon-based materials [Citation35–37], etc. The most essential difference among these cathodes lies on the ion storage mechanism, which can be categorized into intercalation-, adsorption-, conversion-type mechanism. Intercalation-type cathodes rely on the typical ion insertion/extraction, and usually suffer from inadequate specific capacities and unstable cyclability. These limit the energy density of AZDs to 50–300 Wh kg−1 [Citation18,Citation24,Citation29,Citation38,Citation39], which is even less than those of commercial LIBs (e.g. 387 Wh kg−1 for LiCoO2/Graphite battery) [Citation40]. The discrepancy between theoretical and practical values is attributed to the poor electrical conductivity, slow transfer kinetics, inferior structural stability caused by volumetric strain, and dissolution of active materials [Citation9,Citation41]. Introducing capacitive storage electrochemistry to cathode sides enables AZDs with high power capability and ultralong life-span, due to facile kinetics of ions adsorption/desorption absent of the bulk ions insertion/extraction [Citation42–45]. Adsorption-type electrodes are expected to have adequate specific surface area (SSA), rich microstructure as well as the synergy of Faradic/non-Faradic storage for both electrical double-layer capacitance (EDLC) and pseudocapacitance. These cathodes make the AZDs with capacitor characteristic in nature and improved power/cycling performances. However, capacity concerns still remain due to the energy density of even less than 200 Wh kg−1. On the other hand, conversion-type cathodes are promising to break capacity bottlenecks of AZDs, especially with the emergence of multi-electron transfer type cathodes (e.g. S, Se, Te). For instance, the two-electron transfer process of chalcogens-based cathodes delivers extremely high energy density over 700 Wh kg−1 (specific capacities over 1000 mAh g−1chalcogens) [Citation30–33,Citation46], which are superior to both intercalation- and adsorption-type cathodes. Nevertheless, these proposed promising cathodes still suffer from the restricted electron/ion transport and volume expansion as the intercalation-type cathodes, which lead to unsatisfactory capacity retention and cycling life.
Currently, the focus of performance challenges mainly lies on the architecture design of existing cathodes, e.g. nano technology [Citation47–49], defective engineering [Citation50,Citation51], and interlayer expansion [Citation48,Citation52,Citation53]. Carbon nanomaterials with abundant resources, adjustable morphology, and porosity, as well as graphitic microstructure, can adapt to different charge storage scenarios [Citation54–59]. Thus, building carbonaceous architecture is a feasible and promising approach to stimulate cathode for better performances. In this regard, lots of pioneering papers reveal the functionality of carbon nanomaterials in cathodes with various dimensions, e.g. 0D carbon quantum dots (QDs) [Citation37,Citation60–62], 1D carbon nanotubes (CNTs) [Citation25,Citation63–69] and carbon nanofibers (CNFs) [Citation39,Citation70–73], 2D graphene [Citation20,Citation74,Citation75] and carbon nanosheets (CNSs) [Citation76–78], and 3D hierarchical porous carbon (PC) [Citation79–82]. Figure (a) exhibits that the publication of AZDs has increased six-fold in the past decade, and nearly ten-fold for carbon-based AZDs. Carbon nanomaterials are reported to participate in host–guest electrochemistry in both intercalation and conversion-type materials as shown in Figure (b,c). Nanocarbons play an important role to bridge the gap of kinetics transfer and structural stability of active materials, resulting in improved rate capacities (∼10–100% increase at low rate, and ∼20–400% increase at high rate) and cyclability (∼10–300% increase). Furthermore, the selected carbons with special surface chemistry and microstructure can autonomously activate capacitive process as adsorption-type cathodes. Consequently, advanced carbonaceous architecture has captured a surge of interest and has been attempted in all three types of cathodes.
Figure 1. (a) Statistics of the number of publications from January 1st 2012 to September 1st 2022 by indexing the keywords of ‘zinc ion battery’ and ‘zinc ion battery and carbon’ in the Web of Science. (b) Microtopography of carbon composite in selected cases (Mn3O4/CDs: Reproduced with permission. Copyright 2022, Wiley-VCH [Citation60]. V2O5/CNFs: Reproduced with permission. Copyright 2022, Elsevier [Citation83]. Cu2O/GO: Reproduced with permission. Copyright 2022, Elsevier [Citation84]. KVO/SWCNTs: Reproduced with permission. Copyright 2020, American Chemical Society [Citation66]. NVO/G: Reproduced with permission. Copyright 2019, Elsevier [Citation23]. γ-MnO2/G: Reproduced with permission. Copyright 2020, Elsevier [Citation85]. α-MnO2/G: Reproduced with permission. Copyright 2020, American Chemical Society [Citation86]. VS4/rGO: Reproduced with permission. Copyright 2018, Royal Society of Chemistry [Citation87]. V2O5·H2O/PC: Reproduced with permission. Copyright 2021, Wiley-VCH [Citation88]. MnO2/HCS: Reproduced with permission. Copyright 2020, Royal Society of Chemistry [Citation89]. ZMO/PC: Reproduced with permission. Copyright 2022, Wiley-VCH [Citation21]. MnVO/PC: Reproduced with permission. Copyright 2021, Elsevier [Citation90]. (c) Rate and cycling performance comparison of the selected samples (as indicated in Figure (b)) with or without carbon assistance.
![Figure 1. (a) Statistics of the number of publications from January 1st 2012 to September 1st 2022 by indexing the keywords of ‘zinc ion battery’ and ‘zinc ion battery and carbon’ in the Web of Science. (b) Microtopography of carbon composite in selected cases (Mn3O4/CDs: Reproduced with permission. Copyright 2022, Wiley-VCH [Citation60]. V2O5/CNFs: Reproduced with permission. Copyright 2022, Elsevier [Citation83]. Cu2O/GO: Reproduced with permission. Copyright 2022, Elsevier [Citation84]. KVO/SWCNTs: Reproduced with permission. Copyright 2020, American Chemical Society [Citation66]. NVO/G: Reproduced with permission. Copyright 2019, Elsevier [Citation23]. γ-MnO2/G: Reproduced with permission. Copyright 2020, Elsevier [Citation85]. α-MnO2/G: Reproduced with permission. Copyright 2020, American Chemical Society [Citation86]. VS4/rGO: Reproduced with permission. Copyright 2018, Royal Society of Chemistry [Citation87]. V2O5·H2O/PC: Reproduced with permission. Copyright 2021, Wiley-VCH [Citation88]. MnO2/HCS: Reproduced with permission. Copyright 2020, Royal Society of Chemistry [Citation89]. ZMO/PC: Reproduced with permission. Copyright 2022, Wiley-VCH [Citation21]. MnVO/PC: Reproduced with permission. Copyright 2021, Elsevier [Citation90]. (c) Rate and cycling performance comparison of the selected samples (as indicated in Figure 1(b)) with or without carbon assistance.](/cms/asset/089ecf32-5a1f-4d95-8ba7-0d953a4dfd1b/tmrl_a_2178860_f0001_oc.jpg)
Despite the booming research on the carbons in AZDs, there are few comprehensive overviews covering from the storage models to the application of carbons in AZDs. In this review, we start with a critical discussion on the typical cathodic electrochemistry and discuss the key design parameters on high-performance cathodes. Then we outline the progress of multi-dimensional structured carbon nanomaterials in three types of cathodes. The functionality of carbon is discussed and the critical scientific/technical issues are identified in both non-all-carbon based intercalation- and conversion-type electrodes, and the all-carbon based adsorption-type electrodes. Finally, we conclude with perspectives and suggestions for advanced carbon designers towards high-performance AZDs.
2. Fundamental electrochemistry of cathodes
Cathodic electrochemistry is a descriptor for internal migration patterns of anions/cations upon charge–discharge processes. This descriptor distinctly conveys the electrode reaction route regarding structure evolution, discharging potential plateau, and electron transfer number of redox couples. Thus, the fundamental electrochemistry of the cathode is closely associated with overall energy/power-cyclability performances. Figuring out the influence of key parameters on performance and the original discrepancy of cathodic electrochemistry are an essential focus of the electrode architecture design. Here we first discuss the typical cathodic electrochemistry. The respective fundamental principles, and performances as well as the current issues for typical cathodes will be discussed in this part.
2.1. Intercalation-type electrochemistry
Figure (a) shows the typical configuration of intercalation-type electrochemistry in AZDs. The cathode undergoes Zn2+ intercalation into crystal and reduction of the associated elements upon discharge. The reversed deintercalation occurs with oxidation of the principal elements during charge. This storage mechanism in AZDs on the reversible Zn2+ insertion/extraction into/from MnO2 was first proposed in 2012 [Citation91]. Since then, a suite of in-depth and innovative studies of AZDs boomed in this decade. Thereinto, the advances of intercalation-type electrochemistry can be divided into four sub-categories: exclusive Zn2+ intercalation, H+ and Zn2+ co-intercalation, displacement- and hybrid-type intercalation. Currently, the electrochemistry of various sub-types motivated continuous enrichment of major cathode family for AZDs. The existing cathodes could be classified into several categories: Mn-based materials (e.g. MnO, MnO2, Mn2O3, MnO2-birnessite, ZnMn2O4) [Citation49,Citation92–96], V-based materials (e.g. VO2, V2O5, Zn2V2O7, NaV3O8·1.5H2O, Ag0.4V2O5) [Citation24,Citation74,Citation97–99], and PBA-based materials (e.g. KCuFeIII(CN)6) [Citation28].
Figure 2. Schematic illustration of the cell configurations, mechanisms and key parameters of the limiting factors for performance of AZDs based on zinc anode and (a) intercalation-, (b) adsorption-, (c) conversion-type cathodes. (d) A representative exhibition of specific capacities at low/high rates, maximum energy densities and cycling performances of various reported cathodes respectively based on intercalation- [Citation48,Citation94,Citation95,Citation116–122], adsorption- [Citation37,Citation61,Citation65,Citation70,Citation108,Citation123-127], and conversion-type electrochemistry as shown in Figure a–c [Citation31–34,Citation46,Citation103,Citation112,Citation113,Citation115,Citation128].
![Figure 2. Schematic illustration of the cell configurations, mechanisms and key parameters of the limiting factors for performance of AZDs based on zinc anode and (a) intercalation-, (b) adsorption-, (c) conversion-type cathodes. (d) A representative exhibition of specific capacities at low/high rates, maximum energy densities and cycling performances of various reported cathodes respectively based on intercalation- [Citation48,Citation94,Citation95,Citation116–122], adsorption- [Citation37,Citation61,Citation65,Citation70,Citation108,Citation123-127], and conversion-type electrochemistry as shown in Figure 2a–c [Citation31–34,Citation46,Citation103,Citation112,Citation113,Citation115,Citation128].](/cms/asset/0c384517-8e9c-42a0-ae71-34a6abf2584d/tmrl_a_2178860_f0002_oc.jpg)
However, the performances of those cathodes still fall behind expectations as exhibited in Figure (d). For instance, the Mn-based materials can deliver moderate capacities of 250–300 mAh g−1 associated with high discharge voltage (1.2–1.4 V vs. Zn2+/Zn) [Citation93], while the pursuit of high energy is harder for V- and PBA-based materials due to mutually exclusive access of capacity and discharge voltage (300–400 mAh g−1 and <1 V (vs. Zn2+/Zn) for V-oxides, <100 mAh g−1 and 1.5–1.8 V (Zn2+/Zn) for PBA) [Citation10]. To improve the electrochemical performances, we should start with identifying the key parameters of electrochemistry process and the drawbacks of existing cathodes. Firstly, the solvation shell formed outside of Zn2+ via Coulombic interaction enlarges the radius by more than five times (4.3 Å for [Zn(H2O)6]2+ vs. 0.74 Å for Zn2+) [Citation4,Citation9], which means more energy consumption is required to break ZnII-H2O bonds for Zn2+ intercalation. Additionally, the steric hindrance from continuous insertion results in unstable kinetics. The strong electrostatic interactions between the host and divalent Zn2+ further make the extraction harder in a reversible way. The above factors lead to decreased ion transfer rate and reduced cathode efficiency. Secondly, the oxides-based cathodes are troubled by electron transport efficiency as well. Metal oxides species (e.g. 10−5∼10−6 S cm−1 for MnO2 [Citation100], 10−2∼10−3 S cm−1 for V2O5 [Citation101]) exhibit unsatisfied electrical conductivity compared with highly conductive carbons (e.g. 106 S cm−1 for graphene [Citation7]). The sluggish electrochemical kinetics causes the low utilization of active materials, and consequently poor rate capacity and power performances. Thirdly, the ions insertion/extraction leads to volume variation of cathode material upon discharge/charge process. For instance, after exclusive Zn2+ insertion, γ-MnO2 composed of a tunnel structure was transformed to layered-type ZnyMnO2 with volume expansion of 9.21% [Citation102]. The drastic volumetric strain calls for structure robustness to maintain capacity during cycling. Fourthly, Mn2+ dissolution in the electrolyte causes irreversible capacity loss. Typical MnO2 working between 1 and 1.8 V (vs. Zn2+/Zn) prefer to exist stably as Mn2+ in a neutral or mild acidic system [Citation103,Citation104]. This explains that the formed Mn3+ species likely convert to Mn2+ via disproportionation reaction (2Mn3+ (s) → Mn4+ (s) + Mn2+ (aq)) [Citation103], thus causing Mn loss due to the high solubility of Mn2+ (70 g MnSO4 per 100 mL H2O at 7°C) [Citation16,Citation38]. The dissolved Mn2+ cannot participate in the sequent redox reduction, leading to irreversible capacity loss and short cycling life. To sum up, the intercalation-type electrochemistry has sparked many keen interests and greatly boosted the progress of cathodes. However, critical challenges need to be addressed for the pursuit of high-performance cathodes.
2.2. Adsorption-type electrochemistry
Figure (b) displays reversible ions adsorption/desorption in the adsorption-type electrochemistry. This mechanism in AZDs can be dated back to 2016 [Citation64], but carbonaceous materials have been skyrocketed since activated carbon (AC) was reported in 2018 [Citation61]. Ion storage based on this electrochemistry relies mainly on both physical and chemical models [Citation54,Citation105]. The physical model refers to the routine EDLC by physical electronic attraction. While the chemical adsorption/desorption of Zn2+/H+ generally activated by heteroatomic sites (e.g. O doped graphitic sites) on carbons, which provide extra pseudocapacitance [Citation106]. The adsorption-type electrochemistry relates to both cations and anions in the electrolyte. This dual-ions adsorption means that different charge carrier functions at respective regions which can be defined by zero charge potential. The cathodic electrochemistry below critical point is mainly attributed to cations, while the contribution of anions takes place at higher potential regions. These two models feature fast kinetics due to the absence of bulk insertion/extraction. Therefore, the AZDs assembled using carbon cathodes exhibit remarkable rate capability (>20 A g−1) and durability (over 10,000 cycles). The achieved power density of more than 30 kW kg−1 is superior to other types of cathodes. Regardless of the incomparable power density, even the state-of-the-art carbons deliver low energy density of less than 200 Wh kg−1 (specific capacities less than 300 mAh g−1) as shown in Figure (d).
Therefore, this inadequate energy density calls for more efficient and controllable carbons to boost performance. Firstly, a key criterion for estimating the availability of carbons is the active surface areas because it determines the charge accumulation of EDLC and pseudocapacitance [Citation54]. A proof example is that AC with the highest SSA delivers a higher capacity (1923 m2 g−1 for 121 mAh g−1) compared with counterparts with low SSA (e.g. 1408 m2 g−1 for 78 mAh g−1, 142 m2 g−1 for 9 mAh g−1) [Citation61]. Therefore, designing carbon cathodes with high SSA is beneficial for capacitive applications. Secondly, although the high SSA is helpful to improve capacity, no exact relationship was observed. Additionally, the calculated theoretical capacity values for carbons with SSA of 1000–3000 m2 g−1 can reach 300–500 F g−1 but the achieved experimental values are only 100–250 F g−1 [Citation42,Citation54]. This means that uncontrollable factors such as inaccessible pores, poor compatibility between electrolyte ions and pore sizes, and surface wettability make the attainable SSA far less than the theoretical value. Therefore, elaborately designed pore structure is quite necessary for easy access of ions accumulation and high SSA utilization. The focus should be on understanding the different roles of various nanopores in ion storage and optimizing the overall pore size distribution for rapid dynamics transfer [Citation107,Citation108]. Thirdly, well-established modification strategies (e.g. doping engineering [Citation106,Citation107,Citation109], chemical activation [Citation36,Citation110]) allow carbons with good surface functionality. Adequate sites that activate pseudocapacitance are used to compensate traditional EDLC, while the chemical adsorption relies on the affinity of approachable electrochemical sites with charge carriers. Therefore, adjusting the doping amount and species meanwhile amplifying the synergistic effect of each component require careful consideration. Fourthly, the capacity of the cathode should be as large as possible to match the paired zinc counterpart with high theoretical capacity. Thus, simultaneous increase of both capacity and tap density of active materials are required to achieve high energy density on the cell level. In brief, although the adsorption-type electrochemistry has made a great contribution to efficient cathodes, more and higher requirements for materials themselves are set to achieve high energy performances.
2.3. Conversion-type electrochemistry
Figure (c) shows the conversion-type electrochemistry in which the ions react with active materials to form a new substance upon discharge. In terms of conversion-type electrochemistry, the consecutive conversion reaction for ions storage is free from the rigid framework of host and saturation of active sites. This enables those cathodes to achieve high capacity. The electrochemistry of the conversion reaction can be distinguished as cationic- and anionic-redox. Cationic-redox refers to the oxidation/reduction of transition metal elements in Mn-based oxides (e.g. α-MnO2, Mn3O4) [Citation103,Citation111]. Anionic-redox mainly involves non-metallic elements (e.g. oxygen, nitrogen) in V-based compounds (e.g. VOPO4, VNxOy) [Citation112,Citation113]. Although single electron transfer type Mn- and V-based cathodes can hardly achieve any breakthrough in terms of performance, chalcogens-based cathodes with anionic-redox present an opportunity due to the multiple-electron transfer. The representatives of S and Se undergo a conversion reaction with two electrons transfer (S + Zn2+ + 2e- ↔ ZnS, Se + Zn2+ + 2e- ↔ ZnSe) [Citation31,Citation33,Citation114]. Another aqueous Zn-Te system was based on a two-step solid-to-solid conversion with the successive formation of ZnTe2 and ZnTe (2Te + Zn2+ + 2e- ↔ ZnTe2, ZnTe2 + Zn2+ + 2e- ↔ 2ZnSe) [Citation34]. These chalcogen-based cathodes experienced the similar electrochemical processes but different plateaus voltages and capacities. For both Zn-Te and Zn-S systems, the distinct voltage plateau is usually below 0.6 V vs. Zn2+/Zn [Citation31,Citation34], which is far less than the value of Zn-Se system (>1.1 V vs. Zn2+/Zn) [Citation33]. To date, the Zn-Te battery using bare Te nanosheets as cathodes delivered a capacity of 419 mAh g−1Te (241 Wh kg−1Te) [Citation34]. According to the Figure (d), the reported maximum capacities for Zn-S system with supporting of carbon host on cathode side were in the range of 800–1600 mAh g−1s (250–1100 Wh kg−1s) [Citation30–32,Citation114]. Meanwhile, a typical Zn-Se system assembled by CMK-3 encapsulated Se cathode delivered energy of 751 Wh kg−1Se (611 mAh−1Se) owing to the advantage of high voltage [Citation33].
Despite high capacities, the conversion-type cathodes suffer from poor cycle stability and rate performance, which requires more comprehensive investigation (Figure (d)). First of all, the poor electronic conductivity of chalcogens-based materials (e.g. 10−7 S cm−1 for S, 10−5 S cm−1 for Se, 2 S cm−1 for Te) necessitates the incorporation of conductive host such as carbon [Citation32,Citation34,Citation115]. The sluggish diffusion kinetics in the solid–solid reaction further exacerbated the poor power performance. The unsatisfied kinetics performance of both ions and electrons results in the underutilization of active materials. On the other hand, the negligible solubility constant of product roles out the dissolution issue (1.2 × 10−23 for ZnS, 3.6 × 10−26 for ZnSe in aqueous electrolyte) [Citation115]. However, the drastic volume changes (53% for S to ZnS, 62% for Se to ZnSe, 49% for Te to ZnTe) in conversion reactions still cause low efficiency and rapid capacity decay upon cycling. Generally, the conversion-type cathodes especially the emerging chalcogens hold great promise for improving the energy density of AZDs, but the existing intractable challenges deserve more in-depth considerations.
3. Carbon nanomaterials for cathode
Carbons, known as rich dimensionality and diversity of existence forms (using alone or in combination), are emerging as the promising materials in cathodes. The well-established processing technologies and theories allow the produced carbon with diverse properties, such as large SSA, abundant porosity, and functional surfaces. This makes nanocarbon suitable for applying in the existing cathodes, which is revealed by numerous pioneering works. Therefore, this section systematically summarizes the applications of multi-dimensional structured carbon nanomaterials (0D-3D) in three types of cathodes as shown in Figure . We first summarize recent works on carbons in the intercalation-type cathodes to construct the non-all-carbon based cathodes, followed by the design of all-carbon based cathodes in the adsorption-type cathodes. Finally, we overview non-all-carbon based conversion-type cathodes, with attention emphatically being given to chalcogen-based species.
Figure 3. An illustration showing vital roles of multi-dimensional structured carbon nanomaterials (0D-3D) for intercalation-, adsorption- and conversion-type cathodes application in AZDs.
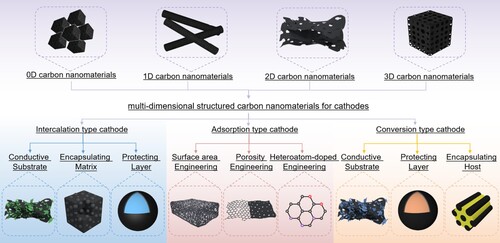
3.1. Carbon in intercalation-type cathode
The previously mentioned issues of intercalation-type cathodes could be well addressed by introducing functional carbon nanomaterials with controllable physicochemical properties and microtopography. Carbon can assist intercalation-type species to construct non-all-carbon based cathodes. Here we outline the progress of multi-dimensional structured carbons applied in intercalation-type cathodes in terms of specific functions of conductive substrate, encapsulating matrix and protecting layer.
3.1.1. Conductive substrate
Conductive substrate is the most economically and technically feasible function of carbons for intercalation-type cathodes due to the advantages of special micro-textures and functional surfaces. The non-all-carbon electrode design can facilitate efficient ion/electron transport and accommodate volume changes. To date, great progress has been made using this strategy.
0D carbon dots (CDs) of a few nanometers in size can serve as substrates with the structural merits of graphited cores and functional surfaces. In the example of Mn3O4/CDs composites, N-doped CDs-derived carbon skeleton was constructed as a conductive substrate [Citation60]. The carbon skeleton demonstrated good affinity to Mn species and guided uniform arrangement to eliminate the discontinuous aggregation according to the TEM images comparison in Figure (a,b). The native hydrophilic interface of substrate with heteroatomic site contributed greatly to immobilize the active species. Moreover, the hydrophilic groups and high SSA on carbon also improved wettability and conductivity of the cathode, leading to fast ion and electron kinetics. The electrochemical characterizations in Figure (c,d) validated that the Mn3O4/CDs exhibited a much smaller electrochemical resistance and higher rate capacities in contrast with pure Mn3O4.
Figure 4. Transmission electron microscopy (TEM) images of (a) Mn3O4/CDs and (b) pure Mn3O4. (c) Electrochemical impedance spectroscopy (EIS) and (d) rate properties of Mn3O4/CDs and pure Mn3O4. Reproduced with permission. Copyright 2022, WILEY-VCH [Citation60]. (e) Optical image of the free-standing cathode of amorphous MnO2/CNT. Reproduced with permission. Copyright 2020, Elsevier [Citation129]. The mechanical stability of (f) V2O5 nanoparticles/CNT, reproduced with permission, copyright 2019, Royal Society of Chemistry [Citation130], (g) V2O5 nanofibers/CNT, reproduced with permission, copyright 2020, Elsevier [Citation25], and (h) KVO nanobelts/CNT free-standing electrode, reproduced with permission, copyright 2020, American Chemical Society [Citation66]. (i) TEM image of ZnMn2O4/rGO and corresponded particle size distribution of ZnMn2O4. Reproduced with permission. Copyright 2020, Elsevier [Citation131]. (m) TEM image of ZnMn2O4/NG. Reproduced with permission. Copyright 2019, Elsevier [Citation132]. Scanning electron microscope (SEM) images of (j) MnO2 nanowires/rGO, reproduced with permission, copyright 2022, WILEY-VCH [Citation20], (n) H2V3O8 nanorods/graphene, reproduced with permission, copyright 2020, American Chemical Society [Citation133], and (o) H2V3O8 nanowires/graphene, reproduced with permission, copyright 2018, WILEY-VCH [Citation134]. (k) TEM image of 1D V2O5·H2O/graphene. Reproduced with permission. Copyright 2018, WILEY-VCH [Citation48]. Schematic illustration of the architectural frame structure of (l) 2D VO2 nanobelts/rGO, reproduced with permission, copyright 2021, Elsevier [Citation135], and (p) Od-VO2 nanobelts/rGO, reproduced with permission, copyright 2020, Elsevier [Citation136]. (q) SEM image of ZMO@PCPs. (r) Schematic diagram of electronic transport of ZMO@PCPs cathodes. Reproduced with permission. Copyright 2020, Elsevier [Citation137]. (s) Rate performances of MnO2/NHCSs and pure MnO2. Reproduced with permission. Copyright 2020, Royal Society of Chemistry [Citation89].
![Figure 4. Transmission electron microscopy (TEM) images of (a) Mn3O4/CDs and (b) pure Mn3O4. (c) Electrochemical impedance spectroscopy (EIS) and (d) rate properties of Mn3O4/CDs and pure Mn3O4. Reproduced with permission. Copyright 2022, WILEY-VCH [Citation60]. (e) Optical image of the free-standing cathode of amorphous MnO2/CNT. Reproduced with permission. Copyright 2020, Elsevier [Citation129]. The mechanical stability of (f) V2O5 nanoparticles/CNT, reproduced with permission, copyright 2019, Royal Society of Chemistry [Citation130], (g) V2O5 nanofibers/CNT, reproduced with permission, copyright 2020, Elsevier [Citation25], and (h) KVO nanobelts/CNT free-standing electrode, reproduced with permission, copyright 2020, American Chemical Society [Citation66]. (i) TEM image of ZnMn2O4/rGO and corresponded particle size distribution of ZnMn2O4. Reproduced with permission. Copyright 2020, Elsevier [Citation131]. (m) TEM image of ZnMn2O4/NG. Reproduced with permission. Copyright 2019, Elsevier [Citation132]. Scanning electron microscope (SEM) images of (j) MnO2 nanowires/rGO, reproduced with permission, copyright 2022, WILEY-VCH [Citation20], (n) H2V3O8 nanorods/graphene, reproduced with permission, copyright 2020, American Chemical Society [Citation133], and (o) H2V3O8 nanowires/graphene, reproduced with permission, copyright 2018, WILEY-VCH [Citation134]. (k) TEM image of 1D V2O5·H2O/graphene. Reproduced with permission. Copyright 2018, WILEY-VCH [Citation48]. Schematic illustration of the architectural frame structure of (l) 2D VO2 nanobelts/rGO, reproduced with permission, copyright 2021, Elsevier [Citation135], and (p) Od-VO2 nanobelts/rGO, reproduced with permission, copyright 2020, Elsevier [Citation136]. (q) SEM image of ZMO@PCPs. (r) Schematic diagram of electronic transport of ZMO@PCPs cathodes. Reproduced with permission. Copyright 2020, Elsevier [Citation137]. (s) Rate performances of MnO2/NHCSs and pure MnO2. Reproduced with permission. Copyright 2020, Royal Society of Chemistry [Citation89].](/cms/asset/764232e8-6bd8-432c-afcf-c81b10895bf6/tmrl_a_2178860_f0004_oc.jpg)
1D carbon nanomaterials possess a well-defined unidirectional spatial structure to carry active materials. For typical CNFs, a hierarchical core–shell structure of CNF@birnessite-MnO2 was reported in which MnO2 nanosheet shells were vertically aligned on CNF core [Citation71]. In this case, the hydrophobic surfaces of commercial CNFs are far less effective in anchoring active species than the N-doped carbon mentioned above. But an ingenious strategy proposed by the authors was pre-grinding CNFs with KMnO4 powder, which endows the CNFs with hydrophilicity and reactivity. The small MnOx nuclei or domains produced on the CNFs surface induced the growth of MnO2 tightly and uniformly [Citation71]. For substrate function, the key point are the firmness and denseness of anchoring sites. Careful consideration should be given to enhancing surface functionality of substrate because it is related to the loading mass and density as well as even the availability of substrates [Citation138]. Another advantage to employ 1D carbon nanomaterials is to fabricate free-standing electrodes. 1D carbon can act as both conductive agent and binding material to improve flexibility of the electrode and utilization of the active material. The unwanted cost and weight of metal current collectors are also eliminated in the free-standing electrodes [Citation107]. The CNT with 1D hollow structure acting as conductive substrate shows the advantage of this strategy [Citation101]. Interlaced 1D CNT monomers can be integrated into packed 3D macroscopic architectures, and the tight anchoring makes loaded species undergo a post-densified procedure to obtain free-standing sample. Furthermore, the binding effect from interconnected conductive skeleton not only alleviates aggregation and accommodates volume change, but also contributes to fast electron/ion transfer. Inspired by this, there are a number of intercalation-type materials that are assembled into free-standing electrodes with the support of CNT, such as amorphous MnO2 (Figure (e)) [Citation129], V2O5 nanoparticles (Figure (f)) [Citation130], V2O5 nanofibers (Figure (g)) [Citation25], and KV3O8·0.75H2O (KVO) nanobelts (Figure (h)) [Citation66]. Thereinto, simple vacuum filtering is often adopted as processing method. In fact, the solution formula for filtration habitually follows the slurry ratio in conventional electrode preparation. However, the mass ratio between active species with high capacity and carbon with high conductivity needs to be optimized to maximize the overall energy density without losing the free-standing structure.
2D carbon nanomaterials are also used as the conductive substrate, and the 2D spatial structure is more suitable for in-situ growth and arrangement of loaded species compared to the 1D carbon. Graphene, as a typical 2D carbon, can be used both in conventional and flexible substrates. In conventional substrates, 0D ZnMn2O4 nanoparticle/2D reduced graphene oxide (rGO) composite was proposed (denoted as ZnMn2O4/rGO). The ultrasmall ZnMn2O4 nanodots (5–10 nm) uniformly anchored on rGO without agglomeration were shown in Figure (i). In the resultant composite, the small active nanoparticle was in favor of exposing sites and shortening the diffusion path, while the rGO contributed to the large surface area and high electrical conductivity. Therefore, ZnMn2O4/rGO delivered higher capacity than the ZnMn2O4 microspheres [Citation131]. The particle size was also well defined at 21 nm in another case of ZnMn2O4/N-doped graphene (denoted as ZnMn2O4/NG) (Figure (m)). Moreover, lattice parameter quantitative calculation highlighted the weak distortion triggered by ion insertion/extraction in the hybrid electrode [Citation132]. This also shows the prominent contribution of conductive substrate in terms of both size and volume limitation. Additionally, 1D nanomaterials with various morphology were rationally designed as 1D/2D hybrid by integrating with graphene. The representative examples include MnO2 nanowires/rGO (Figure (j)) [Citation20], V2O5·nH2O nanowires/graphene (Figure (k)) [Citation48], H2V3O8 nanorods/graphene (Figure (n)) [Citation133], H2V3O8 nanowires/graphene (Figure (o)) [Citation134], etc. In these cases, the graphene substrates were constructed into 3D interconnected conductive framework. This design is beneficial to not just productively alleviating the buildup of active materials, but also facilitating the rapid transfer of electrons/ions. Therefore, those hybrid electrodes can perform well even at ultrahigh rates as shown in Table . In terms of 2D active species/graphene hybrid, the bright spot is the face-to-face contact form. For example, the layer-by-layer architecture of VO2 nanobelts/rGO [Citation135] and Od-VO2 nanobelts/rGO were shown in Figure (l,p) [Citation136]. Compared with point-to-face contact of 0D/2D and line-to-face contact of 1D/2D, the more intimately face-to-face contact guarantees fast kinetics and efficient control of volumetric strain. Simultaneously, dead volume was released and more sites were exposed because the stacking orientation was suppressed. For the flexible substrate, the vacuum filtration method is also effective for 2D graphene, such as in the case of MnO2 nanowires/rGO [Citation20]. In addition, the flexible engineering can be operated on deformable carbon skeleton where active material in situ grows on the surface [Citation139,Citation140]. For example, a flexible skeleton was constructed by vertical multilayer graphene arrays growing on carbon cloth (CC), which offered the reliable deformation adaptability for the loaded MnO2 [Citation140]. In addition to graphene, there are also well-designed artificial CNS as conductive substrates to construct non-all-carbon electrodes, such as V2O3/CNS (derived from pentyl viologen dibromide) with layer-by-layer structure [Citation27] and V2O5/CNS (derived from sodium citrate) with 2D sandwich structure [Citation88]. Furthermore, the non-graphene CNSs are no less impressive for flexible electrode preparation. Xia et al. prepared a flexible substate via growing N-doped CNSs arrays on CC to anchor active MnO2 [Citation141]. Although the vacuum filtration used by CNT and graphene are not universally applicable to the common composite materials, reasonable structural design, such as the tight loading on flexible CC, is also applicable in the free-standing electrodes.
Table 1. A summary of multi-dimensional structured carbon nanomaterials for intercalation-type cathodes.
3D carbon nanomaterials are another choice for conductive substrates. Many 1D and 2D carbon substrates prefer to exist in an interconnected network rather than standing alone. The 3D structure is more durable upon the frequent insertion/extraction. Moreover, the associated efficient mass transfer is also well-expected. 3D carbon with naturally interconnected framework can offer the possibility of high mass loading but without losing the ability to control the volume changes. Meanwhile, the abundant porosity permits easy accessibility of electrolyte ions to ensure high utilization. For instance, Zhao et al. have employed (metal–organic framework) MOF-derived porous carbon polyhedrons as 3D skeleton to anchor ZnMn2O4 nanoparticles (denoted as ZMO@PCPs) (Figure (q)) [Citation137]. As shown in Figure (r), the 3D conductive framework greatly promotes the efficient transport of electrons. Besides, MnO2 nanosheets grown on N-doped hollow carbon sphere (NHCS) also exhibited hollow structures in 3D skeleton construction [Citation89]. As shown in Figure (s), the rate performances of MnO2/NHCS were much higher than that of pure MnO2 hollow spheres. As for the flexible application, the above mentioned ZMO/PCPs composites were coated on carbon paper to prepare the electrode for assembling the flexible device. Such flexible post-treatment engineering can give deformable properties. However, this is quite different from 3D macroscopic stacking formed by vacuum filtration of CNT and graphene and in-situ growth on flexible CC, since the integrity and stability of the coated active material during operation need to be guaranteed to enable long cycling life.
3.1.2. Encapsulating matrix
Encapsulating matrix is another major function of carbon nanomaterials for intercalation-type cathodes, where the active ingredients are entirely embedded in the carbon. Going from cooperating with substrate to matrix, the intercalation-type species undergo a transition from the surface to the interior in the micro-space of carbons. However, this function transition still maintains or even improves the contribution ability of carbons to electrical conductivity and mass transfer because of the huge omnidirectional contact. Moreover, the embedment strategy not only isolates the electrolyte from the active species to relieve dissolution issue, but also enhances the constraint on volumetric strain. Here we summarize the progress of 1-3D structured carbon nanomaterials as encapsulating matrix for intercalation-type species.
For 1D CNFs, the electrospinning is the most common technology to construct the core–shell structure with the embedded active species. In this design, the porous carbon skeleton shell acts as conductive matrix to not only prevent active nanoparticles from contacting the electrolyte, but also restrict aggregation and unlimited volume expansion. In this regard, the mass ratio is more of a concern than surface functionality in this matrix function. For instance, Mn3O4 nanoparticles were encapsulated in hollow carbon fibers (HCFs) via coaxial electrospinning (denoted as Mn3O4@HCFs) [Citation22]. Polyacrylonitrile (PAN) precursors in electrospinning solutions were pre-designed with a series of concentration gradients to optimize the embedding effect. Mn3O4@HCFs with carbon content of 12.7 wt% did the best in encapsulating active nanoparticles and delivered most excellent performances. For another case of V2O3/N-doped carbon hybrid nanofibers (Figure (a)), the regulation of embedded species was achieved by the addition of different vanadium-containing precursors [Citation142]. The rate performance in Figure (b) indicated the optimized embedding amount of V2O3 was 85.3 wt% (denoted as S-VO85). CNFs can be woven into a dense stacking network by electrospinning to assemble into a free-standing film. For example, a flexible electrode consisting of carbon fiber cloth (CFC) and V2O5 was fabricated via electrospinning method (denoted as V2O5/CFC, Figure (c)) [Citation72]. By comparison of SEM images in Figure (c,d), the free-standing film exhibited good durability even after 100 cycles in which the overall structure with embedded V2O5 was well maintained. Bi-continues conductive network was also constructed using electrospinning approach [Citation39]. As shown in Figure (e), morphology observation showed that the shell was composed of the hollow fibers, while the core was contributed to the inner porous network. These built bi-continuous conductive paths and porous networks provided the encapsulated nanoparticles with rapid electrons/ions transfer. Moreover, the robust 1D hierarchical carbon matrix endowed composites with good pliability and high flexibility. The design can also be applied to embed both V2O5 and Zn2V2O7 nanoparticles (Figure (f,g)). The self-supported composite delivered better performances than the reference samples with naked metals oxides or replaced by activated carbon according to the rate performances in Figure (h).
Figure 5. (a) TEM image of V2O3/N-doped carbon. (b) Rate capability of V2O3/N-doped carbon electrodes at various current densities. Reproduced with permission. Copyright 2021, Elsevier [Citation142]. (c) SEM image of V2O5/CFC and digital photograph of free-standing electrode during the flexibility test (inserted). (d) SEM image of V2O5/CFC after the 100th cycle. Reproduced with permission. Copyright 2022, Elsevier [Citation72]. (e) Scheme of the hierarchical structure of the hybrid fibers. (f) SEM and (g) TEM images of the 1D hierarchical hybrid fibers with embedded Zn2V2O7 nanoparticles. (h) Comparisons of the discharge capacities between hybrid fibers and reference samples with naked metals oxides (MC) or replaced by activated carbon (PS) at different current densities. Reproduced with permission. Copyright 2019, American Chemical Society [Citation39]. (i) Schematic diagram of the synthesis steps of ZnMn2O4 and ZnMn2O4@C composites. (j) SEM image of ZnMn2O4@C and photographs of egg-waffle-like architecture (inserted). (k) Rate performance at different current densities and cycling performance of ZnMn2O4 and ZnMn2O4@C. Reproduced with permission. Copyright 2020, Elsevier [Citation143]. (l) Schematic illustration of fabrication process of ZMO QD@C. (m) Illustration of the Mn valence state distribution in ZMO QD@C and the mechanism of the structural stability of ZMO QD@C. Reproduced with permission. Copyright 2022, WILEY-VCH [Citation21].
![Figure 5. (a) TEM image of V2O3/N-doped carbon. (b) Rate capability of V2O3/N-doped carbon electrodes at various current densities. Reproduced with permission. Copyright 2021, Elsevier [Citation142]. (c) SEM image of V2O5/CFC and digital photograph of free-standing electrode during the flexibility test (inserted). (d) SEM image of V2O5/CFC after the 100th cycle. Reproduced with permission. Copyright 2022, Elsevier [Citation72]. (e) Scheme of the hierarchical structure of the hybrid fibers. (f) SEM and (g) TEM images of the 1D hierarchical hybrid fibers with embedded Zn2V2O7 nanoparticles. (h) Comparisons of the discharge capacities between hybrid fibers and reference samples with naked metals oxides (MC) or replaced by activated carbon (PS) at different current densities. Reproduced with permission. Copyright 2019, American Chemical Society [Citation39]. (i) Schematic diagram of the synthesis steps of ZnMn2O4 and ZnMn2O4@C composites. (j) SEM image of ZnMn2O4@C and photographs of egg-waffle-like architecture (inserted). (k) Rate performance at different current densities and cycling performance of ZnMn2O4 and ZnMn2O4@C. Reproduced with permission. Copyright 2020, Elsevier [Citation143]. (l) Schematic illustration of fabrication process of ZMO QD@C. (m) Illustration of the Mn valence state distribution in ZMO QD@C and the mechanism of the structural stability of ZMO QD@C. Reproduced with permission. Copyright 2022, WILEY-VCH [Citation21].](/cms/asset/1f08fa92-93e8-4b02-97cb-61782a801ab5/tmrl_a_2178860_f0005_oc.jpg)
Theoretically, 2D carbon nanomaterials could also be used as the encapsulating matrix, but the micron-thickness unfortunately limits its practical application. Currently, there are only a few reports on 2D encapsulating matrix. Figure (i) showed egg waffle-like architecture consisting of double-shell ZnMn2O4 hollow microspheres and CNS matrix (denoted as ZnMn2O4@C). SEM image in Figure (j) indicated that the ZnMn2O4 microspheres (1.5–3 µm in size) were evenly embedded or anchored in/on the carbon matrix. Additionally, the 2D carbon matrix with high conduction and porous networks was beneficial for the fast kinetics and structure stability upon long cycles. The intuitive values of the electrical conductivity and SSA of ZnMn2O4@C were far greater than those of pure ZnMn2O4 (1.96 S m−1 vs. 0.0284 S m−1, 109.1 m2 g−1 vs. 32.2 m2 g−1). Therefore, the ZnMn2O4@C delivered higher capacities of 364 mAh g−1 at 0.1 A g−1 and 127 mAh g−1 at 2 A g−1 compared to those of pure ZnMn2O4 (154 mAh g−1 and 67 mAh g−1) (Figure (k)). The remaining capacity of ZnMn2O4@C composite after 110 cycles was about 1.8 times that of the reference sample. CNS matrix with ZnMn2O4 uniformly embedded also showed good structural stability as revealed by post morphological characterization after 100 cycles [Citation143]. This egg waffle-like architecture sheds light on the design of 2D carbon matrix. A good match between the size of active species and the thickness of 2D carbon nanosheets is the key point to the successful operation. In addition, how to make full utilization of the flexible nature of the 2D matrix is also worth further exploration.
3D carbon nanomaterials as matrix can completely release the spatial limitations of embedding. A simple and feasible strategy for this is to induce the electrochemical transition of MOF-derived materials. For instance, ZnMn2O4 QDs were introduced in 3D porous carbon framework (denoted as ZMO QD@C) via electrochemically inducing MOF-derived Mn3O4/carbon hybrid (Figure l) [Citation21]. The 3D porous carbon matrix with ultrasmall embedded QDs (5.6 nm) helped to expose more active sites and brought fast transport dynamics. In addition, the spatial confinement effectively avoided particle aggregation. The formed Mn–O–C bond between QDs and matrix greatly strengthened the inherent stability by altering the electron configuration of Mn. Therefore, the dissolution issue can be effectively eliminated (Figure (m)). In the pure ZnSO4 electrolyte, a superior rate capacity (157.7 mAh g−1 at 1 A g−1) and cycle retention (86.4% retention after 1500 cycles) were delivered. A similar study on amorphous V2O5/3D carbon composite was reported [Citation144]. The amorphous V2O5 prepared from the MOF derived V2O3@C precursor favored isotropic diffusion paths and storage sites. The well-designed composite displayed extraordinary rate performance of 72.8 mAh g−1 at 200 A g−1 and record-breaking cyclability of 91.4% retention after 20,000 cycles at 40 A g−1 [Citation144].
3.1.3. Protecting layer
Protecting layer function refers to carbon nanomaterials exist as a thin shell outside the active species. Such a protecting layer further shields active components from direction contact with electrolytes. Meanwhile, it also reduces the risk of structure collapsing and underutilization due to volume changes and over-embedding, respectively. However, kinetic transport in the carbon layer even with ultrathin thicknesses still plays a key role in the electrochemical performance of the electrode. Since the micro-morphology of carbon layer largely depends on active components wrapped inside, the progress of the carbon protecting layer is summarized by the microscopic dimension (1D–3D) of the internal materials in this section.
1D nanomaterials could be enwrapped in center of carbon layer to build a typical coaxial core–shell structure (Figure (a)). For this purpose, graphene with good mechanical strength is appropriate for self-rolling outside the active fibers. For example, defect-rich V6O13-δ (Figure (b)) [Citation51] and α-MnO2 [Citation145] nanowires were coated with multi-layer graphene to form hybrid coaxial scrolls via time-controlled hydrothermal reactions. Per TEM images in Figure c, d, the overall diameter for coaxial core–shell structure of the two samples ranged from 50 to 250 nm and multi-layer graphene was in thickness of 5–10 nm. In the example of V6O13-δ, the time-dependent product evolution in the hydrothermal reaction was investigated. The orientated growth of nanowires, self-assembly, and self-rolling of graphene occurred sequentially as time increased. In addition to morphological evolution, the dosage of added graphene was controlled to set reference samples. The difference in performance between the optimized samples and pristine active species was plotted in Figure (e) to highlight the effectiveness of the graphene layer. For advanced carbon design, the structural parameters of protective layer are worth paying attention to, and more experimental parameters need to be introduced beyond the mass ratio of carbon.
Figure 6. (a, b) Schematic illustration of 1D hybrid scrolls with core-shell structure. Reproduced with permission. Copyright 2020, Elsevier [Citation51]. (c) TEM image of defect-rich V6O13-δ coated with multi-layer graphene. Reproduced with permission. Copyright 2020, Elsevier [Citation51]. (d) TEM image of α-MnO2 nanowires coated with multi-layer graphene. Reproduced with permission. Copyright 2018, WILEY-VCH [Citation145]. (e) Cycling performance at 0.3 A g−1 of α-MnO2 nanowires coated by graphene with different mass ratio. Reproduced with permission. Copyright 2018, WILEY-VCH [Citation145]. (f) TEM image of HAVO@G. (g) Cycling performances at 2 A g−1 of HAVO and HAVO@G. Reproduced with permission. Copyright 2019 Springer Nature [Citation146]. (h) Rate performances at different current densities and cycling performances of GO/CVO and pure CVO. Reproduced with permission. Copyright 2019, American Chemical Society [Citation147]. (i) TEM image of α-MnO2@C sample. (j) Galvanostatic charge-discharge (GCD) profiles of the pristine α-MnO2 and α-MnO2@C sample. Reproduced with permission. Copyright 2017, Elsevier [Citation148]. (k) TEM image of carbon-coated NaVPO4F sample. (l) Schematic illustration of the cell configuration and mechanism of aqueous zinc battery assembled using carbon-coated NaVPO4F cathode. Reproduced with permission. Copyright 2021, American Chemical Society [Citation149]. (m) TEM image of KMOH@C. (n) Schematic illustration of the Zn2+/electron transport in KMOH@C. (o) Rate capability at various current densities of KMOH@C and reference samples. Reproduced with permission. Copyright 2021, Elsevier [Citation150].
![Figure 6. (a, b) Schematic illustration of 1D hybrid scrolls with core-shell structure. Reproduced with permission. Copyright 2020, Elsevier [Citation51]. (c) TEM image of defect-rich V6O13-δ coated with multi-layer graphene. Reproduced with permission. Copyright 2020, Elsevier [Citation51]. (d) TEM image of α-MnO2 nanowires coated with multi-layer graphene. Reproduced with permission. Copyright 2018, WILEY-VCH [Citation145]. (e) Cycling performance at 0.3 A g−1 of α-MnO2 nanowires coated by graphene with different mass ratio. Reproduced with permission. Copyright 2018, WILEY-VCH [Citation145]. (f) TEM image of HAVO@G. (g) Cycling performances at 2 A g−1 of HAVO and HAVO@G. Reproduced with permission. Copyright 2019 Springer Nature [Citation146]. (h) Rate performances at different current densities and cycling performances of GO/CVO and pure CVO. Reproduced with permission. Copyright 2019, American Chemical Society [Citation147]. (i) TEM image of α-MnO2@C sample. (j) Galvanostatic charge-discharge (GCD) profiles of the pristine α-MnO2 and α-MnO2@C sample. Reproduced with permission. Copyright 2017, Elsevier [Citation148]. (k) TEM image of carbon-coated NaVPO4F sample. (l) Schematic illustration of the cell configuration and mechanism of aqueous zinc battery assembled using carbon-coated NaVPO4F cathode. Reproduced with permission. Copyright 2021, American Chemical Society [Citation149]. (m) TEM image of KMOH@C. (n) Schematic illustration of the Zn2+/electron transport in KMOH@C. (o) Rate capability at various current densities of KMOH@C and reference samples. Reproduced with permission. Copyright 2021, Elsevier [Citation150].](/cms/asset/c1eb559a-18de-41e0-b5be-6949d5b86e49/tmrl_a_2178860_f0006_oc.jpg)
2D nanomaterials with carbon layer covering are in the form of face-to-face contact to construct the hybrid layers. The tight attachment on both sides of 2D structured composite maximizes contact areas without sacrificing control over volume variation and dissolution issue. Thereby, this design guarantees structured stability and dynamic transport. There are relevant examples of graphene-wrapped H11Al2V6O23.2 nanobelt (denoted as HAVO@G) (Figure (f)) [Citation146] and graphene oxide -wrapped CuV2O6 nanobelts (denoted as GO/CVO) [Citation147]. In both cases, blank sample with carbon-free was set for comparison. For HAVO@G, it exhibited better reversible capacity of 280.2 mAh g−1 after 200 cycles, while pure HAVO underwent significant capacity fading due to the partial dissolution and poor conductivity (Figure (g)). But in the case of CuV2O6, the designer’s intention was to introduce GO with rich O-groups to enhance capacity. As expected, the GO/CVO sample achieved at least 30% of the capacity improvement compared with the blank sample as shown in Figure (h). This capacity increase can largely be attributed to the contribution of kinetic transport and conductivity by GO. The pseudocapacitance activated by O-groups may also contribute to the increased capacity, which needs to further clarification by systematically characterization. Besides graphene, other organic compounds-derived carbon can serve as protecting layer for 2D active materials including VO2 covered with polyethylene oxide-derived carbon [Citation151], carbon-coated V12O24·12H2O from hydrothermal treatment of ethanol and V2O5 solution [Citation152], and MnO2 coated with dopamine-derived carbon [Citation153]. For the last case, the carbon-coated MnO2 was further vertically grown on flexible CC for flexible application. Moreover, the anti-dissolution function of protecting layer was analyzed in detail. Thereinto, the measured Mn content in electrolyte of the carbon-coated sample after 50 cycles was about 4.70 µg mL−1, approximately 0.74 µg mL−1 lower than that of the unprotected sample.
3D nanomaterials covered with carbon layer are finally described. A typical sample is the carbon-coated α-MnO2 nanoparticles (denoted as α-MnO2@C) prepared via simple gel formation and subsequent annealing. Morphology characterization in Figure (i) showed that micro-spherical MnO2 particles with size of 20 nm were uniformly covered by carbon. The α-MnO2@C exhibited a higher capacity in pure ZnSO4 electrolyte compared to bare MnO2 (272 mAh g−1 vs. 213 mAh g−1) as shown in Figure (j), due to the effectiveness of the carbon layer to inhibit dissolution. In another example, chemical vapor deposition (CVD) was used to coat the pre-synthesized NaVPO4F with a uniform and clear carbon layer of 20 nm thick (Figure (k)). Moreover, the high concentration of electrolyte (15 M NaClO4 + 1 M ZnCF3SO3, Figure (l)) coordinated with carbon layer synergistically achieved the outstanding capacity retention of 94.5% after 4000 cycles. It can be observed that the above carbon layer design is based on a two-step method. In this procedure, the size control of pre-synthesized materials in the first step is of decisive significance. The active particles with well-defined size make the subsequent covering process easy, while the fine regulation of the carbon layer is still necessary. Another example showed a yolk–shell structured K-birnessite (K0.48Mn2O4·0.49H2O)@mesoporous carbon nanospheres (denoted as KMOH@C, Figure (m)). In this case, the outer carbon layer structure was of particular interest [Citation150], because the carbon shells regulated the transport of reaction ions by surface charge and pore structure to induce target yolk–shell structure. And the customized hollow carbon spheres as conductive framework ensured fast transfer of ions/electrons in the electrochemical process (Figure (n)). Furthermore, the abundant void space between internal core and outside shell can effectively adjust the volume strain of KMOH nanosheets. Therefore, the KMOH@C delivered a better rate and cycling performances at various currents, while the capacities of reference samples declined to unacceptable values quickly (Figure (o)). These examples show the efficient operation of construct protecting layer for 3D structured materials. Yet careful controlling and deliberate design of carbon layer structure as depicted in KMOH@C sample must be employed to avoid excessive protection and improve the utilization. Furthermore, a stable layer design with certain mechanical strength to avoid collapse is critical for durability.
3.2. Carbon in adsorption-type cathode
The adsorption-type cathodes mainly depend on the conventional EDLC at effective electrode interfaces and compensative Faradic pseudocapacitances occurred at functional groups. This means that the carbon cathodes are expected to feature with the large SSA to increase the exposure, rich heteroatomic groups to activate pseudocapacitance, and abundant porosity to facilitate ion transfer. Thereby, these typical features spontaneously become the accepted considerations for advanced carbon designs. In this section, we focus on the carbon design engineering of surface area, porosity. and heteroatom-doping. and summarize the progress of all-carbon based cathodes with different microscopic dimension (1D–3D).
3.2.1. Surface area engineering
A large surface area can contribute sufficient sites for both physical electrostatic attraction and Faradic chemical adsorption, thereby being regarded as the key parameter of carbon design. For typical 1D CNTs, the chemical oxidation treatment increased SSA from 120 to 211 m2 g−1, meanwhile enriched the surface oxygen content [Citation64]. These heteroatomic oxygen groups activated the chemical adsorption on oxidized CNTs to offer pseudocapacitances. The enlarged SSA not only provided more EDLC, but also exposed sufficient heteroatomic sites to ensure high utilization. Therefore, oxidized CNT delivered higher capacitances compared to the untreated sample [Citation64]. Except for the harsh chemical treatment mentioned above, surface area engineering can also be operated via the moderate alignment strategy. As an example, a carbon fiber composite consisting rGO and CNTs was assembled hydrothermally to increase SSA [Citation155]. Figure (a) gave the schematic illustration of the synthesis of rGO/CNT and the assembled Zn-ion hybrid fiber capacitor (ZnFC). In this design, the inserted single-walled CNTs among rGO nanosheets can effectively inhibit the completing rGO restacking, which enabled the rGO/CNT fibers with a sizeable accessible SSA (265 m2 g−1) for ion storage (Figure (b)). In comparison, the SSA of hydrothermally assembled pure rGO fibers without CNTs was only 24 m2 g−1. Moreover, the compact architecture endowed CNT/rGO fibers with outstanding self-supporting mechanical strength (127 MPa) that can satisfy the requirements for flexible wearable devices (Figure (c)). One of the advantages of fiber-shaped device is that they can be integrated into sophisticated energy storage units. Several as-assembled ZnFCs can be integrated in series or parallel to realize more extensive operating voltage windows or higher effective currents to meet the energy needs of the practical equipment. Thus, an energy storage unit was constructed by connecting five assembled ZnFCs in parallel first, and then integrating four of such assemblies in series. Thanks to the energy advantage brought by high SSA of the rGO/CNT electrode, this energy storage unit can power a light-emitting diode (LED) panel or a digital watch. Furthermore, the energy unit also showed good deformation adaptability under bending and twisting conditions, highlighting the great potential for flexible wearable devices.
Figure 7. (a) Schematic illustration of the synthesis of rGO/CNT hybrid fiber and Zn-coated graphite fiber for assembling a Zn-ion hybrid fiber capacitor. (b) N2 adsorption-desorption isotherm of rGO/CNT hybrid fiber. (c) The stress-strain curves of rGO/CNT fiber and Zn-coated/graphite fiber. Reproduced with permission. Copyright 2019, Wiley-VCH [Citation155]. (d) Schematic of the preparation of NHG-GO films from GO and NHG solutions with a tunable ratio of NHG to GO. (e) Cross-sectional SEM images of (f) rGO and 75%NHG-rGO films. (g) Tradeoff relationship between SSA and density of rGO and NHG-rGO films. Reproduced with permission. Copyright 2022, Wiley-VCH [Citation75]. (h) Schematic preparation, (i) N2 adsorption/desorption isotherms and (j) rate capability of PSC and PSC-Ax. Reproduced with permission. Copyright 2020, Elsevier [Citation127]. (k) N2 adsorption and desorption isotherms of HCS and SCN. Reproduced with permission. Copyright 2019, Royal Society of Chemistry [Citation79]. (l) The schematic illustrating the preparation procedure of the BGCs. (m) N2 adsorption and desorption isotherms of BGCs. Reproduced with permission. Copyright 2022 Springer Nature [Citation107].
![Figure 7. (a) Schematic illustration of the synthesis of rGO/CNT hybrid fiber and Zn-coated graphite fiber for assembling a Zn-ion hybrid fiber capacitor. (b) N2 adsorption-desorption isotherm of rGO/CNT hybrid fiber. (c) The stress-strain curves of rGO/CNT fiber and Zn-coated/graphite fiber. Reproduced with permission. Copyright 2019, Wiley-VCH [Citation155]. (d) Schematic of the preparation of NHG-GO films from GO and NHG solutions with a tunable ratio of NHG to GO. (e) Cross-sectional SEM images of (f) rGO and 75%NHG-rGO films. (g) Tradeoff relationship between SSA and density of rGO and NHG-rGO films. Reproduced with permission. Copyright 2022, Wiley-VCH [Citation75]. (h) Schematic preparation, (i) N2 adsorption/desorption isotherms and (j) rate capability of PSC and PSC-Ax. Reproduced with permission. Copyright 2020, Elsevier [Citation127]. (k) N2 adsorption and desorption isotherms of HCS and SCN. Reproduced with permission. Copyright 2019, Royal Society of Chemistry [Citation79]. (l) The schematic illustrating the preparation procedure of the BGCs. (m) N2 adsorption and desorption isotherms of BGCs. Reproduced with permission. Copyright 2022 Springer Nature [Citation107].](/cms/asset/a711f079-d30a-4262-9919-26321425e871/tmrl_a_2178860_f0007_oc.jpg)
2D carbon materials could also be carried out by alignment strategy for surface area engineering [Citation75]. Referring to the case in Figure (d), GO was reduced thermally by the hydrazine hydrate/ammonia to act as interlayer mediator (named as N2H4-Graphene, NHG). This mediator exhibited typically reduction feature and crumple geometric configuration. Therefore, the interspacing of laminate graphene films was extended via tailoring ratio of crumple NHG mediator. The resulting interlay spacing could be customized in range of 0.72–0.81 nm (Figure (e–f)), thereby facilitating the transfer kinetics of hydrated Zn2+ with large size. Furthermore, as shown in Figure (g), the interlayer expansion by regulating the ratio of NHG made the SSA increase from 12.5 to 325.1 m2 g−1, and the corresponding packing density decrease from 1.58 to 1.0 g cm−3. Combined with electrolyte engineering to tailor the solvation structure of Zn2+, the optimized sample showed a remarkable cyclability of 93.9% retention over 100,000 cycles and a preeminent volumetric capacitance of 235.4 F cm−3.
3D carbon nanomaterials are usually operated via chemical activation for SSA engineering. Precursors are usually selected carefully because of their direct association with the final product. For example, a hydrothermal-assisted biomass precursor mixing strategy with chemical activation was proposed [Citation110]. The as-obtained carbon integrates both advantages of SSA and conductivity of carbons derived from bagasse and coconut shell precursor. Therefore, the resultant carbon delivered higher rate capacitances than the carbon derived from a single precursor. Another wood-based pencil shavings biomass was employed as a precursor to study the effect of pyrolysis temperature on carbon during KOH activation (Figure (h)) [Citation127]. Figure i showed the N2 adsorption/desorption isotherms of carbons activated at different temperatures. The sample at 600°C (denoted as PSC-A600) with moderate SSA (948 m2 g−1) but stable porous structure delivered better rate performances than the under-activated sample at 500°C (598 m2 g−1) and over-activated sample at 700°C (1293 m2 g−1) (Figure (j)). This also indicated that SSA is not the only dominant factor of capacity. In addition, Liu et al. have investigated the effect of activator dosage by means of phenanthrene molecules precursor. The performance comparison showed that the optimized sample with moderate dosage of activator exhibited the highest SSA and best performances [Citation156]. Employing excessive activator addition or over-temperature carbonization to pursue high SSA at the expense of stable structure are inefficient and counter–productive. Meanwhile, an ultrahigh SSA and low activator dosage are mutually exclusive and intractable to achieve simultaneously in traditional activation process. A recent report indicated that a liquid–liquid micro-mixing strategy of activator and precursor can endow carbon with higher SSA using extremely low dosage of KOH (mass ratio of 0.42:1). Compared with the grinding-assisted solid–solid mixing technique, it achieved homogeneous mixing between precursor and activator at the microscopic level, thereby leading to a higher activation efficiency. The feasibility of this facile strategy was well-verified by operating on both precursors of silkworm cocoon and mushroom stalk [Citation157]. Apart from the single activator, there is also report on the synergistic effect of dual activators to obtain high SSA [Citation158]. The concomitant requirement is that the activator species and formulations need to be carefully decided. Another strategy for SSA engineering is the template approach. For example, a 3D HCSs (denoted as HCS) were fabricated by carbonizing polyaniline-co-polypyrrole employing a surfactant as the soft template, which exhibited a high SSA of 819.5 m2 g−1, far higher than that of the free-template (246.7 m2 g−1, denoted as SCN) with solid nanoparticle morphology [Citation79] (Figure (k)). Furthermore, according to our previous experience [Citation107], the templating/activating co-assisted carbonization processing strategy also helped us transform a routine protein-rich biomass to 3D carbon (denoted as BGCs) with ultrahigh SSA of 3657.5 m2 g−1 (Figure (l,m)).
Although it is believed that high SSA is beneficial for electrochemical performances, there are many samples (as listed in Tables –) with similar SSA delivered vastly different capacitances, even low SSA for higher performances. The fruitful surface area engineering also indicates that both SSA and electrochemical performances are affected by multiple factors. Therefore, pursuing high SSA meanwhile giving a comprehensive consideration to the overall structure of carbon are meaningful to boost performances.
Table 2. Radius and hydrated radius of representative electrolyte ions.
Table 3. A summary of multi-dimensional structured carbon nanomaterials for adsorption-type cathodes.
3.2.2. Porosity engineering
Although the pursuit of high SSA can be satisfied in various nanocarbons, the internal pore structure greatly affects the practical utilization of SSA. Tortuous paths in the interconnected nanopores make the inside materials easily shielded by the external part, which inevitably introduces invalid SSA. Advanced nanostructure design for high performances should be characterized by appropriate SSA cooperating with well-developed porosity. Both porosity and SSA engineering are closely related to each other. Here we are going to discuss the advanced design porosity engineering of multi-dimensional structured carbon nanomaterials.
Generally accepted classification of nanopores includes micropore (<2 nm), mesopore (2–50 nm), and macropore (>50 nm). In practice, the pore size distribution of the prepared carbon is usually not precisely concentrated in a range. A hierarchical architecture with various nanopores in carbon nanomaterials is common. For example, a designed B, N dual-doped 1D carbon microtubes (denoted as BN-CMT) were prepared using polypyrrole as precursor and H3BO3 as salt assistant (Figure (a)) [Citation159]. The N2 adsorption/desorption isotherm of BN-CMT in Figure (b) showed typical II and IV curves, indicating the existence of mesopores and macropores. The macropores can be attributed to the hollow structures, while the mesopore should be related to the activation effect. Moreover, the salt assistant can play dual roles of chemical activator and B dopant, which contributed to both the increase of SSA and the introduction of B-doping (Figure (c,d)). In the other case of 1D carbon, a surface engineering strategy based on two-step activation was employed to design hierarchically porous structure on fibrous carbon surface [Citation160]. The first steam-activated carbon fiber (denoted as MPCF) showed a micropore-dominated pore structure. Further chemical activation endowed the carbon fiber (denoted as HPCF) with hierarchical porous structure consisting of micropores, mesopores and macropores, and larger SSA. The discharge capacity of HPCF cathode was higher than that of MPCF at low rate (141 vs. 85 mAh g−1 at 0.1 A g−1) associated with the advantage of SSA (2000 vs. 810 m2 g−1). The MPCF sample underwent a dramatical decrease in the capacity at high rate, while that of HPCF still remained at a relatively high level. This is because the micropores are more favorable to charge adsorption in capacitors due to their contribution to the active site, and the meso-/macropores can guarantee a superior rate capacity by serving as ions reservoir to shorten transport path [Citation161].
Figure 8. (a) TEM image of BN-CMT. (b) PSD curves, (c) N2 adsorption/desorption isotherms and (d) X-ray photoelectron spectroscopy (XPS) survey spectra of N doped and B, N doped carbon microtube. Reproduced with permission. Copyright 2019, Royal Society of Chemistry [Citation159]. (e) The formation mechanism of porous CNSs based on Zn(NO3)2 and urea. (f) PSD curve of poplar wood powder derived CNSs. Reproduced with permission. Copyright 2021, Elsevier [Citation164]. (g) PSD curve of polyvinylpyrrolidone derived CNSs. Reproduced with permission. Copyright 2022, WILEY-VCH [Citation165]. (h) Schematics of the step-by-step fabrication process for the MSAC. (i) High-magnification SEM image of MSAC-1. (j) Pore size distribution (PSD) curve of MSACs and AC samples. Reproduced with permission. Copyright 2020, Elsevier [Citation37]. (k, l) PSD curves and (n) rate performances of PZC-Ax and reference samples. (m) Schematic illustration for the transportation of ions and electrons. Reproduced with permission. Copyright 2021, Elsevier [Citation126].
![Figure 8. (a) TEM image of BN-CMT. (b) PSD curves, (c) N2 adsorption/desorption isotherms and (d) X-ray photoelectron spectroscopy (XPS) survey spectra of N doped and B, N doped carbon microtube. Reproduced with permission. Copyright 2019, Royal Society of Chemistry [Citation159]. (e) The formation mechanism of porous CNSs based on Zn(NO3)2 and urea. (f) PSD curve of poplar wood powder derived CNSs. Reproduced with permission. Copyright 2021, Elsevier [Citation164]. (g) PSD curve of polyvinylpyrrolidone derived CNSs. Reproduced with permission. Copyright 2022, WILEY-VCH [Citation165]. (h) Schematics of the step-by-step fabrication process for the MSAC. (i) High-magnification SEM image of MSAC-1. (j) Pore size distribution (PSD) curve of MSACs and AC samples. Reproduced with permission. Copyright 2020, Elsevier [Citation37]. (k, l) PSD curves and (n) rate performances of PZC-Ax and reference samples. (m) Schematic illustration for the transportation of ions and electrons. Reproduced with permission. Copyright 2021, Elsevier [Citation126].](/cms/asset/45057607-6024-40f7-a801-0d35e6a0a06b/tmrl_a_2178860_f0008_oc.jpg)
In an example of 2D carbon nanomaterials, sodium polyacrylate was transformed into carbon nanoflakes using KHCO3 as activator. In this case, optimizing the dosage of activator can not only induce the formation of 2D morphology, but also effectively adjust the pore size distribution [Citation162]. KHCO3 activator with low content was mainly associated with micropores, while more mesopores were generated by a higher amount of activator. The optimized sample with well-developed nanoflakes morphology, appropriate mesopore proportions (42.4%), large pore volume, and SSA achieved the best performances. A similar phenomenon was observed in starch-derived 2D CNSs using KNO3 assisted KOH activation strategy. The suitable ratio of micropores/mesopores resulted from the moderate amount of KNO3 promoted transfer dynamic and ensured a large SSA [Citation163]. Therefore, a well-balanced pore distribution in stable hierarchical structure is critical for the efficient coordination of ion transport and storage. The high activity and strong etching effect of activators are admirable for pore engineering, but the precise control of pore size is insufficient. Thereby, the salt-assisted template method is more advantageous. For instance, the zinc nitrate-assisted pyrolysis strategy was employed to transform biomass precursor into porous 2D CNSs [Citation164,Citation165]. As shown in Figure (e), the redox reaction between zinc nitrate and urea additives released a lot of high-temperature gases to induce sheet morphology. Moreover, in-situ generated zinc species (e.g. ZnO and metal Zn) from zinc nitrate can penetrate and diffuse into the carbon matrix. The removal of these template species produced abundant nanopores with defined sizes in the resultant CNSs. The ammonia gas from urea and oxygen species from precursor were associated with the N, O dual-doped structure. Thereby, the poplar wood and polyvinylpyrrolidone (PVP) precursors derived CNSs by this strategy possessed hierarchically porous structure, which was consisted of micropores and abundant mesopores (Figure (f,g)).
In terms of 3D carbon nanomaterials, AC as a typical micropore-dominated nanocarbon has already showed the availability for adsorption-type cathode [Citation61]. However, the micropore-enriched nanostructure is also subject to drawbacks of deficient ion diffusion and poor wettability, which leads to rapid capacity and life fading at high rates. As shown in Figure (h), a PVP coating-assisted dehydrogenation process was proposed to synthesize mesoporous structured AC (denoted as MSAC) [Citation37]. The aggregated PVP on the AC consumed partial carbon during dehydrogenation to increase the pore size (see SEM image in Figure (b)). The MSAC showed higher fraction of mesopores (51% vs. 7%) (Figure (j)) and oxygen groups (47 wt% vs. 7 wt%) compared to the microporous structured AC, which improved ion diffusion efficiency and surface wettability. Thereby, MSAC delivered an ultra-long life of over 40,000 cycles with capacity retention of 78%. In addition, the 3D N-doped carbon nanocages (denoted as PZC-Ax) were fabricated via carbonizing and activating polypyrrole on the supporting platform of ZIF-8. This resultant hierarchical porous structure synchronously integrated micropores, mesopores, and macropores (Figure (k)) [Citation126]. Specifically, macropores were mainly generated by confinement pyrolysis of the ZIF-8, while the micropores and mesopores can be attributed to KOH activation and precursor carbonization. Generally, the macropores can be regarded as ions buffering reservoirs and transportation channels for facilitating electrolyte into the smaller pores. Mesopores are notable for providing rapid ion transport paths, whereas micropores donate large accessible SSA to expose sufficient active sites, thus playing a crucial role in ion storage. In addition, it can be observed that the micropore sizes of the obtained PZC samples were concentrated at 0.76, 0.8, and 0.84 nm (see zoom-in PSD curves in Figure (l)). Gogotsi and Simon argued that the pore size being optimized close to the ion diameters could endow the EDLC with an anomalous increase [Citation166]. Thereby the expected micropores are modulated to exceed 0.8 nm in view of the diameter of hydrated Zn2+ (0.86 nm) [Citation43,Citation127]. Consequently, the specific capacities of optimized PZC sample were up to 124 mAh g−1 and 81.7 mAh g−1 at 0.25 and 20 A g−1 (Figure (n)) benefited from the 3D hierarchical framework architecture. Despite the hierarchical porous structure with several advantages, the suitable ratio of the possible nanopores with different sizes is critical for the combination of efficient ion diffusion and storage. While for the specific nanopores (mainly micropores), the size compatibility between electrolyte ions and existing nanopores also needs to be considered carefully. Advanced carbon design should take both into account. Furthermore, available ions for adsorption are not only Zn2+, but also various anions in aqueous electrolyte. At this point, we summarized the representative electrolyte ions with original and hydration ion radius in Table to serve as a reference for carbon design.
3.2.3. Heteroatom-doped engineering
The conventional Helmholtz layer capacitance for carbon cathodes is inadequate, but a synergistic contribution of Faradic/non-Faradic charge storage is highly desired. Heteroatom-doped engineering can enrich the functionality of carbon surface to activate the Faradic behaviors for extra pseudocapacitance. Accordingly, heteroatoms such as O, N, B, P, S have been attempted to dope in carbon skeleton with single or multiple forms in the reports of all-carbon based cathodes. Thereby, this section is organized by multi-dimensional structured carbons with various elements doped to present the advanced heteroatom-doped engineering.
Since porous carbon generally undergoes low-temperature carbonization and activation, introduction of oxygen to carbon surface is inevitable. Common doped oxygen can activate the Faradic pseudocapacitive reaction and optimize the physicochemical properties of carbon. For instance, oxygen-enriched 1D porous flexible CNFs (denoted as OPCNFs) were synthesized via the electrospinning and acid-assisted oxidation technology [Citation70]. After oxidation treatment, the new peak appearing in FTIR spectra at 1728 cm−1 indicated that the carbonyl (C=O) and carboxyl (COOH) groups were introduced (Figure (a)). Oxidation time was optimized to balance the oxidation degree. The CNFs after 20 h treatment with a moderate oxygen content showed the best conductivity and hydrophilicity. The reversible composition change observed from ex-situ XPS characterization during discharge/charging process indicated that both C=O and COOH were involved in pseudocapacitive reaction (Figure (b)). The effect of the type of oxygen functional groups on CNT’s electrochemical behaviors was further elucidated by different functionalized CNTs (i.e. c-CNTs, p-CNTs, h-CNTs) [Citation177]. XPS characterization confirmed the existence of hydroxyl and carboxyl groups on the surface of h-CNT and c-CNT, respectively, but p-CNT contained almost no oxygen. It argued that the number of hydroxyl groups on the h-CNTs surface is larger than that of carboxyl groups on the c-CNTs since there two have similar oxygen content but hydroxyl monomer in h-CNT requires less oxygen atoms. The similar structure of the three CNTs highlights the contribution of oxygen groups to capacity. A significate amount of hydroxyl groups of h-CNT can not only activate chemical adsorption (C–O + Zn2+ + 2e- ↔ C–O···Zn) for extra pseudocapacitance, but also endow the h-CNTs with better hydrophilic feature. Thus, the h-CNTs cathode delivered higher ion storage ability than others. As for 2D carbon nanomaterials, oxygen functionalized CNSs (denoted as HPC-x) were prepared via the direct pyrolysis of potassium citrate [Citation175]. In this case, regulation of oxygen doping was achieved efficiently by controlling the pyrolysis temperature. The sample pyrolyzed at 600°C (denoted as HPC-600) showed the highest proportion of hydroxyl group (C-OH) among the HPC samples as depicted in Figure (e). Comprehensive characterizations showed that the chemisorption of Zn2+ mainly occurred at C–OH and COOH groups to form C–O–Zn and C–OO–Zn. Thereby, benefiting from the high content of C-OH groups to offer abundant interaction sites, HPC-600 delivered the best rate performances (Figure (f)). In the 3D carbon material, an oxygen-doped porous carbon was prepared by annealing the tetra-alkali metal pyromellitic acid salts [Citation35]. The highly reactive alkali metal ions (Li, Na, K, Rb, and Cs) can lead to the self-activation phenomenon. As shown in Figure (h), the optimized Rb-activated carbon (denoted as RbPC) exhibited well-developed porosity and abundant lattice defects. Moreover, XPS fitting results showed that RbPC possessed a high percentage of –C=O and –C–OH groups, which can effectively enhance the chemical adsorption and surface wettability (Figure (i)). Thus, the RbPC delivered the highest capacity compared with other samples (Figure (j)).
Figure 9. (a) Fourier transform infrared spectroscopy (FTIR) spectra of PCNF and OPCNF-x. (b) Oxygen functional group ratios of OPCNF sample based on ex-situ XPS fitting results during the discharge (state I-III) and charge process (state III-V). Reproduced with permission. Copyright 2021, Elsevier [Citation70]. (c) Chemical composition of N, O doped CNF samples. Reproduced with permission. Copyright 2022, Elsevier [Citation174]. (d) The configurations of Zn adsorption on the N-PCNTs and SN-PCNTs as well as corresponding adsorption energy and calculated charge density difference. Reproduced with permission. Copyright 2022, Royal Society of Chemistry [Citation65]. (e) The contents of oxygen bonding configurations in PC-600 and HPC-x. (f) Rate capability of HPC-x at different current density. Reproduced with permission. Copyright 2021, Royal Society of Chemistry [Citation175]. (g) Charge pattern of N–C–O–H and P–C–O–H in CNPK sample. Reproduced with permission. Copyright 2021, Royal Society of Chemistry [Citation77]. (h) TEM image of RbPC. (i) High-resolution O 1s XPS spectra of RbPC. (j) GCD curves at 1 A g−1 of RbPC and contrast samples. Reproduced with permission. Copyright 2022, American Chemical Society [Citation35]. (k) C 1s XPS spectra at full-discharge state and (l) relative energy profiles along the reaction pathway of PC and HNPC. Reproduced with permission. Copyright 2019, WILEY-VCH [Citation106]. Relative energy values of (m) B0S3C and (n) B2S0C in the reaction process compared with B0S0C. Reproduced with permission. Copyright 2022, Royal Society of Chemistry [Citation176].
![Figure 9. (a) Fourier transform infrared spectroscopy (FTIR) spectra of PCNF and OPCNF-x. (b) Oxygen functional group ratios of OPCNF sample based on ex-situ XPS fitting results during the discharge (state I-III) and charge process (state III-V). Reproduced with permission. Copyright 2021, Elsevier [Citation70]. (c) Chemical composition of N, O doped CNF samples. Reproduced with permission. Copyright 2022, Elsevier [Citation174]. (d) The configurations of Zn adsorption on the N-PCNTs and SN-PCNTs as well as corresponding adsorption energy and calculated charge density difference. Reproduced with permission. Copyright 2022, Royal Society of Chemistry [Citation65]. (e) The contents of oxygen bonding configurations in PC-600 and HPC-x. (f) Rate capability of HPC-x at different current density. Reproduced with permission. Copyright 2021, Royal Society of Chemistry [Citation175]. (g) Charge pattern of N–C–O–H and P–C–O–H in CNPK sample. Reproduced with permission. Copyright 2021, Royal Society of Chemistry [Citation77]. (h) TEM image of RbPC. (i) High-resolution O 1s XPS spectra of RbPC. (j) GCD curves at 1 A g−1 of RbPC and contrast samples. Reproduced with permission. Copyright 2022, American Chemical Society [Citation35]. (k) C 1s XPS spectra at full-discharge state and (l) relative energy profiles along the reaction pathway of PC and HNPC. Reproduced with permission. Copyright 2019, WILEY-VCH [Citation106]. Relative energy values of (m) B0S3C and (n) B2S0C in the reaction process compared with B0S0C. Reproduced with permission. Copyright 2022, Royal Society of Chemistry [Citation176].](/cms/asset/0be9f51a-3fd7-40aa-83fe-da0ba18d91e3/tmrl_a_2178860_f0009_oc.jpg)
The doped oxygen is usually not alone in carbon, but accompanied by other heteroatomic elements. The first need to mention should be nitrogen. The outstanding contributions in optimizing physicochemical properties and boosting chemisorption inspired a series of studies on N doping engineering. As a typical example, N, O riched-1D CNFs were obtained by KOH activating and following N doping [Citation174]. N doping process was operated at various pyrolysis temperature (700–1100°C) in which the maximum N doping concentration and electrical conductivity were achieved at 900°C (denoted as N-CNF900). Moreover, as shown in Figure (c), the N-CNF900 with highest content of pyrrolic N and graphitic N showed the best performances, thereby highlighting the contribution of these configurations to improve capacity. In addition, the 2D CNSs with N, O dual doping were prepared by carbonizing glycerol assisted by zinc acetate template and urea. The optimization of template dosage not only dominated the morphology, pore structure and SSA, but also affected the content of O and N [Citation76]. The optimized sample with medium doping levels but highest SSA and rich mesopores performed better than others. This also indicates that SSA and porosity need to be considered in heteroatoms-doped engineering. For 3D carbon nanomaterials, a hierarchical porous carbon with N doping (denoted as HNPC) was constructed for the purpose of enhancing the chemical adsorption of Zn2+ on oxygen groups [Citation106]. XPS fitting results showed that N doping increases the strength ratio of C–O–Zn and C–OH from 0.654 of contrast sample (PC) to 1.417 of HNPC at full-discharge state (Figure (k). Moreover, as revealed by theoretical simulations in Figure (l), N doping engineering can significantly decrease the energy barrier for forming C–O–Zn bonding (1.25 eV for PC vs. 2.13 eV for HNPC), thus effectively boosting the chemical adsorption of Zn2+ at the carbon surface.
Except for single N, Zhang et al. presented S incorporated N, O-rich porous carbon nanotubes (denoted as SN-PCNTs) [Citation65]. The sulfur incorporation can effectively modulate absorption kinetics and electron transfer behaviors to boost Zn2+ storage capability, which was well confirmed by higher Zn2+ diffusion coefficient of SN-PCNTs than that of S-free sample (N-PCNTs) (1.21 × 10−12 cm2 s−1 vs. 9.78 × 10−14 cm2 s−1). According to the density functional theory (DFT) simulations (Figure (d)), the adsorption energy of Zn2+ for SN-PCNTs was 0.33 eV, while only 0.17 eV for N-PCNTs. Furthermore, the O-doped 2D CNSs with incorporated N and P heteroatoms (denoted as CNPK) were proposed [Citation77]. As revealed by DFT calculation, the N and P doping effectively boosted the chemisorption of Zn2+ on O-groups through significantly decreasing the energy barrier (1.09, 0.78, and 2.06 eV for N-doped, P-doped, and non-doped sample, respectively). Additionally, the Bader charge analysis indicated that N introduction can endow O–H in N–C–O–H with stronger polarization, thereby being conducive to breaking O–H and forming C–O–Zn bond (Figure (g)). The P-doping can give O atoms in P–C–O–H more negative charges for easily attracting and reacting with Zn2+. Therefore, the CNPK performed better than non-doped samples (103.2 vs. 52.9 mAh g−1 at 0.1 A g−1, 52.8 vs. 21.4 mAh g−1 at 20 A g−1). Another 3D spongy-like hierarchically porous carbon with B, S co-doping was similarly analyzed by means of DFT calculation (Figure (m,n)) [Citation176]. The energy barrier for B-doped (B2S0C) and S-doped (B0S3C) samples were much lower than those for samples without doping (B0S0C) (2.290 and 1.429 eV vs. 3.203 eV).
Although the role of oxygen played in heteroatom-doped engineering for boosting pseudocapacitance is remarkable, the specific mechanism of dominant heteroatomic configuration (e.g. C–OH, C=O and –COOH) remains to be further studied. Moreover, maximizing the contribution of doping engineering requires that many heteroatoms work together. However, in the existing multiple-atom doping cases, the contributions of non-oxygen heteroatoms doping are only highlighted to boost the pseudocapacitive behaviors of O-groups. Therefore, further research is still needed on the effects of doping level, functional configuration of these non-oxygen doped atoms on adsorption behaviors and electrochemical performances.
3.3. Carbon in conversion-type cathode
Current conversion-type cathodes include traditional Mn-, V-based materials and emerging chalcogens. For traditional Mn- and V-based materials, the transformation of storage mechanism is unable to bypass the intrinsic drawbacks of poor conductivity, dynamic transport and severe volume expansion. Therefore, the functionality of nanocarbons in intercalation-type cathodes can be inherited as well. While the emerging chalcogens also suffer from these challenges, which inevitably include a carbon host. In this part, we largely inherited summary rules based on functionality of carbons in intercalation-type cathodes to discuss the progress of multi-dimension structured carbons, but only including limited number of reports according to our thorough research (Table ).
Table 4. A summary of multi-dimensional structured carbon nanomaterials for conversion-type cathodes.
3.3.1. Conductive substrate
Carbon nanomaterials as conductive substrates to anchor active species can not only contribute to facilitating kinetics of both ions and electrons, but also improving structural stability and even endowing composite with flexibility. Although some of the Mn and V-based materials were already verified to rely on the conversion-type mechanism, these materials have not been reported to be constructed as non-all-carbon based electrodes. Here we can only discuss the limited number of the existing cases. For example, active Co3O4 nanosheets array arranged on 1D carbon nanotube fibers (CNTFs) were constructed via oxidation treatment of Co-based MOF on CNTF (denoted as Co3O4@CNTFs) [Citation180]. The evenly distributed Co3O4 nanosheets with porous and ultrathin structure were conducive to the exposure of reactive sites and permeation of electrolyte. Line resistance comparison of CNTF and Co3O4@CNTF (23.00 Ω cm−1 vs. 53.53 Ω cm−1) showed that the Co3O4@CNTF inherited the excellent conductivity of pristine CNTF. Moreover, Figure (a,b) indicated that the flexible fiber-shaped Co3O4@CNTF electrode showed good deformation adaptability upon 0-150° bending with negligible resistance change. A pair of redox peaks was observed in cyclic voltammetry (CV) curves, which was attributed to the reversible conversion reactions (Co3O4 + 2H+ + 2e- ↔ 3CoO + H2O). Further analysis indicated the fitting slope b by plotting the relationship between log i (peak current) and log v (scan rate) was 0.82–0.88 (Figure (c)). And capacitance contribution was as high as 72.67% at high scan rate of 7 mV s−1 (Figure (d)). This efficient pseudocapacitive behavior is benefited from the fast ion/electron transport in self-supported 3D structure of the Co3O4@CNTF with high conductive CNTF framework.
Figure 10. (a) Photographs of the bending process of the Co3O4@CNTF cathode. (b) I-V measurements of Co3O4@CNTF at various bending angles. (c) b values determined by the relationship between the scan rate and peak current densities. (d) Normalized contribution ratio of the capacitive and diffusion-controlled capacities at different scan rates. Reproduced with permission. Copyright 2021, American Chemical Society [Citation180]. (e) Schematic of the synthetic procedure for the VN/NC hybrid nanosheets. The SEM images of (f) VN-control and (g) VN/NC after 500 cycles at 10 A g−1. Reproduced with permission. Copyright 2022, Royal Society of Chemistry [Citation78]. (h) TEM image of V2O3@C. (i) Schematic illustration of energy storage mechanism and (j) rate capability of V2O3@C in voltage ranges of 0.3–1.2 V and 0.3–1.6 V. Reproduced with permission. Copyright 2022, Royal Society of Chemistry [Citation181].
![Figure 10. (a) Photographs of the bending process of the Co3O4@CNTF cathode. (b) I-V measurements of Co3O4@CNTF at various bending angles. (c) b values determined by the relationship between the scan rate and peak current densities. (d) Normalized contribution ratio of the capacitive and diffusion-controlled capacities at different scan rates. Reproduced with permission. Copyright 2021, American Chemical Society [Citation180]. (e) Schematic of the synthetic procedure for the VN/NC hybrid nanosheets. The SEM images of (f) VN-control and (g) VN/NC after 500 cycles at 10 A g−1. Reproduced with permission. Copyright 2022, Royal Society of Chemistry [Citation78]. (h) TEM image of V2O3@C. (i) Schematic illustration of energy storage mechanism and (j) rate capability of V2O3@C in voltage ranges of 0.3–1.2 V and 0.3–1.6 V. Reproduced with permission. Copyright 2022, Royal Society of Chemistry [Citation181].](/cms/asset/8a2aad4f-6c25-4f8c-b337-a480a49fd45f/tmrl_a_2178860_f0010_oc.jpg)
Another typical conversion-type V-based cathode (VN) was supported by 2D CNSs [Citation78]. Specially, as shown in Figure (e), the hybrid nanosheets (denoted as VN/NC) with layer-by-layer stacked structure consist of VN nanocrystals and N doped CNSs, which were fabricated via in-situ thermal conversion of V2O5 with pyrolyzing pentyl viologen (PV) intercalated. During the pyrolysis, the V2O5 nanosheets were converted into VN nanocrystals, while the intercalated PV molecules were synchronously carbonized into N-doped CNSs. The blank sample of pure VN was fabricated via pyrolyzing V2O5 without PV, which showed sheet-like morphology and stacked into porous blocks. It was observed that the CNSs can inhibit the two-dimensional growth of VN crystals and serve as support to induce the formation of VN nanocrystals. The VN/NC with a stable structure consisting of ultrasmall VN and conductive substrate delivered more excellent rate capacity than pure VN. Moreover, the CNS can offer robust support for VN nanocrystals to restrain volume variation and self-aggregation, thus minimizing the damage to crystal structure during cycling. Therefore, the hybrid nanosheets with nanocrystals anchored were well maintained after long-cycling while VN blocks were broken into small pieces (see the SEM images in Figure (f,g)). Consequently, VN/NC sample showed better cyclability compared to pure VN.
In regarding the substrate function, reasonable arrangement of reactive species with optimized morphology on the substrate is crucial to improve utilization and structural stability. In the above two examples, Co3O4 was anchored on the substrate with an ultrathin 2D nanosheet, while VN was as nanocrystal. Both of these are helpful for exposing active sites and increasing utilization. In addition, further expand study for carbon substrate is also urgently needed because of the conversion-type cathodes are not carefree in rate/cycling performances.
3.3.2. Protecting layer
In the case of above-mentioned Co3O4@CNTFs, the additional CoSO4 solvent in electrolyte contributed to the excellent performance of Co3O4@CNTF by inhibiting dissolution behaviors [Citation180]. This suggests that dissolving concerns also come with some conversion-type species, therefore, using the protective layer to isolate the electrolyte should be considered. In a typical example, V2O3 with the coated carbon layer (denoted as V2O3@C) was fabricated via carbothermic reduction of polyaniline-coated V2O5·nH2O [Citation181]. TEM image showed that the thickness of this carbon shell was about 4.9 nm (Figure (h)). Moreover, the introduction of carbon layer (∼25.52 wt%) endowed the composite with a high SSA of 106.7 m2 g−1 and abundant mesopores, which can contribute to efficient ion transport. Interestingly, as depicted in Figure (i), a distinct phase transition from the original V2O3 to V2O5·3H2O occurred during the initial discharge in the extended high-voltage region (0.3–1.6 V). Moreover, the electrode exhibited a highly reversible conversion reaction between V2O5·3H2O and Zn3V2O7(OH)2·2H2O during the following charge/discharge process. The as-assembled system operated at 0.3–1.6 V also exhibited lower charge transfer resistance (186.8 Ω vs. 306.4 Ω) and larger Zn2+ diffusion coefficients (4.39 × 10−10–4.15 × 10−9 cm2 s−1 vs. 1.88 × 10−10–3.35 × 10−9 cm2 s−1). Thereby, the delivered capacity of 472.4 mAh g−1 was approximately five times higher than that of the original V2O3 at 0.3–1.2 V (Figure (j)). The protecting carbon layer can not only restrict dissolution issue, but also support and stabilize the electrochemically induced phase transitions.
Notwithstanding the aforementioned progress, the studies on carbon layer for conversion-type cathodes are few. Although the isolation of electrolyte by the carbon layer is beneficial to alleviate the dissolution and volume change, it also poses a challenge to the carbon separator between the electrolyte and the active species. The precise regulation on the thickness, internal pore structure and SSA of carbon layer is critical for ensuring the efficient transport of ions across the carbon layer as described in the carbon layer for intercalation-type cathodes.
3.3.3. Encapsulating host
In this section, we focus on the chalcogens-based cathodes. Due to the high theoretical capacity and multi-electron transfer processes, those cathodes are superior to other types of active species in terms of capacity and energy density. But it inevitably requires the introduction of a carbon to ease the concerns on electronic conductivity and volume expansion. Here the active species are generally encapsulated into nanopores of carbon by melting diffusion. This is not exactly the same function as conductive matrix, thereby the title of ‘host’ for carbon is more accurate.
In a report of 1D CNTs, the infiltration of active S was achieved via melting-diffusion strategy (160°C for 16 h) [Citation31]. The first concern is to examine the effect of electrolyte with different types of anions to electrochemical behaviors in which the focus was on multiple electrochemical performances. Based on the comprehensive comparison of reversible capacity, Coulombic efficiency and voltage hysteresis, the CNTs supported 50 wt% sulfur (denoted as S@CNT-50) preferred to work in the Zn(CH3COO)2 electrolyte with 0.05 wt% I2 as additive (pH of 6.6). Thereinto, the additive of 0.05 wt% I2 made S@CNT-50 show a smaller voltage hysteresis of 0.72 V and higher reversible capacity of 1105 mAh g−1 (Figure (a)). Such case can be attributed to that I2 served as medium of Zn2+ to reach lower reaction barrier. Then, another concern of S content was considered. Figure (b) showed the electrochemical performances of S@CNT samples with different content of active S. Despite the highest capacity and the smallest overpotential, S@CNTs-30 (S content of 30 wt%) still suffered severe parasitic reaction during the charge. While for S@CNTs-60, the lowest capacity and high overpotential caused by severe underutilization were unsatisfied. Thereinto, S@CNTs-50 with relatively high capacity and low overpotential was most appropriate based on comprehensive consideration. Moreover, it also showed the excellent cyclability with capacity retention of 85% over 50 cycles at 1000 mA g−1 (Figure (c)). It should be noted that S@CNTs-50 with 5 wt% Pt catalyst addition delivered a higher capacity of 1193 mAh g−1S and a smaller voltage hysteresis of 0.6 V (Figure (d)). This provides valuable information that introduction of catalysts into the host may improve the electrochemical behavior of S, as being well verified in some non-carbon hosts [Citation32,Citation46].
Figure 11. GCD curves of (a) S@CNTs-50 cathode at 100 mA g−1 and (b) S@CNTs with different sulfur contents at 200 mA g−1 in 1 M Zn(CH3COO)2 with 0.05 wt% I2 as additive. (c) Cycling performance of S@CNTs-50 cathode at a current density of 1000 mA g−1 (d) GCD curve of S@CNTs-50 with 5 wt% Pt at 100 mA g−1. Reproduced with permission. Copyright 2020, WILEY-VCH [Citation31]. (e) SEM image of SeS2@PCS. (f) N2 adsorption/desorption isotherms of PCS and SeS2@PCS. (g) TGA curve of SeS2@PCS. (h) GCD curves of SeS2@PCS in 1 M ZnSO4 with or without additive. Reproduced with permission. Copyright 2021, Elsevier [Citation128]. (i) TGA curves of pure CMK-3 and the Se/CMK-3 composite. (j) GCD curves under different current densities and (k) cycling performance under 1 A g−1 of Se/CMK-3 composite based on 2 M ZnTFSI/PEG/water aqueous electrolyte. Reproduced with permission. Copyright 2021, Royal Society of Chemistry [Citation33].
![Figure 11. GCD curves of (a) S@CNTs-50 cathode at 100 mA g−1 and (b) S@CNTs with different sulfur contents at 200 mA g−1 in 1 M Zn(CH3COO)2 with 0.05 wt% I2 as additive. (c) Cycling performance of S@CNTs-50 cathode at a current density of 1000 mA g−1 (d) GCD curve of S@CNTs-50 with 5 wt% Pt at 100 mA g−1. Reproduced with permission. Copyright 2020, WILEY-VCH [Citation31]. (e) SEM image of SeS2@PCS. (f) N2 adsorption/desorption isotherms of PCS and SeS2@PCS. (g) TGA curve of SeS2@PCS. (h) GCD curves of SeS2@PCS in 1 M ZnSO4 with or without additive. Reproduced with permission. Copyright 2021, Elsevier [Citation128]. (i) TGA curves of pure CMK-3 and the Se/CMK-3 composite. (j) GCD curves under different current densities and (k) cycling performance under 1 A g−1 of Se/CMK-3 composite based on 2 M ZnTFSI/PEG/water aqueous electrolyte. Reproduced with permission. Copyright 2021, Royal Society of Chemistry [Citation33].](/cms/asset/ca0766c5-7ebd-4d41-9edc-37ccd37823b2/tmrl_a_2178860_f0011_oc.jpg)
For 2D carbon nanomaterials, the concern on spatial restriction such as those in the carbon encapsulating matrix are totally freed because the active molecules are encapsulated into nanopores. For example, the P-doped porous carbon sheets (denoted as PCS) were prepared to encapsulate the active SeS2 (Figure (e)) [Citation128]. The PCS showed specific surface area of 1828.5 m2 g−1 and pore volume of 1.03 cm3 g−1. The flourishing porous structure can afford adequate accommodation for the encapsulation of SeS2 and the infiltration of electrolyte. As shown in Figure (f), the sharp decrease of SSA from 1828.5 to 381.9 m2 g−1 and pore volume from 1.03 to 0.19 m2 g−1 can be described as the penetration of nanopores by SeS2. Thermogravimetric analysis (TGA) indicated that the mass content of SeS2 was 61 wt% (Figure (g)). This report highlighted the I2 additive as redox mediator to boost the reversible capacity and kinetics (Figure (h)). While from the viewpoint of carbon, doping of non-metallic heteroatoms is also worthy of attention. It has been verified in the organic system that non-metallic doping can reduce the activation energy to accelerate conversion reaction. However, the catalytic potency of doping for redox reaction in aqueous systems remains to be theorized.
Nanopores are also worth attention to confine active molecules. For instance, the 3D mesopore-enriched CMK-3 carbon was employed as an encapsulating host for Se via melting-diffusion method [Citation33]. As demonstrated by TG in Figure (i), the mass content of Se in Se/CMK-3 composite was up to 50.1 wt%. A significant decay in SSA and the disappearance of mesopores in CMK-3 host can be found after Se infiltration. The homogeneous encapsulation of Se in the ordered channels of CMK-3 ensured efficient utilization of the active substance and restriction for volume expansion. The Zn-Se system was operated in a ‘molecule crowding’ aqueous electrolyte in which the additive of 5 wt % polyethylene glycol (PEG) ensured a stable potential up to 2.2 V due to the reduced chemical activity of water. Thereby, it delivered a high specific capacity of 611 mAh g−1Se (751 Wh kg−1) with the potential plateau of more than 1 V (Figure (j)) and retained 365 mAh g−1Se at 1 A g−1 after 1000 cycles with retention of 80.3% (Figure (k)).
These chalcogens-based cathodes are still in their infancy, consequently further research is urgently needed. Furthermore, the reported carbon hosts only include CNT and template-assisted carbon, and more porous carbons are worth considered as the encapsulating host. Moreover, the optimization of carbon host should not only focus on the mass ratio, and the pore structure and doped metallic/non-metallic heteroatoms with catalytic function deserve more attention for boosting the performances.
4. Conclusions and perspectives
To sum up, we provide a comprehensive review covering the typical cathode storage models and highlighting the advanced carbon architecture designs to boost the cathode performances. The progress of multi-dimensional structured carbon nanomaterials for constructing the non-all-carbon based intercalation- and conversion-type electrodes, and the all-carbon based adsorption-type electrodes have been systematically discussed. However, some essential electrochemical details still remain to be elucidated. The pioneer carbon theories deserve more consideration to guide future endeavors of carbon-based cathodes design. Meanwhile, advanced characterization techniques are expected to explore the underlying mechanism of the electrochemistry process. Particularly, considering further promotion of the carbon architecture in the cathodes, more explorations are expected in the following directions as shown in Figure .
Figure 12. Perspectives for future fundamental research on carbon nanomaterials for intercalation, adsorption and conversion type cathodes in AZDs.
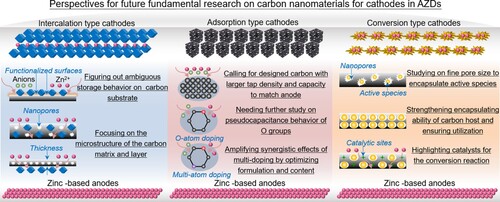
For the intercalation-type cathodes, nanocarbons function by serving as conductive substrates, encapsulating matrixes, and protecting layers to boost the performances. In these scenarios, the direct contact between the employed carbons and the electrolyte is unavoidable. Meanwhile, in order to pursue higher conductivity and more stable combination, especially for conductive substrates, enriching surface functionality via the introduction of heteroatomic groups is highly favored. Most intercalation-type species work at the voltage region of 0.3–1.8 V (vs. Zn2+/Zn), which overlaps with the physicochemical (de)adsorption region of available electrolyte ions. These favorable conditions make the functional nanocarbon potentially contribute to the surface-controlled capacitive behaviors. Simply assessing the capacity contribution of carbons to blur out these behaviors is inadequate. Study is needed to figure out these ambiguous electrochemical behaviors with the support of advanced in-situ or operando techniques. For the carbon matrix and layer, isolation effect amplifies the dominance of carbon shell for overall dynamic transport. Further careful control and deliberate design of carbon shell structure (e.g. micromorphology, thickness, internal porosity, and SSA) are needed for efficient ion penetration. Furthermore, balance should be established on the accessibility and robustness of the carbon shell to prevent excessive protection for improving utilization and to ensure certain mechanical strength for durability.
For the adsorption-type cathodes, the critical parameters that guide the selection of nanocarbons are as follows: high SSA, well-developed pore geometry, and sufficient pseudocapacitive active sites. Various hierarchical porous carbons, noted for large SSA, have sprung up. However, the current capacity level of carbon cathodes is seriously mismatched with that of zinc anode counterpart. The loading mass of active nanocarbons at laboratory level on cathode is generally 2 mg cm−2, corresponding to an areal specific capacity of approximately 0.5 mAh cm−2 (calculated with specific capacity of 250 mAh g−1). But the theoretical specific capacity of zinc anode with the thickness of 100 µm in laboratory usage is as high as 58.51 mAh cm−2. The energy density of the AZD device will plummet if being normalized by the total mass because anode is overused by more than 100 times. The extreme mass loads of active nanocarbons for electrochemical testing in the laboratory from some literatures can reach 30–40 mg cm−2, but the drastic capacity decay associated with underutilization is not acceptable. In terms of cathodes, future engineering on increasing mass loading, meanwhile maintaining the existing capacity level for active carbon is essential to driving practical applications. This calls for designed carbon with larger tap density and reasonable microstructure to ensure dynamic transport even at high mass loading. In addition, for the heteroatom-doping engineering, the high-profile but still controversial mechanisms for oxygen groups are worthy of further interpretation via in-situ techniques or simulation calculation. The synergistic effects of multi-atom doping also need to be continuously amplified by rational design of species collocation. The specific behaviors of the functional configuration for non-oxygen doped atoms in the electrochemical reaction are waiting to be theorized.
For the conversion-type cathodes, we’re going to focus on the chalcogens-based conversion cathodes in which carbon emerges as the encapsulating host. Introducing carbon host mainly relies on the typical melting-diffusion operation to encapsulate active chalcogens species into the nanopores. So, pore structure is worth paying attention to. It has been verified in the organic system that the internal pore size in carbon host interferes with the allotrope formation of resolidified chalcogens species. Therefore, detailed exploration to deliberate the role of nanopore size played on encapsulating active species and concurrent perturbations of electrochemical behavior would be of great interest. Moreover, the proportion of carbon host should also be taken seriously. At present, the inactive host accounts for 20–50% of the total mass, which substantially diminishes the overall energy density even though most of the reported values so far are normalized by only chalcogens mass. Strengthening the encapsulating capability of carbon host to afford reduced carbon usage is also urgently needed. Another point of concern is the sluggish kinetics of multiple-electron transfer. According to the sporadic inspired works in some non-carbon hosts, constructing the catalytic active sites in carbon host (e.g. metallic/non-metallic heteroatoms doping) to reduce the reaction energy barrier should be taken into further consideration.
Disclosure statement
No potential conflict of interest was reported by the author(s).
Additional information
Funding
References
- Zhu Y-h, Cui Y-f, Xie Z-l, et al. Decoupled aqueous batteries using pH-decoupling electrolytes. Nat Rev Chem. 2022;6(7):505–517.
- Ma L, Schroeder MA, Borodin O, et al. Realizing high zinc reversibility in rechargeable batteries. Nat Energy. 2020;5(10):743–749.
- Huang M, Wang X, Liu X, et al. Fast ionic storage in aqueous rechargeable batteries: from fundamentals to applications. Adv Mater. 2022;34(9):2105611.
- Deng Y-P, Liang R, Jiang G, et al. The current state of aqueous Zn-based rechargeable batteries. ACS Energy Lett. 2020;5(5):1665–1675.
- Xu L, Xu N, Yan C, et al. Storage mechanisms and improved strategies for manganese-based aqueous zinc-ion batteries. J Electroanal Chem. 2021;888:115196.
- Li C, Zhang X, He W, et al. Cathode materials for rechargeable zinc-ion batteries: from synthesis to mechanism and applications. J Power Sources. 2020;449:227596.
- Wu B, Luo W, Li M, et al. Achieving better aqueous rechargeable zinc ion batteries with heterostructure electrodes. Nano Res. 2021;14(9):3174–3187.
- Zhao X, Liang X, Li Y, et al. Challenges and design strategies for high performance aqueous zinc ion batteries. Energy Storage Mater. 2021;42:533–569.
- Wang X, Zhang Z, Xi B, et al. Advances and perspectives of cathode storage chemistry in aqueous zinc-Ion batteries. ACS Nano. 2021;15(6):9244–9272.
- Yang Q, Li X, Chen Z, et al. Cathode engineering for high energy density aqueous Zn batteries. Acc Mater Res. 2022;3(1):78–88.
- Niu Y, Gong S, Liu X, et al. Engineering iron-group bimetallic nanotubes as efficient bifunctional oxygen electrocatalysts for flexible Zn–air batteries. eScience. 2022;2(5):546–556.
- Liu Y, Umar A, Wu X. Metal-organic framework derived porous cathode materials for hybrid zinc ion capacitor. Rare Met. 2022;41(9):2985–2991.
- Hu Y, Fu C, Chai S, et al. Construction of zinc metal-Tin sulfide polarized interface for stable Zn metal batteries. Adv Powder Mater. 2023;2(2):100093.
- Wang M, Meng Y, Li K, et al. Toward dendrite-free and anti-corrosion Zn anodes by regulating a bismuth-based energizer. eScience. 2022;2(5):509–517.
- Huang S, Zhu J, Tian J, et al. Recent progress in the electrolytes of aqueous zinc-ion batteries. Chem Eur J. 2019;25(64):14480–14494.
- Jia X, Liu C, Neale ZG, et al. Active materials for aqueous zinc Ion batteries: synthesis, crystal structure, morphology, and electrochemistry. Chem Rev. 2020;120(15):7795–7866.
- Zhang T, Tang Y, Guo S, et al. Fundamentals and perspectives in developing zinc-ion battery electrolytes: a comprehensive review. Energy Environ Sci. 2020;13(12):4625–4665.
- Islam S, Alfaruqi MH, Mathew V, et al. Facile synthesis and the exploration of the zinc storage mechanism of β-MnO2nanorods with exposed (101) planes as a novel cathode material for high performance eco-friendly zinc-ion batteries. J Mater Chem A. 2017;5(44):23299–23309.
- Guo C, Liu H, Li J, et al. Ultrathin δ-MnO2 nanosheets as cathode for aqueous rechargeable zinc ion battery. Electrochim Acta. 2019;304:370–377.
- Wang J, Wang J-G, Liu H, et al. A highly flexible and lightweight MnO2/graphene membrane for superior zinc-ion batteries. Adv Funct Mater. 2021;31(7):2007397.
- Deng S, Tie Z, Yue F, et al. Rational design of ZnMn2O4quantum dots in a carbon framework for durable aqueous zinc-Ion batteries. Angew Chem Int Ed. 2022;61(12):e202115877.
- Long J, Yang Z, Yang F, et al. Electrospun core-shell Mn3O4/carbon fibers as high-performance cathode materials for aqueous zinc-ion batteries. Electrochim Acta. 2020;344:136155.
- Zhou W, Chen J, He C, et al. Hybridizing δ-type NaxV2O5·nH2O with graphene towards high-performance aqueous zinc-ion batteries. Electrochim Acta. 2019;321:134689.
- Sambandam B, Soundharrajan V, Kim S, et al. Aqueous rechargeable Zn-ion batteries: an imperishable and high-energy Zn2V2O7nanowire cathode through intercalation regulation. J Mater Chem A. 2018;6(9):3850–3856.
- Li Y, Huang Z, Kalambate PK, et al. V2o5 nanopaper as a cathode material with high capacity and long cycle life for rechargeable aqueous zinc-ion battery. Nano Energy. 2019;60:752–759.
- Ko JS, Paul PP, Wan G, et al. NASICON na3v2(PO4)3enables quasi-two-stage Na+and Zn2+intercalation for multivalent zinc batteries. Chem Mater. 2020;32(7):3028–3035.
- Niu Y, Wang D, Ma Y, et al. Cascading V2O3/N-doped carbon hybrid nanosheets as high-performance cathode materials for aqueous zinc-ion batteries. Chin Chem Lett. 2022;33(3):1430–1434.
- Trócoli R, La Mantia F. An aqueous zinc-ion battery based on copper hexacyanoferrate. ChemSusChem. 2015;8(3):481–485.
- Ma L, Chen S, Long C, et al. Achieving high-voltage and high-capacity aqueous rechargeable zinc Ion battery by incorporating Two-species redox reaction. Adv Energy Mater. 2019;9(45):1902446.
- Cui M, Fei J, Mo F, et al. Ultra-High-Capacity and dendrite-free zinc–sulfur conversion batteries based on a low-cost deep eutectic solvent. ACS Appl Mater Interfaces. 2021;13(46):54981–54989.
- Li W, Wang K, Jiang K. A low cost aqueous Zn–S battery realizing ultrahigh energy density. Adv Sci. 2020;7(23):2000761.
- Zhang H, Shang Z, Luo G, et al. Redox catalysis promoted activation of sulfur redox chemistry for energy-dense flexible solid-state Zn–S battery. ACS Nano. 2022;16(5):7344–7351.
- Chen Z, Mo F, Wang T, et al. Zinc/selenium conversion battery: a system highly compatible with both organic and aqueous electrolytes. Energy Environ Sci. 2021;14(4):2441–2450.
- Chen Z, Yang Q, Mo F, et al. Aqueous zinc–tellurium batteries with ultraflat discharge plateau and high volumetric capacity. Adv Mater. 2020;32(42):2001469.
- Wang L, Peng M, Chen J, et al. High energy and power zinc Ion capacitors: a dual-Ion adsorption and reversible chemical adsorption coupling mechanism. ACS Nano. 2022;16(2):2877–2888.
- Wu S, Chen Y, Jiao T, et al. An aqueous Zn-ion hybrid supercapacitor with high energy density and ultrastability up to 80 000 cycles. Adv Energy Mater. 2019;9(47):1902915.
- An G-H. Ultrafast long-life zinc-ion hybrid supercapacitors constructed from mesoporous structured activated carbon. Appl Surf Sci. 2020;530:147220.
- Lee B, Yoon CS, Lee HR, et al. Electrochemically-induced reversible transition from the tunneled to layered polymorphs of manganese dioxide. Sci Rep. 2014;4(1):6066.
- Wang H, Zhang S, Deng C. In situ encapsulating metal oxides into core–shell hierarchical hybrid fibers for flexible zinc-ion batteries toward high durability and ultrafast capability for wearable applications. ACS Appl Mater Interfaces. 2019;11(39):35796–35808.
- Yin Y-X, Xin S, Guo Y-G, et al. Lithium–sulfur batteries: electrochemistry, materials, and prospects. Angew Chem Int Ed. 2013;52(50):13186–13200.
- Li H, Ma L, Han C, et al. Advanced rechargeable zinc-based batteries: recent progress and future perspectives. Nano Energy. 2019;62:550–587.
- Yin J, Zhang W, Alhebshi NA, et al. Electrochemical zinc ion capacitors: fundamentals, materials, and systems. Adv Energy Mater. 2021;11(21):2100201.
- Wang H, Ye W, Yang Y, et al. Zn-ion hybrid supercapacitors: achievements, challenges and future perspectives. Nano Energy. 2021;85:105942.
- Tang H, Yao J, Zhu Y. Recent developments and future prospects for zinc-Ion hybrid capacitors: a review. Adv Energy Mater. 2021;11(14):2003994.
- Zhu C-L, Wang H-L, Fan W-J, et al. Large-scale doping-engineering enables boron/nitrogen dual-doped porous carbon for high-performance zinc ion capacitors. Rare Met. 2022;41(7):2505–2516.
- Ma L, Ying Y, Chen S, et al. Electrocatalytic selenium redox reaction for high-mass-loading zinc-selenium batteries with improved kinetics and selenium utilization. Adv Energy Mater. 2022;12(26):2201322.
- Alfaruqi MH, Islam S, Putro DY, et al. Structural transformation and electrochemical study of layered MnO2 in rechargeable aqueous zinc-ion battery. Electrochim Acta. 2018;276:1–11.
- Yan M, He P, Chen Y, et al. Water-lubricated intercalation in V2O5·nH2O for high-capacity and high-rate aqueous rechargeable zinc batteries. Adv Mater. 2018;30(1):1703725.
- Jiang B, Xu C, Wu C, et al. Manganese sesquioxide as cathode material for multivalent zinc Ion battery with high capacity and long cycle life. Electrochim Acta. 2017;229:422–428.
- Huang S, He S, Qin H, et al. Oxygen defect hydrated vanadium dioxide/graphene as a superior cathode for aqueous Zn batteries. ACS Appl Mater Interfaces. 2021;13(37):44379–44388.
- Lin Y, Zhou F, Chen M, et al. Building defect-rich oxide nanowires@graphene coaxial scrolls to boost high-rate capability, cycling durability and energy density for flexible Zn-ion batteries. Chem Eng J. 2020;396:125259.
- Wang D, Wang L, Liang G, et al. A superior δ-MnO2cathode and a self-healing Zn-δ-MnO2battery. ACS Nano. 2019;13(9):10643–10652.
- Li X, Li M, Yang Q, et al. Vertically aligned Sn4+ preintercalated Ti2CTX MXene sphere with enhanced Zn Ion transportation and superior cycle lifespan. Adv Energy Mater. 2020;10(35):2001394.
- Frackowiak E, Béguin F. Carbon materials for the electrochemical storage of energy in capacitors. Carbon N Y. 2001;39(6):937–950.
- Tian W, Zhang H, Duan X, et al. Porous carbons: structure-oriented design and versatile applications. Adv Funct Mater. 2020;30(17):1909265.
- Dou S, Tian Q, Liu T, et al. Stress-regulation design of mesoporous carbon spheres anodes with radial pore channels toward ultrastable potassium-Ion batteries. Small Sci. 2022;2(10):2200045.
- Xu W, Tang C, Huang N, et al. Adina rubella-like microsized SiO@N-doped carbon grafted with N-doped carbon nanotubes as anodes for high-performance lithium storage. Small Sci. 2022;2(4):2100105.
- Wang X, Wang H. Designing carbon anodes for advanced potassium-ion batteries: materials, modifications, and mechanisms. Adv Powder Mater. 2022;1(4):100057.
- Chen R, Tang H, He P, et al. Interface engineering of biomass-derived carbon used as ultrahigh-energy-density and practical mass-loading supercapacitor electrodes. Adv Funct Mater. 2022;33(8):2212078.
- Song T-B, Huang Z-H, Niu X-Q, et al. In-Situ growth of Mn3O4 nanoparticles on nitrogen-doped carbon dots-derived carbon skeleton as cathode materials for aqueous zinc Ion batteries. ChemSusChem. 2022;15(6):e202102390.
- Dong L, Ma X, Li Y, et al. Extremely safe, high-rate and ultralong-life zinc-ion hybrid supercapacitors. Energy Storage Mater. 2018;13:96–102.
- Hu H, Wu G. Porous carbon derived from sweet potato biomass as electrode for zinc-ion hybrid supercapacitors. Int J Electrochem Sci. 2021;16(9):210937.
- Sun G, Yang H, Zhang G, et al. A capacity recoverable zinc-ion micro-supercapacitor. Energy Environ Sci. 2018;11(12):3367–3374.
- Tian Y, Amal R, Wang D-W. An aqueous metal-ion capacitor with oxidized carbon nanotubes and metallic zinc electrodes. Front Energy Res. 2016;4:34.
- Li J, Yu L, Wang W, et al. Sulfur incorporation modulated absorption kinetics and electron transfer behavior for nitrogen rich porous carbon nanotubes endow superior aqueous zinc ion storage capability. J Mater Chem A. 2022;10(17):9355–9362.
- Wan F, Huang S, Cao H, et al. Freestanding potassium vanadate/carbon nanotube films for ultralong-life aqueous zinc-ion batteries. ACS Nano. 2020;14(6):6752–6760.
- Li W, Chen S, Yu J, et al. In-situ synthesis of interconnected SWCNT/OMC framework on silicon nanoparticles for high performance lithium-ion batteries. Green Energy Environ. 2016;1(1):91–99.
- Manjunatha R, Dong L, Zhai Z, et al. Pd nanocluster-decorated CoFe composite supported on nitrogen carbon nanotubes as a high-performance trifunctional electrocatalyst. Green Energy Environ. 2022;7(5):933–947.
- Sun J, Guo N, Song T, et al. Revealing the interfacial electron modulation effect of CoFe alloys with CoC encapsulated in N-doped CNTs for superior oxygen reduction. Adv Powder Mater. 2022;1(3):100023.
- He H, Lian J, Chen C, et al. Super hydrophilic carbon fiber film for freestanding and flexible cathodes of zinc-ion hybrid supercapacitors. Chem Eng J. 2021;421:129786.
- Chen X, Li W, Zeng Z, et al. Engineering stable Zn-MnO2 batteries by synergistic stabilization between the carbon nanofiber core and birnessite-MnO2 nanosheets shell. Chem Eng J. 2021;405:126969.
- Xu N, Yan C, He W, et al. Flexible electrode material of V2O5 carbon fiber cloth for enhanced zinc ion storage performance in flexible zinc-ion battery. J Power Sources. 2022;533:231358.
- Jin J, Sun Z, Yan T, et al. Demystifying activity origin of M–N–C single-atomic mediators toward expedited rate-determining step in Li–S electrochemistry. Small Sci. 2022;2(10):2200059.
- Wang X, Li Y, Wang S, et al. 2D amorphous V2O5/graphene heterostructures for high-safety aqueous Zn-Ion batteries with unprecedented capacity and ultrahigh rate capability. Adv Energy Mater. 2020;10(22):2000081.
- Luo J, Xu L, Liu H, et al. Harmonizing graphene laminate spacing and zinc-Ion solvated structure toward efficient compact capacitive charge storage. Adv Funct Mater. 2022;32(20):2112151.
- Wang D, Pan Z, Chen G, et al. Glycerol derived mesopore-enriched hierarchically carbon nanosheets as the cathode for ultrafast zinc ion hybrid supercapacitor applications. Electrochim Acta. 2021;379:138170.
- Zhang H, Chen Z, Zhang Y, et al. Boosting Zn-ion adsorption in cross-linked N/P co-incorporated porous carbon nanosheets for the zinc-ion hybrid capacitor. J Mater Chem A. 2021;9(30):16565–16574.
- Niu Y, Xu W, Ma Y, et al. Layer-by-layer stacked vanadium nitride nanocrystals/N-doped carbon hybrid nanosheets toward high-performance aqueous zinc-ion batteries. Nanoscale. 2022;14(20):7607–7612.
- Chen S, Ma L, Zhang K, et al. A flexible solid-state zinc ion hybrid supercapacitor based on co-polymer derived hollow carbon spheres. J Mater Chem A. 2019;7(13):7784–7790.
- Shang K, Liu Y, Cai P, et al. N, P, and S co-doped 3D porous carbon-architectured cathode for high-performance Zn-ion hybrid capacitors. J Mater Chem A. 2022;10(12):6489–6498.
- Wang H, Chen Q, Xiao P, et al. Unlocking zinc-ion energy storage performance of onion-like carbon by promoting heteroatom doping strategy. ACS Appl Mater Interfaces. 2022;14(7):9013–9023.
- Khatoon R, Attique S, Liu R, et al. Carbonized waste milk powders as cathodes for stable lithium–sulfur batteries with ultra-large capacity and high initial coulombic efficiency. Green Energy Environ. 2022;7(5):1071–1083.
- Xiong L, Qu Z, Shen Z, et al. In situ construction of ball-in-ball structured porous vanadium pentoxide intertwined with carbon fibers induces superior electronic/ionic transport dynamics for aqueous zinc-ion batteries. J Colloid Interface Sci. 2022;615:184–195.
- Wu J, Meng J, Yang Z, et al. Energy storage mechanism and electrochemical performance of Cu2O/rGO as advanced cathode for aqueous zinc ion batteries. J Alloy Compd. 2022;895:162653.
- Wang C, Zeng Y, Xiao X, et al. γ-MnO2 nanorods/graphene composite as efficient cathode for advanced rechargeable aqueous zinc-ion battery. J Mater Chem. 2020;43:182–187.
- Wang C, Wang M, He Z, et al. Rechargeable aqueous zinc–manganese dioxide/graphene batteries with high rate capability and large capacity. ACS Appl Energy Mater. 2020;3(2):1742–1748.
- Qin H, Yang Z, Chen L, et al. A high-rate aqueous rechargeable zinc ion battery based on the VS4@rGO nanocomposite. J Mater Chem A. 2018;6(46):23757–23765.
- Shao Y, Zeng J, Li J, et al. Sandwich structure of 3D porous carbon and water-pillared V2O5nanosheets for superior zinc-Ion storage properties. ChemElectroChem. 2021;8(10):1784–1791.
- Chen L, Yang Z, Cui F, et al. Ultrathin MnO2nanoflakes grown on N-doped hollow carbon spheres for high-performance aqueous zinc ion batteries. Mater Chem Front. 2020;4(1):213–221.
- Wang X, Ye L, Zou Y, et al. Constructing ultra-long life and super-rate rechargeable aqueous zinc-ion batteries by integrating Mn doped V6O13 nanoribbons with sulfur-nitrogen modified porous carbon. Mater Today Energy. 2021;19:100593.
- Xu C, Li B, Du H, et al. Energetic zinc Ion chemistry: the rechargeable zinc Ion battery. Angew Chem Int Ed. 2012;51(4):933–935.
- Wang J, Wang J-G, Liu H, et al. Electrochemical activation of commercial MnO microsized particles for high-performance aqueous zinc-ion batteries. J Power Sources. 2019;438:226951.
- Sun W, Wang F, Hou S, et al. Zn/MnO2battery chemistry with H+and Zn2+coinsertion. J Am Chem Soc. 2017;139(29):9775–9778.
- Qiu N, Chen H, Yang Z, et al. Low-cost birnessite as a promising cathode for high-performance aqueous rechargeable batteries. Electrochim Acta. 2018;272:154–160.
- Zhang N, Cheng F, Liu Y, et al. Cation-Deficient spinel ZnMn2O4cathode in Zn(CF3SO3)2electrolyte for rechargeable aqueous Zn-Ion battery. J Am Chem Soc. 2016;138(39):12894–12901.
- Li J, Luo N, Kang L, et al. Hydrogen-bond reinforced superstructural manganese oxide as the cathode for ultra-stable aqueous zinc ion batteries. Adv Energy Mater. 2022;12(44):2201840.
- Park J-S, Jo JH, Aniskevich Y, et al. Open-structured vanadium dioxide as an intercalation host for Zn ions: investigation by first-principles calculation and experiments. Chem Mater. 2018;30(19):6777–6787.
- Wan F, Zhang L, Dai X, et al. Aqueous rechargeable zinc/sodium vanadate batteries with enhanced performance from simultaneous insertion of dual carriers. Nat Commun. 2018;9(1):1656.
- Shan L, Yang Y, Zhang W, et al. Observation of combination displacement/intercalation reaction in aqueous zinc-ion battery. Energy Storage Mater. 2019;18:10–14.
- Huang M, Li F, Dong F, et al. MnO2-based nanostructures for high-performance supercapacitors. J Mater Chem A. 2015;3(43):21380–21423.
- Wu L, Dong Y. Recent progress of carbon nanomaterials for high-performance cathodes and anodes in aqueous zinc ion batteries. Energy Storage Mater. 2021;41:715–737.
- Alfaruqi M, Mathew V, Gim J, et al. Electrochemically induced structural transformation in a γ-MnO2cathode of a high capacity zinc-ion battery system. Chem Mater. 2015;27(10):3609–3620.
- Pan H, Shao Y, Yan P, et al. Reversible aqueous zinc/manganese oxide energy storage from conversion reactions. Nat Energy. 2016;1(5):16039.
- Boyd S, Augustyn V. Transition metal oxides for aqueous sodium-ion electrochemical energy storage. Inorg Chem Front. 2018;5(5):999–1015.
- Shao Y, Shen F, Shao Y. Recent advances in aqueous zinc-ion hybrid capacitors: a minireview. ChemElectroChem. 2021;8(3):484–491.
- Zhang H, Liu Q, Fang Y, et al. Boosting Zn-Ion energy storage capability of hierarchically porous carbon by promoting chemical adsorption. Adv Mater. 2019;31(44):1904948.
- Fan W, Ding J, Ding J, et al. Identifying heteroatomic and defective sites in carbon with dual-ion adsorption capability for high energy and power zinc ion capacitor. Nano-Micro Lett. 2021;13(1):59.
- Lu Y, Li Z, Bai Z, et al. High energy-power Zn-ion hybrid supercapacitors enabled by layered B/N co-doped carbon cathode. Nano Energy. 2019;66:104132.
- Zheng Y, Zhao W, Jia D, et al. Porous carbon prepared via combustion and acid treatment as flexible zinc-ion capacitor electrode material. Chem Eng J. 2020;387:124161.
- Yu P, Zeng Y, Zeng Y, et al. Achieving high-energy-density and ultra-stable zinc-ion hybrid supercapacitors by engineering hierarchical porous carbon architecture. Electrochim Acta. 2019;327:134999.
- Zhu C, Fang G, Zhou J, et al. Binder-free stainless steel@Mn3O4nanoflower composite: a high-activity aqueous zinc-ion battery cathode with high-capacity and long-cycle-life. J Mater Chem A. 2018;6(20):9677–9683.
- Wan F, Zhang Y, Zhang L, et al. Reversible oxygen redox chemistry in aqueous zinc-ion batteries. Angew Chem Int Ed. 2019;58(21):7062–7067.
- Fang G, Liang S, Chen Z, et al. Simultaneous cationic and anionic redox reactions mechanism enabling high-rate long-life aqueous zinc-Ion battery. Adv Funct Mater. 2019;29(44):1905267.
- Luo L-W, Zhang C, Wu X, et al. A Zn–S aqueous primary battery with high energy and flat discharge plateau. Chem Commun. 2021;57(77):9918–9921.
- Li W, Ma Y, Li P, et al. Synergistic effect between S and Se enhancing the electrochemical behavior of SexSy in aqueous Zn metal batteries. Adv Funct Mater. 2021;31(20):2101237.
- Zhang N, Cheng F, Liu J, et al. Rechargeable aqueous zinc-manganese dioxide batteries with high energy and power densities. Nat Commun. 2017;8(1):405.
- Soundharrajan V, Sambandam B, Kim S, et al. Aqueous magnesium zinc hybrid battery: an advanced high-voltage and high-energy MgMn2O4Cathode. ACS Energy Lett. 2018;3(8):1998–2004.
- Zhang N, Dong Y, Jia M, et al. Rechargeable aqueous Zn–V2O5battery with high energy density and long cycle life. ACS Energy Lett. 2018;3(6):1366–1372.
- Zhang N, Jia M, Dong Y, et al. Hydrated layered vanadium oxide as a highly reversible cathode for rechargeable aqueous zinc batteries. Adv Funct Mater. 2019;29(10):1807331.
- Xia C, Guo J, Li P, et al. Highly stable aqueous zinc-ion storage using a layered calcium vanadium oxide bronze cathode. Angew Chem Int Ed. 2018;57(15):3943–3948.
- Kundu D, Adams BD, Duffort V, et al. A high-capacity and long-life aqueous rechargeable zinc battery using a metal oxide intercalation cathode. Nat Energy. 2016;1(10):16119.
- Yang Q, Mo F, Liu Z, et al. Activating C-coordinated iron of iron hexacyanoferrate for Zn hybrid-Ion batteries with 10 000-cycle lifespan and superior rate capability. Adv Mater. 2019;31(32):1901521.
- Lee YG, An G-H. Synergistic effects of phosphorus and boron Co-incorporated activated carbon for ultrafast zinc-ion hybrid supercapacitors. ACS Appl Mater Interfaces. 2020;12(37):41342–41349.
- Wu D, Ji C, Mi H, et al. A safe and robust dual-network hydrogel electrolyte coupled with multi-heteroatom doped carbon nanosheets for flexible quasi-solid-state zinc ion hybrid supercapacitors. Nanoscale. 2021;13(37):15869–15881.
- Liu P, Liu W, Huang Y, et al. Mesoporous hollow carbon spheres boosted, integrated high performance aqueous Zn-Ion energy storage. Energy Storage Mater. 2020;25:858–865.
- Zhu X, Guo F, Yang Q, et al. Boosting zinc-ion storage capability by engineering hierarchically porous nitrogen-doped carbon nanocage framework. J Power Sources. 2021;506:230224.
- Li Z, Chen D, An Y, et al. Flexible and anti-freezing quasi-solid-state zinc ion hybrid supercapacitors based on pencil shavings derived porous carbon. Energy Storage Mater. 2020;28:307–314.
- Li W, Jing X, Ma Y, et al. Phosphorus-doped carbon sheets decorated with SeS2 as a cathode for aqueous Zn-SeS2 battery. Chem Eng J. 2021;420:129920.
- Bi S, Wu Y, Cao A, et al. Free-standing three-dimensional carbon nanotubes/amorphous MnO2 cathodes for aqueous zinc-ion batteries with superior rate performance. Mater Today Energy. 2020;18:100548.
- Yin B, Zhang S, Ke K, et al. Binder-free V2O5/CNT paper electrode for high rate performance zinc ion battery. Nanoscale. 2019;11(42):19723–19728.
- Yao Z, Cai D, Cui Z, et al. Strongly coupled zinc manganate nanodots and graphene composite as an advanced cathode material for aqueous zinc ion batteries. Ceram Int. 2020;46(8):11237–11245.
- Chen L, Yang Z, Qin H, et al. Advanced electrochemical performance of ZnMn2O4/N-doped graphene hybrid as cathode material for zinc ion battery. J Power Sources. 2019;425:162–169.
- Duan W, Zhao M, Li Y, et al. Excellent rate capability and cycling stability of novel H2V3O8 doped with graphene materials used in New aqueous zinc-ion batteries. Energy Fuels. 2020;34(3):3877–3886.
- Pang Q, Sun C, Yu Y, et al. H2v3o8nanowire/graphene electrodes for aqueous rechargeable zinc Ion batteries with high rate capability and large capacity. Adv Energy Mater. 2018;8(19):1800144.
- Luo H, Wang B, Wu F, et al. Synergistic nanostructure and heterointerface design propelled ultra-efficient in-situ self-transformation of zinc-ion battery cathodes with favorable kinetics. Nano Energy. 2021;81:105601.
- Luo H, Wang B, Wang C, et al. Synergistic deficiency and heterojunction engineering boosted VO2 redox kinetics for aqueous zinc-ion batteries with superior comprehensive performance. Energy Storage Mater. 2020;33:390–398.
- Yang C, Han M, Yan H, et al. In-situ probing phase evolution and electrochemical mechanism of ZnMn2O4 nanoparticles anchored on porous carbon polyhedrons in high-performance aqueous Zn-ion batteries. J Power Sources. 2020;452:227826.
- Xu D, Li B, Wei C, et al. Preparation and characterization of MnO2/acid-treated CNT nanocomposites for energy storage with zinc ions. Electrochim Acta. 2014;133:254–261.
- Dai X, Wan F, Zhang L, et al. Freestanding graphene/VO2 composite films for highly stable aqueous Zn-ion batteries with superior rate performance. Energy Storage Mater. 2019;17:143–150.
- Zhang Y, Deng S, Pan G, et al. Introducing oxygen defects into phosphate ions intercalated manganese dioxide/vertical multilayer graphene arrays to boost flexible zinc Ion storage. Small Methods. 2020;4(6):1900828.
- Zhang Y, Deng S, Li Y, et al. Anchoring MnO2 on nitrogen-doped porous carbon nanosheets as flexible arrays cathodes for advanced rechargeable Zn–MnO2 batteries. Energy Storage Mater. 2020;29:52–59.
- Zhang H, Yao Z, Lan D, et al. N-doped carbon/V2O3 microfibers as high-rate and ultralong-life cathode for rechargeable aqueous zinc-ion batteries. J Alloy Compd. 2021;861:158560.
- Wang S, Zhang S, Chen X, et al. Double–shell zinc manganate hollow microspheres embedded in carbon networks as cathode materials for high–performance aqueous zinc–ion batteries. J Colloid Interface Sci. 2020;580:528–539.
- Deng S, Yuan Z, Tie Z, et al. Electrochemically induced metal–organic-framework-derived amorphous V2O5for superior rate aqueous zinc-Ion batteries. Angew Chem Int Ed. 2020;59(49):22002–22006.
- Wu B, Zhang G, Yan M, et al. Graphene scroll-coated α-MnO2nanowires as high-performance cathode materials for aqueous Zn-Ion battery. Small. 2018;14(13):1703850.
- Zhang W, Liang S, Fang G, et al. Ultra-High mass-loading cathode for aqueous zinc-ion battery based on graphene-wrapped aluminum vanadate nanobelts. Nano-Micro Lett. 2019;11(1):69.
- Liu Y, Li Q, Ma K, et al. Graphene oxide wrapped CuV2O6nanobelts as high-capacity and long-life cathode materials of aqueous zinc-ion batteries. ACS Nano. 2019;13(10):12081–12089.
- Islam S, Alfaruqi MH, Song J, et al. Carbon-coated manganese dioxide nanoparticles and their enhanced electrochemical properties for zinc-ion battery applications. J Mater Chem. 2017;26(4):815–819.
- Bin D, Wang Y, Tamirat AG, et al. Stable high-voltage aqueous zinc battery based on carbon-coated NaVPO4F cathode. ACS Sustainable Chem Eng. 2021;9(8):3223–3231.
- Zhai X-Z, Qu J, Wang J, et al. Diffusion-driven fabrication of yolk-shell structured K-birnessite@mesoporous carbon nanospheres with rich oxygen vacancies for high-energy and high-power zinc-ion batteries. Energy Storage Mater. 2021;42:753–763.
- Wang C, Wang M, Liu L, et al. 3D porous sponge-inspired electrode for high-energy and high-power zinc-ion batteries. ACS Appl Energy Mater. 2021;4(2):1833–1839.
- Wu W, Wang S, Zhang C, et al. Facile and scalable synthesis of 3D structures of V10O24·12H2O nanosheets coated with carbon toward ultrafast and ultrastable zinc storage. ACS Appl Mater Interfaces. 2021;13(16):18704–18712.
- Xu H, Du Y, Emin A, et al. Interconnected vertical δ-MnO2nanoflakes coated by a dopamine-derived carbon thin shell as a high-performance self-supporting cathode for aqueous zinc Ion batteries. J Electrochem Soc. 2021;168(3):0030540.
- Chen L, Yang Z, Qin H, et al. Graphene-wrapped hollow ZnMn2O4 microspheres for high-performance cathode materials of aqueous zinc ion batteries. Electrochim Acta. 2019;317:155–163.
- Zhang X, Pei Z, Wang C, et al. Flexible zinc-ion hybrid fiber capacitors with ultrahigh energy density and long cycling life for wearable electronics. Small. 2019;15(47):1903817.
- Zhuang J, Wei F, Zou H, et al. Oxygen-rich lotus-shaped porous carbon cathode for high areal capacity zinc ion hybrid capacitors. Mater Lett. 2022;313:131737.
- Yu P, Zeng Y, Cao Q, et al. Liquid–liquid micromixing strategy enables low KOH-amount synthesis of ultrahighly porous carbon for zinc-ion storage. SN Appl Sci. 2020;2(5):829.
- Wei F, Zhang H, Wang J, et al. N, N, S co-doped porous carbons with well-developed pores for supercapacitor and zinc ion hybrid capacitor. J Alloy Compd. 2022;907:164536.
- Han L, Huang H, Li J, et al. Novel zinc–iodine hybrid supercapacitors with a redox iodide ion electrolyte and B, N dual-doped carbon electrode exhibit boosted energy density. J Mater Chem A. 2019;7(42):24400–24407.
- Li Y, Yang W, Yang W, et al. Towards high-energy and anti-self-discharge Zn-Ion hybrid supercapacitors with New understanding of the electrochemistry. Nano-Micro Lett. 2021;13(1):95.
- Li X, Li Y, Zhao X, et al. Elucidating the charge storage mechanism of high-performance vertical graphene cathodes for zinc-ion hybrid supercapacitors. Energy Storage Mater. 2022;53:505–513.
- Pan Z, Lu Z, Xu L, et al. A robust 2D porous carbon nanoflake cathode for high energy-power density Zn-ion hybrid supercapacitor applications. Appl Surf Sci. 2020;510:145384.
- Wang D, Pan Z, Lu Z. From starch to porous carbon nanosheets: promising cathodes for high-performance aqueous Zn-ion hybrid supercapacitors. Microporous Mesoporous Mater. 2020;306:110445.
- Lou G, Pei G, Wu Y, et al. Combustion conversion of wood to N, O co-doped 2D carbon nanosheets for zinc-ion hybrid supercapacitors. Chem Eng J. 2021;413:127502.
- Shang P, Liu M, Mei Y, et al. Urea-mediated monoliths made of nitrogen-enriched mesoporous carbon nanosheets for high-performance aqueous zinc Ion hybrid capacitors. Small. 2022;18(16):2108057.
- Largeot C, Portet C, Chmiola J, et al. Relation between the Ion size and pore size for an electric double-layer capacitor. J Am Chem Soc. 2008;130(9):2730–2731.
- Nightingale ER. Phenomenological theory of Ion solvation. effective radii of hydrated ions. J Phys Chem. 1959;63(9):1381–1387.
- Persson I. Hydrated metal ions in aqueous solution: how regular are their structures? Pure Appl Chem. 2010;82(10):1901–1917.
- Khuyen NQ, Zondaka Z, Harjo M, et al. Comparative analysis of fluorinated anions for polypyrrole linear actuator electrolytes. Polymers (Basel). 2019;11(5):849.
- Zeng J, Dong L, Sun L, et al. Printable zinc-ion hybrid micro-capacitors for flexible self-powered integrated units. Nano-Micro Lett. 2021;13(1):19.
- Marcus Y. Ionic radii in aqueous solutions. Chem Rev. 1988;88(8):1475–1498.
- Liu P, Chen Y, Xiang H, et al. Benefitting from synergistic effect of anion and cation in antimony acetate for stable CH3NH3PbI3-based perovskite solar cell with efficiency beyond 21%. Small. 2021;17(46):2102186.
- Asakawa T, Kubode H, Ozawa T, et al. Micellar counterion binding probed by fluorescence quenching of 6-methoxy-N-(3-sulfopropyl)quinolinium. J Oleo Sci. 2005;54(10):545–552.
- Amiri A, Naraghi M, Polycarpou AA. Zinc-ion hybrid supercapacitors with ultrahigh areal and gravimetric energy densities and long cycling life. J Mater Chem. 2022;70:480–491.
- Wang L, Huang M, Huang J, et al. Coupling of EDLC and the reversible redox reaction: oxygen functionalized porous carbon nanosheets for zinc-ion hybrid supercapacitors. J Mater Chem A. 2021;9(27):15404–15414.
- Zhang X, Zhang Y, Qian J, et al. Synergistic effects of B/S co-doped spongy-like hierarchically porous carbon for a high performance zinc-ion hybrid capacitor. Nanoscale. 2022;14(5):2004–2012.
- Li X, Li Y, Xie S, et al. Zinc-based energy storage with functionalized carbon nanotube/polyaniline nanocomposite cathodes. Chem Eng J. 2022;427:131799.
- Chen S, Yang G, Zhao X, et al. Hollow mesoporous carbon spheres for high performance symmetrical and aqueous zinc-ion hybrid supercapacitor. Front Chem. 2020;8:663.
- Zhao Y, Hao H, Song T, et al. High energy-power density Zn-ion hybrid supercapacitors with N/P co-doped graphene cathode. J Power Sources. 2022;521:230941.
- Lu Y, Zhang H, Liu H, et al. Electrolyte dynamics engineering for flexible fiber-shaped aqueous zinc-ion battery with ultralong stability. Nano Lett. 2021;21(22):9651–9660.
- Ma Y, Qi Y, Niu Y, et al. A distinctive conversion mechanism for reversible zinc ion storage. Inorg Chem Front. 2022;9(11):2706–2713.