Abstract
After cerebral infarction, the regeneration of microvascular played an important role in the recovery. Ginsenoside Rg1 (Rg1) had good effects on promoting angiogenesis and neuro-protection in cerebral infarction treatment. However, the blood-brain barrier (BBB) restricted Rg1 to enter into cerebral tissue. Transferrin receptor (TfR) was over-expressed in the BBB. In this study, we fabricated a TfR targeting nano-carrier (PATRC) to penetrate the BBB for treatment of cerebral infarction. A TfR targeted peptide was conjugated with the nano-carrier wrapped hydrophobic Rg1. The nanoscale size (132 ± 12 nm), polydispersity index (PDI =0.29) and the zeta potential (−38mv) were tested with dynamic light scattering optical system. Surface morphology (ellipse, mean diameter 122 ± 26 nm) was detected by transmission electron microscope (TEM). PATRC implement cell targeting ability on rat brain microvascular endothelial cells RBE4 in vitro detected by immunofluorescence and flow cytometry methods. Comparing with Rg1 threated group, the PATRC exhibited more prominent ability on the tube formation ability (p < .05) in vitro. Comparing with the Rg1 treated group, PATRC penetrated BBB in vivo detected by HPLC, decreased the brain infarction volume tested with TTC staining and promoted regeneration of microvascular in infarction zone detected by CD31 immunofluorescence. PATRC has great potentiality for wide application in clinic.
Introduction
Cerebral infarction was caused by brain blood supply disorders. Hypoxia and ischemia of brain tissue brought about the necrosis of brain tissue. Cerebral infarction was with high mortality, high disability rate, and high recurrence rate. Treatments for cerebral infarction have been mainly restricted to thrombo-lytic and neuro-protective therapies. However, the injection of thrombo-lytic drug for treatment of acute cerebral infarction was recommended for selected patients only within 4.5 h after cerebral infarction [Citation1]. The intracranial haemorrhage was with potentially fatal complication after intravenous thrombolysis treatment. The poor regeneration capacity of the cerebral tissue was regarded as the irreversible and progressive nature of cerebral infarction. Therefore, neuro-protection was a vital target shared by many clinical neurologists. Neuron-protective agents were other specialized treatments for cerebral infarction. Neuron-protection means to salvage brain tissue or delay the process of cerebral infarction that was to say; reducing the neuronal apoptosis or promoting nerve regeneration in cerebral infarction tissue under anoxia condition. After acute cerebral infarction, microvessels were under hypoxia condition, the microvessels apoptosis or necrosis occurred. To revascularize blood vessels and to restore the blood flow in ischemic brain tissue could improve the hypoxic state of ischemic tissue. To reduce the cerebral vascular deterioration and to enhance the formation of microvessels could implement neuron-protective and neuron-genesis function. Ginsenoside Rg1 (Rg1) is one of major ingredients in Panax notoginseng saponins [Citation2]. Great deal clinic reports and researches have suggested that Rg1 was with treatment function for some central nervous system (CNS) illnesses including anti-fatigue, anti-stress, anti-oxidation, anti-oedema, and the reduction of cerebral infarct volume, etc. [Citation3,Citation4]. Rg1 reduced the damage of free radicals to the nerve cells, promoted the reconstruction of nerve function, enhanced angiogenesis function, increased the blood flow of the damaged brain tissue, activated the neurotropic factor, and promoted the recovery of nerve function [Citation5,Citation6]. However, lots of pharmacokinetic researches on Rg1 indicated the poor cerebral enrichment of Rg1 [Citation7,Citation8]. One of the important causes was that the Rg1 could not effectually penetrate the blood-brain barrier (BBB) and implement accumulation in cerebral tissues. BBB allowed only some molecules to penetrate which limited the use of Rg1 to promote neuro-protection function in cerebral ischemia area [Citation4,Citation9].
With aid of drug delivery systems penetrating BBB would possess important clinical application for cerebral ischemia. The high expression of transferrin receptor (TfR) in cerebral cortex microvessels could aid the nano-medicine to cross the BBB by endocytosis [Citation10–12]. A brain-targeted drug delivery system targeting TfR, modified by polyamides dendrimer PAMAM, was developed for the treatment of gliomas using a specific antibody (OX26) modified brain targeting drug delivery system [Citation13,Citation14]. OX26 antibody was also fixed on the surface of polyglycerol-PLGA nanoparticles for the treatment of Alzheimer's disease and glioblastoma. Previously, we have reported an OX26 fixed Rg1 nanoparticle penetrating the BBB to improve the cerebral function of diabetic rats complicated with cerebral infarction [Citation15]. However, OX26 monoclonal antibodies were relatively larger in molecular weight which restricted its deeper tissue penetration, and the nonhuman FC fragments in many antibodies were with certain immunogenicity [Citation16,Citation17]. Peptides screened from the phage display methods showed a good affinity to the target and were smaller in size [Citation12]. The peptides conjugated with drug carrier could realize deeper tissue penetration and almost without immunogenicity. The use of peptides screened from phage display technology binding to TfR to penetrate BBB have been carried out in many researches [Citation18]. TfR targeted peptides were applied to couple liposome packaging digoxin to penetrate BBB [Citation19,Citation20], which exhibited great therapeutic effects for cancer with the aid of TfR mediated endocytosis. However, TfR targeted peptide conjugated drug carrier for the treatment of cerebral infarction has not been reported yet. The ideal drug carrier, γ-Poly glutamic acid (PGA), was produced from biological fermentation which was safe, nontoxic, degradable, and none immunogenicity. PGA based nano-drug carrier implement high drug wrapping rate, lower side effects [Citation21–23]. In this research, we used maleimide-fixed chitosan -γ-PGA complex to load bulk of Rg1 by self-assembly strategy for cerebral infarction treatment. Therefore, this work may present an efficient strategy for cerebral infarction treatment.
Materials and methods
Materials
An 8.0-μm chamber for trans-well assay was from Corning (NY, USA); γ-Poly glutamic acid was bought from Bolian company (Xian, China); the cell culture medium for endothelial cells (ECM) and rat cerebrovascular endothelial cell RBE4 were from Scinencell (California, USA); matrigel, penicillin/streptomycin(P/S), TTC solution, hematoxylin, eosin, DAPI and CD31 antibody were purchased from Sigma (Saint Louis, Missouri, USA); chitosan (MW 60 kDa, 85% deacetylation) was bought from Koyo Chemical company (Japan); N-Aminomaleimide was from GL Biochem company (Shanghai, China). TfR targeted peptide (TP:sequence CHAIYPRH) fixed with fluorescein isothiocyanate (FITC) and irrelevant control peptide (iTP:sequence CPIAHRHY) fixed with FITC were synthesized from Sino peptide company (Shanghai, China).
Fabrication of Rg1-loaded nanoparticles PATRC
Maleimide was universal to conjugate with kinds of ligands with thiols by a thia-Michael addition reaction to form thioether bond [Citation24,Citation25]. In this research, N-Aminomaleimide was used to conjugate with TfR targeted peptide with sulfhydryl groups on cysteine in peptide. N-Aminomaleimide(NAM, 80 mg) was conjugated to 200 mg (30 kDa) γ-PGA in 4 ml ultra-pure water by amide reactivity with EDCI for 24 h at room temperature to form the complex PA. 10 mg TfR targeted peptide with cysteine was added to conjugate with maleimide to form PAT complex. The reactivity was maintained at 4 °C in dark and last for 10 h. EDCI, unconjugated NAM and unconjugated TfR targeted peptide were removed by dialysis in ultra-pure water. PAT complex was harvested by vacuum freezing dryer under temperature of −40 °C (Yibokang, Beijing, China). PAT (20 mg) and Rg1 (5 mg) was dissolved in 2 ml DMSO to form the mixer of PATR. The PATR mixer solution was dropped into aqueous chitosan (1 mg/ml, PH6.0, 20 ml) within a magnetic mixer (120 rpm) at room temperature. Free Rg1 was wiped off through centrifugation (12,000 rpm, 10 min) and the dry nano-particles PATRC were harvested by a vacuum freezing dryer under the temperature of −40 °C.
Characterization of PATRC
PATRC was dyed with the solution of uranyl acetate, and then the shape and surface morphology were detected by a transmission electron microscopy (TEM, Hitachi, JP). The nano-size, zeta potential, and polydisperisty index (PDI) of PATRC were tested by a Malvern (UK) light scattering particle size analyzer.
Embedding efficacy
PATRC nanoparticles were harvested through centrifugation at 15,000 rpm at 4 °C for 10 min. The Rg1 loaded efficiency was analyzed by an Agilent high-performance liquid chromatography (HPLC, 1200, CA, USA) equipped with a C18 reversed-phase column (ODS1 4.6 mm ×150 mm). Supernatant sample of 10 μl was injected. A solution of methanol and 0.02 M sodium acetate (20: 80, V/V, pH 6.0) was used as mobile phase, and the current speed was maintained with 1 ml/min. The detector wavelength was kept 203 nm under 25 °C. The embedding efficiency (EE) of Rg1 in PATRC was calculated by the equitation EE = M1/M2, M1 stand for Rg1 in PATRC, and M2 means the total Rg1 applied in the equitation.
Apoptosis induced by hypoxia on RBE4 cells
Hypoxia induction of RBE4 cells were carried out through the incubation with Na2S2O4 that was able to continuously reduce oxygen pressure of solutions [Citation26,Citation27]. RBE4 (2 × 105) were cultured in 6 well plates in ECM culture medium added with 10% FBS and 1% P/S overnight. RBE4 cells were divided into PBS treatment group (added with10 μl PBS, control group), hypoxia induction group (added with 1 mmol/L Na2S2O4, HI group), hypoxia induction and Rg1 treatment group (added with 1 mmol/L Na2S2O4 and 10 μM Rg1, HI + Rg1 group), hypoxia induction and PATRC treatment group (added with 1 mmol/L Na2S2O4 and PATRC, containing 10 μM Rg1 in PATRC, HI + PATRC group). The incubation lasted for 24 h and the cells were digested with trypsin and collected by centrifugation (800 g, 5 min). The apoptosis rate of RBE4 in different group was analyzed according to the instructions of AnnexinV-FITC/PI apoptosis detection kit (BD, USA). Generally, 5 μl AnnexinV-FITC and 5 μl PI were added to incubate cells for 15 min at room temperature, and the apoptosis rate of the cells was detected by the flow cytometer (BD, Calibur, USA) within 1 h.
Tube formation assay under hypoxia condition
Matrigel (50 μL) was daubed at the bottom of 24-well plates at 37 °C and lasted for 30 min to become solidification state. RBE4 cells were cultured in ECM culture medium containing 10% FBS and 1% P/S. RBE4 (4 × 105) cells were obtained and treated with 10 μl PBS (control group). Na2S2O4 was used to mimic hypoxia condition in vitro. Under the hypoxia condition, RBE4 cells were treated with 1 mmol/L Na2S2O4 (HI group). The anti-hypoxia ability of Rg1 and PATRC was carried out as the following. Under the hypoxia condition (RBE4 cells treated with 1 mmol/L Na2S2O4), Rg1 (10 μM, HI + Rg1 group) or PATRC (containing 10 μM Rg1, HI + PATRC group) were added in the culture medium, respectively. After 10 h of culture, the formed tube quantities in different groups were calculated from 5 random fields.
In vitro cell targeting assay
In vitro cell targeting assay was implemented by flow cytometer and immunofluorescence methods. Under flow cytometer detection, RBE4 cells were cultured in a 12-well plate (8 × 104/well) at 37 °C and exposed to PBS (10 μL), iTP (10 μg/ml), TP (10 μg/ml), or PATRC (containing 10 μg/ml TP) for 30 min, respectively. Then, cells were washed with PBS for three times. Cells were harvested by trypsin digestion and the intensities of fluorescence were detected with flow cytometer (BD, Calibur, USA). Under immunofluorescence detection, cells were retained in plate; nucleus were dyed with DAPI solution (1 μg/ml) for 5 min and washed with PBS for 3 times; and then observed under a fluorescence microscope (Leica, DMI4000B, Germany).
Rat brain infarction model and administration
Male Sprague Dawley (SD) rats (n = 120, weight 250–270 g) participated in the study were purchased from Institute of Animal Research Center Affiliated to Nanjing University. Animal protocols were followed by the Ethics Committee of Nanjing University. Normal rats (n = 30) were chosen randomly as control group. The rest rats were used for the establishment of cerebral infarction model (n = 120). Brian infarction model was prepared as follows. Rats were anaesthetized with 3% pentobarbital sodium through rat tail vein injection. The left rat carotid was exposed by neck incision. The left carotid was inserted with 4–0 nylon filament and the filament was advanced to occlude the middle artery. Closed the incision and retrieved the filament 2 h later to restore brain blood flow. Before the recovery of the rats, a heating pad was used to maintain rat with temperature of 37 °C. After that, rats were free to obtain water and food. After the establishment of infarction model, control group (n = 30) were injected with PBS (1 ml) through tail venous. PBS (1 ml, n = 30), Rg1 (50 μmol/kg, 1 ml, n = 30) or PATRC (equal to 50 μmol/kg Rg1, 1 ml, n = 30) were injected into infarction model groups through tail venous, respectively. The injections were continued for 10 days with frequency of once a day.
PATRC brain distribution
As cerebral infarction model was established, the Rg1 permeability of different groups (n = 10) were tested. PBS (1 ml), Rg1 (50 μmol/kg, 1 ml) or PATRC (Rg1 equal to 50 μmol/kg, 1 ml) were injected through caudal vein, respectively. Rats were sacrificed by cervical vertebra dislocation 60 min or 480 min later. Brain tissue samples (1 mg) were homogenate into 5 ml buffer mixed with distilled water and methanol (1:1 volume). After centrifugation (12000 rpm) for 15 min, the supernatants were saved at −80 °C for HPLC detection and HPLC procedures were carried out as described as in Rg1 embedding efficacy part.
TTC staining
As cerebral infarction model was established for 10 days, cerebral tissues of different groups (n = 10) were sliced into 2 mm thick sections. Selections were dyed with 1% TTC solution for 30 min protected from light at 37 °C. Sections were photographed and the infarction volumes of brains were counted by Image J software.
Immunofluorescence of the brain microvessel
At the end of the experiment, the cerebral tissues in diverse groups (n = 10) were harvested from the identified cerebral infarction cortex, then fixed with 10% neutral formalin for paraffin-embedded [Citation28]. The embedded tissues were cut into 5 μm brain sections. The sections were carried out with dewax, rehydration and blocking (1% BSA). The nuclei were stained with DAPI solution and CD31 antibody (1:400) labelled with FITC was applied to detect the brain microvessel density. The microvessel images were collected with a fluorescence microscope (Olympus, Japan).
Statistical analysis
One-way ANOVA analysis was used to analyze data of diverse groups by Graph pad software. A p < .05 was considered as statistically significant. All data are presented as mean ± SD.
Results
PATRC characterization
The poor solubility in water and short biological half-life in vivo of Rg1 limit its clinic usage [Citation29]. In this research, PARTC was with high Rg1 encapsulation rate (45.37%) and encapsulated Rg1 can be protected from degradation. In this research, the morphology of PATRC was almost spherical with diameters of 122 ± 26 nm () through TEM detection. The mean size of PATRC was 132 ± 12 nm with PDI = 0.29 detected with DLS (). The size uniformity PATRC was great. The zeta potential is an important indicator of stable nano-materials. In aqueous condition, +30 mV or −30 mV was usually believed as the border [Citation30]. In aqueous condition, the zeta potential of PATRC was −38 mV, the strong zeta potential could prevent precipitation and keep stability.
Figure 1. PATRC characterization. (A) The nanometer of PATRC was tested by the size analyzer of dynamic light scattering (DLS); (B) The solution of PATRC was added onto the copper wire, and the morphology characterization was observed through dying with uranyl acetate for transmission electron microscopy (TEM).
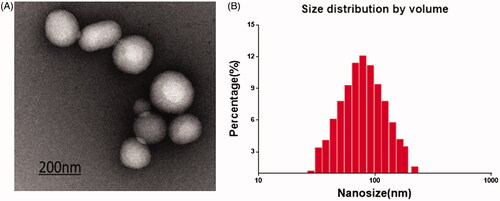
PATRC target RBE4 in vitro
To test whether PATRC obtained high enough targeting ability in vitro, the cellular uptake of PATRC by brain capillary endothelial cells, major component of BBB, was greatly investigated. Owing to the overexpressed TfR on the cellular membrane, stronger fluorescence signal was observed as RBE4 cells were incubated with PATRC () or TP peptide (). However, hardly any fluorescence signal could be detected as RBE4 treated with iTP (). The fluorescence intensities were further detected by flow cytometry method. The mean fluorescence intensity was 4.21 in control group (). As iTP treated with RBE4 the mean fluorescence intensity was 7.75 in and the mean fluorescence intensity was 97.41 as RBE4 treated with PATRC () group. Greater fluorescent signal (mean fluorescence intensity 105.32) was observed as RBE4 proceeded with incubation with TP (). In this study, PATRC could realize the brain microvascular endothelial cells targeting function. TfR was overexpressed on BBB [Citation31] and TfR targeted peptide could specifically bind to TfR and entry into the cell through endocytosis.
Figure 2. The RBE4 cell targeting of PATRC was detected by immunofluorescence. (A) RBE4 incubated with TP (10 μg/ml); (B) RBE4 incubated with PATRC (containing 10 μg/ml TP); (C) RBE4 incubated with iTP (10 μg/ml). Images were observed under a 20-fold objective lens. RBE4 targeting of PATRC was detected by flow cytometry method. (D,1) RBE4 cells incubated with PBS; (D,2) RBE4 incubated with iTP (10 μg/ml); (D,3) RBE4 incubated with PATRC (containing 10 μg/ml TP); (D,4) RBE4 incubated with TP (10 μg/ml).
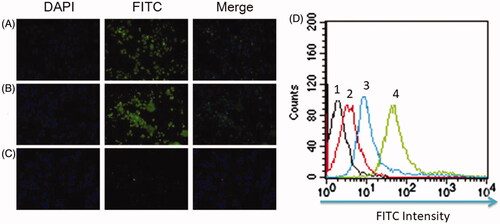
Figure 3. A flow cytometry was used to detect RBE4 apoptosis induced by hypoxia. RBE4 cells were divided into (A) PBS treatment group (10 μl PBS, control group); (B) hypoxia induction (1 mmol/L Na2S2O4, HI group); (C) hypoxia induction plus Rg1 treatment (1 mmol/L Na2S2O4 and 10 μM Rg1, HI + Rg1 group); (D) hypoxia induction plus PATRC treatment (1 mmol/L Na2S2O4 and 10 μM Rg1, HI + PATRC group).
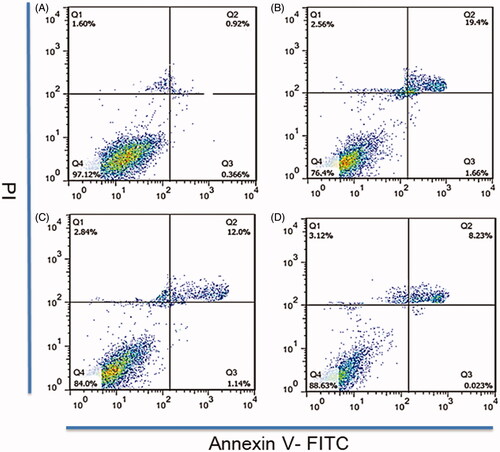
Figure 4. The RBE4 tube production in vitro. RBE4 cells were treated with matrigel for tube formation. (A) PBS treatment (10 μl PBS, control group); (B) under hypoxia condition, (1 mmol/L Na2S2O4, HI group); (C) under hypoxia condition, treated with Rg1 (1 mmol/L Na2S2O4 and 10 μM Rg1, HI + Rg1 group); (D) under hypoxia condition, treated with PATRC (containing 1 mmol/L Na2S2O4 and 10 μM Rg1, HI + PATRC group). After 8 h of incubation, then observed under a 4-fold objective lens; E. relative tube amount of RBE4. *p < .05, **p < .01.
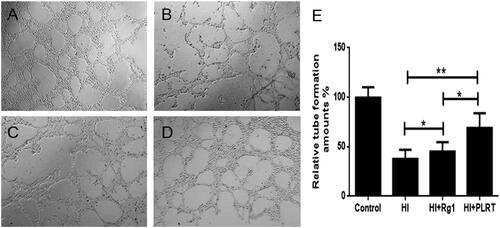
PATRC reduced the apoptosis of RBE4
Brain microvessels provided nutrients and oxygen for nervous tissue. After ischemia, the microvessels were blocked. Under hypoxia condition, the microvessels apoptosis or necrosis will occur. To revascularize blood vessels and to restore the blood perfusion of ischemic brain tissue could help improve the hypoxic state of ischemic tissue. The number of apoptotic cells in control group () was least (1.2%), and the rate of apoptosis in HI group () was the highest (21.6%). In HI + Rg1 treated group () the apoptosis rate was decreased (13.14%). Compared with the HI + Rg1 treatment group, the number of apoptotic cells decreased to 8.25% after PATRC treatment (). Under the acute cerebral infarction, angiogenesis abilities were reduced. The newly formed microvessels could implement neuron-protective and neuron-genesis function. Under hypoxic conditions, both PATRC and Rg1 decreased the apoptosis of RBE4 ability than HI group which could help improve the microcirculation in ischemic area, improve the hypoxic state of ischemic brain tissue, and reduce the cerebral tissue in infarct area in the early stage of acute cerebral infarction.
PATRC enhanced tube formation under hypoxic condition
To revascularize blood vessels and to restore the blood perfusion of ischemic brain tissue could help improve the hypoxic state of ischemic tissue. The protection on the brain microvessels and angiogenesis were important in clinic, because after cerebral infarction, the induced angiogenesis promoted regeneration of nerve tissue, and improved prognosis of the nervous system [Citation32–34]. Control group gained the most tube formation amounts (). Comparing with the control group, the tube formation amount in HI group () was decreased (p < .05). Comparing with the HI group, in HI + Rg1 group () treated with Rg1, the tube formation amount increased (p < .05). Comparing with the HI + Rg1 group, as RBE4 treated with PATRC (), the tube formation ability was also promoted (p < .01). PATRC also promoted the formation of capillary in the cerebral infarction region in vivo which was in virtue of the endocytosis mediated by TfR. The angiogenesis in cerebral infarction could aid the function recovery.
PATRC cross blood-brain barrier in vivo
HPLC had the characteristics of high pressure, high speed, high efficiency, high sensitivity and had become one of the most powerful analysis tools for the analysis and identification of drug distribution in vivo. In this research, HPLC method was used to identify whether PATRC could enhance the Rg1 accumulation in brain tissue. Poor brain tissue distribution (14.59 ± 3.24 ng/ml, 60 min) was detected in Rg1 treatment group. Nano drug delivery system could significantly improve the stability of drugs, improved the utilization rate of drugs, and improved the compliance of patients [Citation35–37]. Comparing with Rg1 treatment group, higher Rg1 distribution (158.85 ± 14.23 ng/ml) in PATRC treatment () group was detected on 60 min. Comparing with free Rg1 (19.28 ± 4.34 ng/ml) treatment group, much higher Rg1 concentration in rat brain tissue (297.43 ± 45.23 ng/ml) was detected in PATRC treatment group on 480 min which suggested that PATRC could effectively cross the BBB. In this research, we can detect that after the treatment of PATRC, Rg1 was highly enriched in brain which means that PATRC achieve the BBB penetration and enhanced the Rg1 brain distribution which was mainly due to the TfR mediated endocytosis.
Table1. BBB penetrating ability of PATRC in vivo.
PATRC decreased the ischemic lesion volumes
To investigate the therapeutic effect of PATRC in vivo, the rat cerebral ischemic model was established. Rats were sacrificed and the cerebral infarct volumes were detected using TTC staining. TTC staining was classical method to detect cerebral infarction areas. The normal tissue turned red, while ischemic tissues appeared pale. No ischemic lesion was detected in control group (). The ischemic volumes were 234 ± 40.67 mm3 () in model group (), 178.2 ± 27.75 mm3 in Rg1 treated group () and 100.6 ± 16.21 mm3 in PATRC group (), respectively. Comparing with the Rg1 treatment group, the ischemic volumes were markedly decreased as rat were treated PATRC (p < .05). Neuron-protection was important strategy for the treatment of cerebral infarction. Rg1 was with favourable neuron-protective effect, but cannot be transported to the brain. BBB blocks Rg1 entrance from the blood to the brain. The penetration of BBB was crucial for drugs to implement therapeutic effect. The more favourable therapeutic effect of PATRC than free Rg1 in cerebral infarction treatment mean that PATRC was with BBB penetrating ability.
Figure 5. TTC staining of brain tissues. Brains tissues were cut into 2 mm thick slides then immersed in 1% TTC at 37 °C for 30 min. The pale regions were infarction tissues and the red regions were normal tissues. (A) Control group, normal rats treated with PBS; (B) model group, cerebral infarction model group treated with PBS; (C) Rg1 group, cerebral infarction model group treated with Rg1 (50 μmol/kg); (D) PATRC group, cerebral infarction model group treated with PATRC (containing 50 μmol/kg Rg1); E. Cerebral infarction volume of different groups.*p < .05,**p < .01.
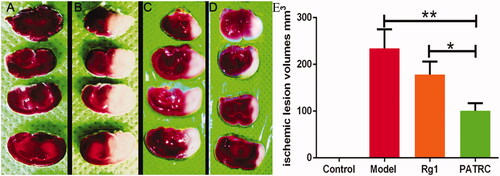
Table 2. Cerebral infarct volume of different groups detected by TTC staining.
PATRC promoted the microvessels distribution in infarction area
It was reported that stroke under hypoxia would bring about cell apoptosis, increased endothelial cell permeability, disassembled the tight junctions of brain vascular endothelial cell, and led to BBB disruption [Citation38]. The lesion of BBB was reversible at early stage but will become permanently inflamed few days later, which might bring about more severe lesions such as brain oedema and haemorrhage. Protection and regeneration of cerebral vessels was important for the recovery of cerebral infarction. Microvessel distribution densities in brain infarction tissue were tested by immunofluorescence method. There were most microvessels detected in control group (). As the infarction model was formed, the microvessels density was significantly decreased (). As infarction model group treated with Rg1, the microvessel density increased (). After the treatment of PATRC, comparing with Rg1 treatment group(), the microvessel density increased (p < .05), which indicated that PATRC treatment group played an superior role in the functional recovery of cerebral vessels than free Rg1. PATRC was with favourable targeting function on TfR which could achieve high Rg1 concentration in BBB.
Figure 6. The capillary of infarction tissue detected with immunofluorescence. Identified ischemic tissues were harvested; formalin fixed; paraffin embedded and cut into 5 μm for CD31 immunofluorescence. (A) Control group; (B) model group; (C) cerebral infarction model group treated with Rg1; (D) cerebral infarction model group treated with PATRC; (E) microvessels quantity in cerebral infarction area of different groups. Scale bar was 100 μm. *p < .05, **p < .01.
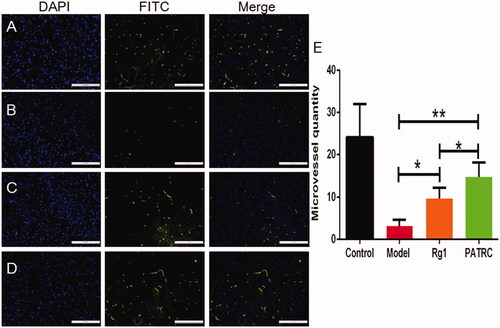
Discussion
Prompt and efficient treatment on cerebral infarction tissue with therapeutic agents was vital in the early stage of cerebral infarction, whereas BBB prevents drug entering into cerebral infarction tissue. Thrombolytic therapies were the clinical efficacy methods; however, the limited therapeutic time window made a fraction of patients benefit from effective thrombolytic effect. The limited self-repairing capacity of the cerebral tissue was considered to be the irreversible and progressive character of cerebral infarction. Neuro-protection was considered as interference to enhance the recovery of the neuron system, which was a vital goal for many clinical neurologists [Citation39]. Lots of researches ensured that neuroprotective measures were definitely feasible for cerebral infarction. To find the optimal approaches for clinical applications were with all sorts of difficulties and obstacles [Citation40,Citation41]. Many CNS treatment drugs were with prominent neuron protection effect while with poor brain distribution. The BBB played a protective role in the central nervous system and also obstructed the delivery of diagnostic and therapeutic drugs to the brain. Thus, the therapeutic efficacy for neuro-protection was greatly limited. Drug carriers traversing the brain barrier were rarely reported in the treatment of cerebral infarction. Brain microvascular endothelial cells were the major components of the BBB. Therefore, developing an elaborate strategy to achieve simultaneous BBB crossing and protection for stroke treatment were of great significance. Brain-targeted drug delivery system could highly enriched drug brain distribution. Although many brain-targeted drug delivery vectors have been reported, the treatments for cerebral infarction were merely reported [Citation42,Citation43]. In this research, PATRC could realize targeting effects on rat cerebral microvascular endothelial cells detected by flow cytometry and immunofluorescence assays in vitro. We also detected a stronger Rg1 brain distribution treated with PATRC in cerebral infarction model investigated by HPLC method in vivo. BBB restricted Rg1 penetrating brain tissues; the enhanced Rg1 brain distribution was strong evidence for BBB penetrating ability of PATRC.
After the occurrence of cerebral infarction, brain tissues were under hypoxia condition, brain microvascular necrosis and apoptosis occurred which led to brain tissue necrosis due to lack of oxygen and nutrient supply. At the same time, inflammation reaction would occur in the infarcted area, which further damaged brain tissue; therefore, reducing vascular apoptosis was with important clinic significance. In this research, PATRC exhibited stranger tube formation capacity under hypoxic state, comparing with free Rg1 treatment group in vitro. More microvessel in ischemic region resulted in the lower incidence and the longer survival time. Many researches revealed that angiogenesis after stroke had the potential research value [Citation44,Citation45]. PATRC enhanced the distribution of microvessels in infarction area detected by immunofluorescence in vivo. To revascularize blood vessels and to restore the blood perfusion of ischemic brain tissue could help improve the hypoxic state of ischemic tissue. Protection and regeneration of cerebral vessels were important for the recovery of cerebral infarction.
In our previous report, a brain target drug carriers were fixed with OX26 antibody with high affinity to TfR to implement BBB penetrating and improved the cerebral function of diabetic rats complicated with cerebral infarction [Citation15]. However, antibodies were prepared from heterologous species and the immunogenicity poses a high clinical risk. Peptides with high affinity to TfR were much smaller in size, compared with the antibody, and could realize deeper tissue penetration. What's more, peptides were easily synthesized and with economy advantages. In this research, PGA and chitosan were promising biomaterials [Citation46] to fabricate drug carriers because they were non-toxic, biodegradable and almost no immunogenicity which made them possess good tissue compatibility with human tissues and organs. The fixing of maleimide group enabled the drug carriers to conjugate with kinds of molecules with thiols in an effective and mild reaction which maintained good bioactivity of molecules. The therapeutic effect of peptide conjugated nanoparticle PATRC on cerebral infarction was no inferior to our previous research. Therefore, this work emphasized more effective tactics for the targeted treatment for cerebral infarction and may bring implications for the treatment of other neurodegenerative diseases.
Conclusion
We fabricated a novel TfR targeted drug carrier which loaded Rg1 with high drug loading efficiency and the targeting carrier could implement superior curative effect than Rg1 for cerebral infarction treatment. PATRC may provide a novel therapy for cerebral infarction treatment in clinic.
Disclosure statement
No potential conflict of interest was reported by the authors.
Additional information
Funding
References
- Lee SJ, Cheong JS, Ryu HU, et al. Recanalization of chronic occlusion of the middle cerebral artery with tissue plasminogen activator treatment: a case report. Clin Neuropharmacol. 2018;21:0000000000000282.
- Chen C, Mu X-Y, Zhou Y, et al. Ginsenoside Rg1 enhances the resistance of hematopoietic stem/progenitor cells to radiation-induced aging in mice. Acta Pharmacol Sin. 2014;35:143–150.
- Wang J, Xu H-M, Yang H-D, et al. Rg1 reduces nigral iron levels of MPTP-treated C57BL6 mice by regulating certain iron transport proteins. Neurochem Int. 2009;54:43–48.
- Wang J, Li D, Hou J, et al. Protective effects of geniposide and ginsenoside Rg1 combination treatment on rats following cerebral ischemia are mediated via microglial microRNA1555p inhibition. Mol Med Rep. 2018;17:3186–3193.
- Xie C-L, Li J-H, Wang W-W, et al. Neuroprotective effect of ginsenoside-Rg1 on cerebral ischemia/reperfusion injury in rats by downregulating protease-activated receptor-1 expression. Life Sci. 2015;121:145–151.
- Li YB, Wang Y, Tang JP, et al. Neuroprotective effects of ginsenoside Rg1-induced neural stem cell transplantation on hypoxic-ischemic encephalopathy. Neural Regen Res. 2015;10:753–759.
- Fernández-Moriano C, González-Burgos E, Iglesias I, et al. Evaluation of the adaptogenic potential exerted by ginsenosides Rb1 and Rg1 against oxidative stress-mediated neurotoxicity in an in vitro neuronal model. PLoS One 2017;12:e0182933.
- Xie CL, Wang WW, Xue XD, et al. A systematic review and meta-analysis of Ginsenoside-Rg1 (G-Rg1) in experimental ischemic stroke. Sci Rep. 2015;5:7790.
- Shen HH. Core concept: circumventing the blood-brain barrier. Proc Natl Acad Sci USA. 2017;114:11261–11263.
- Nogueira-Librelotto DR, Codevilla CF, Farooqi A, et al. Transferrin-conjugated nanocarriers as active-targeted drug delivery platforms for cancer therapy. CPD. 2017;23:454–466.
- Li H, Sun H, Qian ZM. The role of the transferrin-transferrin-receptor system in drug delivery and targeting. Trends Pharmacol Sci. 2002;23:206–209.
- Han L, Li J, Huang S, et al. Peptide-conjugated polyamidoamine dendrimer as a nanoscale tumor-targeted T1 magnetic resonance imaging contrast agent. Biomaterials. 2011;32:2989–2998.
- Yuan Q, Fu Y, Kao WJ, et al. Transbuccal delivery of CNS therapeutic nanoparticles: synthesis, characterization, and in vitro permeation studies. ACS Chem Neurosci. 2011;2:676–683.
- Li Y, He H, Jia X, et al. A dual-targeting nanocarrier based on poly(amidoamine) dendrimers conjugated with transferrin and tamoxifen for treating brain gliomas. Biomaterials. 2012;33:3899–3908.
- Shen J, Zhao Z, Shang W, et al. Ginsenoside Rg1 nanoparticle penetrating the blood-brain barrier to improve the cerebral function of diabetic rats complicated with cerebral infarction. IJN. 2017;12:6477–6486.
- Knezevic I, Kang HN, Thorpe R. Immunogenicity assessment of monoclonal antibody products: a simulated case study correlating antibody induction with clinical outcomes. Biologicals. 2015;43:307–317.
- Waldmann H. Human monoclonal antibodies: the residual challenge of antibody immunogenicity. Methods Mol Biol. 2014;1060:1–8.
- Loureiro JA, Gomes B, Fricker G, et al. Cellular uptake of PLGA nanoparticles targeted with anti-amyloid and anti-transferrin receptor antibodies for Alzheimer's disease treatment. Colloids Surf B Biointerfaces. 2016;145:8–13.
- Huang N, et al. PLGA nanoparticles modified with a BBB-penetrating peptide co-delivering Abeta generation inhibitor and curcumin attenuate memory deficits and neuropathology in Alzheimer's disease mice. Oncotarget. 2017;8:81001–81013.
- Sahin A, Yoyen-Ermis D, Caban-Toktas S, et al. Evaluation of brain-targeted chitosan nanoparticles through blood-brain barrier cerebral microvessel endothelial cells. J Microencapsul. 2017;34:659–666.
- Zhang L, Wang T, Li Q, et al. Fabrication of novel vesicles of triptolide for antirheumatoid activity with reduced toxicity in vitro and in vivo. Int J Nanomedicine. 2016;11:2663–2673.
- Geng X, Ye H, Feng Z, et al. Synthesis and characterization of cisplatin-loaded, EGFR-targeted biopolymer and in vitro evaluation for targeted delivery. J Biomed Mater Res A. 2012;100:2839–2848.
- Zhang L, Chang J, Zhao Y, et al. Fabrication of a triptolide-loaded and poly-γ-glutamic acid-based amphiphilic nanoparticle for the treatment of rheumatoid arthritis. Int J Nanomedicine. 2018;13:2051–2064.
- Gabriel CM, Keener M, Gallou F, et al. Amide and peptide bond formation in water at room temperature. Org Lett. 2015;17:3968–3971.
- Zhang L, Geng X, Zhou J, et al. Fabrication of poly(gamma-glutamic acid)-based biopolymer as the targeted drug delivery system with enhanced cytotoxicity to APN/CD13 over-expressed cells. J Drug Target. 2015;23:453–461.
- Luo L, Lü L, Lu Y, et al. Effects of hypoxia on progranulin expression in HT22 mouse hippocampal cells. Mol Med Rep. 2014;9:1675–1680.
- Jiang RG, Zhang XQ, Eyzaguirre C. Hypoxia induced by Na2S2O4 increases [Na+]i in mouse glomus cells, an effect depressed by cobalt. Experiments with Na+-selective microelectrodes and voltage-clamping. Brain Res. 1998;797:197–208.
- Zhao L, et al. Protective effect of rhGLP-1 (7-36) on brain ischemia/reperfusion damage in diabetic rats. Brain Res. 2015;30:153–159.
- Xu QF, Fang XL, Chen DF. Pharmacokinetics and bioavailability of ginsenoside Rb1 and Rg1 from Panax notoginseng in rats. J Ethnopharmacol. 2003;84:187–192.
- Bhattacharjee S. DLS and zeta potential – what they are and what they are not? J Control Release. 2016;235:337–351.
- Haseloff RF, Dithmer S, Winkler L, et al. Transmembrane proteins of the tight junctions at the blood-brain barrier: structural and functional aspects. Semin Cell Dev Biol. 2015;38:16–25.
- Liu J, Wang Y, Akamatsu Y, et al. Vascular remodeling after ischemic stroke: mechanisms and therapeutic potentials. Prog Neurobiol. 2014;115:138–156.
- Kumagai M, et al. A therapeutic angiogenesis of sustained release of basic fibroblast growth factor using biodegradable gelatin hydrogel sheets in a canine chronic myocardial infarction model. Heart Vessels. 2018;14:018–1185.
- Ergul A, Alhusban A, Fagan SC. Angiogenesis: a harmonized target for recovery after stroke. Stroke. 2012;43:2270–2274.
- Pardridge WM. The blood-brain barrier: bottleneck in brain drug development. Neurotherapeutics. 2005;2:3–14.
- Teichberg VI. From the liver to the brain across the blood-brain barrier. Proc Natl Acad Sci USA. 2007;104:7315–7316.
- Pardridge WM. Drug and gene delivery to the brain: the vascular route. Neuron. 2002;36:555–558.
- Ferdinand P, Roffe C. Hypoxia after stroke: a review of experimental and clinical evidence. Exp Transl Stroke Med. 2016;8:9–0023.
- Adibhatla RM, Hatcher JF, Tureyen K. CDP-choline liposomes provide significant reduction in infarction over free CDP-choline in stroke. Brain Res. 2005;5:1–2.
- Siasios I, Kapsalaki EZ, Fountas KN. Cerebral vasospasm pharmacological treatment: an update. Neurol Res Int. 2013;571328:31.
- Vergouwen MDI, Etminan N, Ilodigwe D, et al. Lower incidence of cerebral infarction correlates with improved functional outcome after aneurysmal subarachnoid hemorrhage. J Cereb Blood Flow Metab. 2011;31:1545–1553.
- Upadhyay RK. Drug delivery systems, CNS protection, and the blood brain barrier. Biomed Res Int. 2014;2014:869269.
- Patra JK, et al. Nano based drug delivery systems: recent developments and future prospects. J Nanobiotechnol. 2018;16:018–0392.
- Yin KJ, Hamblin M, Chen YE. Angiogenesis-regulating microRNAs and Ischemic Stroke. Curr Vasc Pharmacol. 2015;13:352–365.
- Li Q, He Q, Baral S, et al. MicroRNA-493 regulates angiogenesis in a rat model of ischemic stroke by targeting MIF. FEBS J. 2016;283:1720–1733.
- Yang Y, Wang S, Wang Y, et al. Advances in self-assembled chitosan nanomaterials for drug delivery. Biotechnol Adv. 2014;32:1301–1316.