Abstract
n-Butylidenephthalide (BP) is a potential anti-cancer drug, which can be extracted from Angelica sinensis (Danggui). Previous reports have shown the effectiveness of BP in treating cancer diseases. However, BP has no targeting capacity towards specific cancer cells. To improve treatment efficiency and reduce the dose of BP used in cancer treatment, targeting-based approaches should be developed. In the present study, we used riboflavin-5′-phosphate (RFMP) immobilized iron oxide magnetic nanoparticles (Fe3O4 MNPs) as carriers for BP to treat cancer cell lines derived from liver, prostate and breast. These model cancer cells overexpress riboflavin receptors on their cell membrane and are also sensitive to BP treatment. Thus, BP-binding free RFMP on MNPs can be used as probes to target these model cells, whereas BP can be readily released on target cancer cells. Cell viability was twofold lower by using Fe3O4@RFMP MNPs immobilized with BP than that achieved by using free-form BP at a similar amount. Moreover, BP-Fe3O4@RFMP MNPs have no apparent harmful effects on non-target cells. In addition, we evaluated the level of cysteine-aspartic acid protease 3 (caspase 3) in the resultant cell lysate obtained after treatment by BP-Fe3O4@RFMP MNPs to demonstrate that apoptosis is mainly involved in the growth inhibition of target cells.
Graphical Abstract
Introduction
Many medical treatments against cancer diseases are available nowadays. However, most of these treatments can cause adverse effects by damaging normal cells. To limit the potential damage caused by these treatments, targeting-based cancer treatments have become alternatives [Citation1]. Certain receptors and transporters, such as folate receptors [Citation2–14], glucose transporters [Citation15–17], transferrin receptors (TfR) [Citation18–21] and riboflavin (RF) receptors [Citation22–25], are highly overexpressed on the surface of certain cancer cells. Thus, these receptors can be used as target sites when employing targeting-based cancer therapy. For example, folate receptors are overexpressed on the cell membrane of ovarian [Citation2], cervical [Citation3], breast [Citation4], lung [Citation5], kidney [Citation6], colon/rectum [Citation7] and brain tumor cells [Citation8]. Thus, folate receptors have been used as targets in studies on drug delivery [Citation9,Citation10] and cell imaging against these target cancer cells [Citation11–14]. In addition, Warburg effect describes the phenomenon that cancer cells consume a larger amount of glucose and have a higher aerobic glycolysis rate than normal cells [Citation15,Citation16]. Cancer cells, including hepatocellular and breast cancer cells, overexpress glycolytic enzymes and the insulin-independent glucose transporter, that is, GLUT-1, on their cell membrane [Citation17]. In addition, TfR is overexpressed on the cell membrane of bladder-transitional cell carcinomas [Citation18], breast cancer cells [Citation19] and lung adenocarcinoma [Citation20]. Thus, TfR has also been taken as the targeted site for drug delivery [Citation21].
Another well-known receptor that is overexpressed on the breast [Citation22], prostate [Citation23] and liver [Citation24] cancer cells is the RF receptor. RF is responsible for electronic and optoelectronic properties in cellular metabolism [Citation25], while RF receptors on the cell membrane of cancer cells have been used as the target sites for anticancer drug delivery [Citation26–28]. For example, cancer drugs including doxorubicin [Citation27] and paclitaxel [Citation28] were delivered by micellar nanoparticles (NPs) and multi-walled carbon nanotubes, respectively. These nanocarriers were conjugated with RF to target RF receptors on target cancer cells. Although doxorubicin [Citation27] and paclitaxel [Citation28] are effective anticancer drugs, their high cost has caused financial burden for cancer patients. Thus, seeking alternative and inexpensive drugs to reduce the cost may help ease the financial burden of cancer patients.
Angelica sinensis (Danggui), a common Chinese medicinal herb, has been used to promote blood circulation [Citation29] to treat female menstrual disorders [Citation30] and menopausal symptoms [Citation31]. n-Butylidenephthalide (BP) is one of the active ingredients of A. sinensis with potential in treating multiforme [Citation32–35], breast cancer [Citation32], prostate cancer [Citation36,Citation37], oral squamous cell carcinoma [Citation38], lung cancer [Citation39] and hepatoma [Citation40].
Previous reports [Citation32–40] showed that BP can induce cell death of certain cancer cells through apoptosis. When cancer cells respond to apoptotic stimuli, the nerve growth factor IB, that is, Nur77, translocates from the nucleus to the mitochondria, where it binds to B-cell lymphoma 2. The binding of the nerve growth factor to B-cell lymphoma 2 induces a conformational change, which leads to the release of cytochrome c into cytoplasm. As a result, cysteine-aspartic acid protease (caspase) 9 and 3 are activated [Citation40,Citation41]. The activation of caspase 3 results in cell apoptosis. Given that A. sinensis is relatively inexpensive [Citation42] compared with known anti-cancer drugs, such as methotrexate [Citation26], doxorubicin [Citation27] and paclitaxel [Citation28], using BP in cancer treatment can potentially reduce the cost of medical treatment. Furthermore, the required dose of anticancer drugs can be reduced if targeting-based strategies can be further developed.
NPs were used as drug carriers in the past decade due to their small sizes and ease of modification. For instance, Fe3O4 magnetic NPs (MNPs) have good biocompability and are top choices in assisting drug delivery [Citation43]. We previously demonstrated the feasibility of using Fe3O4 MNPs immobilized with riboflavin-5′-phosphate (RFMP) as affinity probes to trap BP from the complex A. sinensis extract [Citation44]. RFMP is an RF derivative containing a phosphate and can be immobilized on the surface of Fe3O4 MNPs through Fe-phosphate chelation with a dissociation constant (Kd) of ∼6.14 × 10–6 M [Citation44]. In addition, RFMP contains polycyclic aromatic rings and can interact with BP through π–π interactions, wherein Kd between RFMP and BP was estimated to be ∼6.73 × 10–5 M [Citation44]. In this study, we immobilized RFMP on the surface of Fe3O4 MNPs and employed the aromatic ring on RFMP to interact with BP. The as-prepared BP-Fe3O4@RFMP MNPs were used as affinity probes to target the overexpressed RFMP receptors on the model cancer cells derived from prostate (LNCaP), liver (Hep G2) and breast (T-47D) cancer cells. Mouse embryonic fibroblast cells (NIH/3T3) were used as non-target model cells. BP free binding RFMP units on the MNPs were used to target these selected model cancer cells, while BP carried by Fe3O4@RFMP MNPs was delivered to the target cells to exert its anticancer function. The possible cause that led to the cell growth inhibition of model cancer cells using the developed approach was also discussed.
Experimental
Reagents and materials
Ammonium hydroxide solution (30–33%), 3-BP (≥96%), dimethyl sulfoxide (DMSO), 4-(2-hydroxyethyl) piperazine-1-ethanesulfonic acid, Dulbecco’s modified eagle medium-high glucose (DMEM), iron(III) chloride hexahydrate, kanamycin, minimum essential medium eagle (MEM), Roswell Park Memorial Institute (RPMI)-1640 medium (with l-glutamine), sodium bicarbonate, sodium pyruvate, tetramethylammonium hydroxide pentahydrate, triton X-100, Trypan blue solution (0.4%) and 3-(4,5-dimethylthiazol-2-yl)-3,5-diphenylformazan (MTT) were purchased from Sigma-Aldrich (St. Louis, MO, USA). Hydrochloric acid (36.5–38.0%) and dextrose were purchased from J. T. Baker (Phillipsburg, NJ, USA). Streptomycin sulfate and trifluoroacetic acid were purchased from Merck (Darmstadt, Germany). Sodium sulfite was purchased from Riedel–de Haën (Seelze, Germany). Phosphate-buffered saline (10×, without containing calcium/magnesium) buffer was purchased from Biowest (Nuaillé, France). Fetal bovine serum (FBS) and insulin (human recombinant) were obtained from Biological Industries (Kibbutz Beit Haemek, Israel). Bovine calf serum supplement was obtained from HyClone (USA). Hoechst 33342 dye was obtained from Life Technologies (Eugene, OR, USA). Prostate cancer cells (LNCap clone FGC, BCRC 60088), breast cancer cells (T-47D, BCRC 60250), hepatocellular cancer cells (Hep G2, BCRC RM60025) and mouse embryonic fibroblast cells (NIH/3T3, BCRC 60008) were purchased from the Bioresource Collection and Research Center (Hsinchu, Taiwan). Caspase-3/CPP32 fluorometric assay kit was purchased from BioVisin (Milpitas, CA, USA). Copper disks (200 mesh) coated with carbon (no 01800-F) were purchased from Ted Pella (Redding, CA, USA).
Instrumentation
A JEM 2000FXII transmission electron microscope (TEM) from JEOL (Tokyo, Japan) was used for investigation of the particle size and morphology of the as-prepared Fe3O4 MNPs. Ultraviolet–visible (UV–Vis) absorbance spectra were obtained using a Varian Cary 50 Bio UV–Vis spectrophotometer (Palo Alto, CA, USA). Fluorescence spectra were obtained using a Horiba Jobin Yvon FluoroMax-3 spectrophotometer (NJ, USA). Optical images were obtained using an Axio Imager M2 fluorescence microscope from Zeiss (Germany).
Generation of Fe3O4 MNPs
Iron(III) chloride (3.24 g) was added to a double-necked flask containing aqueous HCl (2 M, 6 mL) solution. Deionized water was added to reach the final volume of 50 mL. A vacuum pump was used to pump out the air in the flask. The flask was filled with nitrogen gas and injected with sodium sulfite solution (80 mM, 25 mL) and aqueous ammonia (5%, 22.5 mL) sequentially with a flow rate of 1.7 and 0.6 mL min−1, respectively, using a syringe pump at room temperature. The resultant mixture was continuously stirred at 70 °C for another 30 min. The generated Fe3O4 MNPs were collected by magnetic isolation and rinsed with deionized water (40 mL × 2). Tetramethylammonium hydroxide solution (2.5 mg mL−1, 20 mL) was added to the resultant MNP suspension (20 mL) and was stirred for 1 h in order to disperse Fe3O4 MNP. The resultant MNPs were magnetically isolated and rinsed with deionized water (40 mL × 2). The as-prepared Fe3O4 MNPs were re-suspended in deionized water (40 mL) and stored in a refrigerator at 4 °C before use.
Characterization of Fe3O4 MNP by TEM
When conducting TEM analysis, the generated Fe3O4 MNPs (∼1.7 μg mL−1, 2 μL) suspended in deionized water were deposited on a copper disk. The as-prepared disk was dried at room temperature for 24 h followed by eliminating water using a vacuum pump (10−3 Torr) for 48 h prior to TEM analysis.
Immobilization of RFMP onto Fe3O4 MNPs
Fe3O4 MNPs (∼0.1 mg) were mixed with RFMP (10−4 M, 0.2 mL) in aqueous solution containing 6 × 10−3% trifluoroacetic acid (TFA) (pH ∼3). Microwave-heating was used to facilitate sample preparation [Citation45,Citation46]. To accelerate the binding of RFMP onto the surface of Fe3O4 MNPs, the mixture was incubated in a microwave oven (540 W) and heated for 3 min. The resultant Fe3O4@RFMP MNPs were magnetically isolated and rinsed with aqueous solution containing 6 × 10−3% TFA (pH ∼3, 0.2 mL × 4). The supernatants containing RFMP obtained before and after microwave-heating were analyzed by fluorescence spectroscopy. The fluorescence intensity at 530 nm (λex = 450 nm) resulting from RFMP was recorded and used to estimate the binding amount of RFMP onto the surface of Fe3O4 MNPs.
Examination of the stability of RFMP on Fe3O4@RFMP MNPs in cell culture medium
To examine if RFMP could retain on the surface of Fe3O4 MNPs in complex culture media, we suspended and incubated Fe3O4@RFMP MNPs in different media (RPMI, MEM and DMEM) for a given time to investigate the remaining binding amount of RFMP on Fe3O4 MNPs. That is, Fe3O4@RFMP MNPs (∼0.1 mg) were suspended in different medium (0.2 mL) and incubated in an incubator at 37°C for 1, 3, 5, 10, 20, 30, 60, 120, 180 and 240 min. The resultant supernatants were collected and examined by fluorescence spectroscopy (λex = 530 nm) to estimate the released amount of RFMP.
Immobilization of BP on Fe3O4@ RFMP MNPs
Fe3O4@RFMP MNPs (∼0.1 mg) were mixed with BP (2.66 × 10−4 M, 0.2 mL) prepared in aqueous solution (pH ∼3) containing 6 × 10−3% TFA for different given times (1, 3, 5, 10, 20, 30, 60 and 90 min) with shaking (800 rpm) at room temperature. The resultant conjugates of BP and Fe3O4@RFMP MNPs (BP-Fe3O4@RFMP MNPs) were magnetically isolated and rinsed by aqueous solution (pH ∼3) containing 6 × 10−3% TFA (0.2 mL × 2). The collected supernatants including those obtained from the rinse solution were examined by UV–Vis absorption spectroscopy. The intensity at 236 nm derived from BP was recorded and used to estimate the binding amount of BP on the as-prepared Fe3O4@RFMP MNPs.
Cell culture
Different types of model cells were cultured in different media. T-47D and LNCaP cells were cultured in RPMI medium. DMEM was used as the medium to grow NIH/3T3 cells, whereas MEM was used as the medium for culturing Hep G2 cells. RPMI medium was prepared by dissolving one pack of RPMI-1640 powder (10.4 g), NaHCO3 (1.5 g), dextrose (4.5 g), 4-(2-hydroxyethyl) piperazine-1-ethanesulfonic acid (2.383 g), sodium pyruvate (0.11 g), kanamycin (0.1 g), streptomycin sulfate (0.1 g) and human insulin (10 mg) in deionized water (900 mL). DMEM was prepared by dissolving one pack of DMEM powder (13.4 g), NaHCO3(1.5 g), dextrose (4.5 g), kanamycin (0.1 g), streptomycin sulfate (0.1 g) and human insulin (10 mg) in deionized water (900 mL) under stirring. MEM was prepared by dissolving one pack of MEM powder (9.6 g), NaHCO3 (1.5 g), sodium pyruvate (0.11 g), kanamycin (0.1 g), streptomycin sulfate (0.1 g) and human insulin (10 mg) in deionized water (900 mL) under stirring. After completely dissolving, the medium solutions were filtered through a filter (pore size: 0.2 µm) and stored in a refrigerator at 4 °C before use. Furthermore, additional FBS (10%, 50 mL) was added to RPMI-1640 (450 mL) and MEM (450 mL), while bovine calf serum (10%, 50 mL) was added to DEME (450 mL) right before conducting cell culture. Cell culture was conducted in a Shel Lab incubator containing 5% CO2 at 37°C.
Cell cytotoxicity of BP and BP-Fe3O4@RFMP MNPs
To examine the cell toxicity of BP and BP-Fe3O4@RFMP MNPs, LNCaP was selected as the model cell. That is, LNCap (20,000 cells mL−1, 0.1 mL) were cultured in each well in a 96-well plate subjected in an incubator containing 5% CO2 at 37 °C for 1 day. Because BP was diluted by ethanol initially, different amounts of ethanol existed in the as-prepared BP samples. That is, BP with the concentration of 5.31 × 10−3 M was initially prepared in ethanol. Subsequently, the as-prepared BP sample (5.31 × 10−3 M) was diluted to 5.31 × 10−4 M by aqueous solution containing 10−3% TFA followed by serially diluted with the same acid solution with a dilution factor of 2. Thus, to observe the effect derived ethanol, different percentages (0, 0.625, 1.25, 2.5, 5 and 10%) of ethanol (0.1 mL) were used to treat the model cells. Moreover, BP (0.1 mL) with different concentrations (0, 3.32 × 10−5, 6.64 × 10−5, 1.33 × 10−4, 2.66 × 10−4 and 5.31 × 10−4 M were also used to treat model cell for 4 h at 37 °C. Fe3O4@RFMP MNPs immobilized with different amounts of BP were prepared by shaking Fe3O4@RFMP (4 mg mL−1, 0.1 mL) with BP (2.66 × 10−4 M, 0.2 mL) for 3, 10, 15 and 30 min. After incubating for 30 min, Fe3O4@RFMP MNPs were bound with BP to the maximum amount. However, if the incubation times were 3, 10 and 15 min, 30, 50 and 70% to the maximum binding amount of BP, respectively, on Fe3O4@RFMP were reached. The as-prepared Fe3O4@RFMP MNPs (0.1 mg) immobilized with different amounts of BP were added to the model cells on the 96-well plate and incubated at 37 °C for 4 h. After 4 h, the media were removed and new media were added to the cells followed by incubation in the same incubator (5% CO2) for 72 h.
To examine the cell viability, MTT (1 mg mL−1, 0.1 mL) prepared in RPMI-1640 medium was added to the resultant cell samples after removing old media followed by incubated at 37 °C for 4 h. DMSO (99.9%, 0.15 mL) was then added to each cell sample to release the dye under shaking for 30 s. The dye released from the cells was collected and examined by UV–Vis absorption spectroscopy. The absorbance difference at the wavelengths between 560 and 630 nm (as background signal) obtained in the resultant absorption spectra of the samples was recorded and used to estimate the cell viability.
Observation of the cellular uptake of functional Fe3O4 MNPs by model cells under optical microscopy
Cancer cell models (20,000 cells mL−1, 1 mL) were loaded on a glass coverslip and were placed in a six-well plate and cultured at 37 °C for 24 h. The cancer cell models were rinsed after culturing. A new media containing Fe3O4 MNPs (4 mg mL−1), Fe3O4@RFMP MNPs (4 mg mL−1) and BP-Fe3O4@RFMP MNPs (4 mg mL−1) immobilized with 50% of the maximum BP binding amount on the MNPs were added to individual cell samples. These cells were incubated at 37 °C for another 4 h. The media were removed after 4 h, and new media were added to the cell samples followed by incubation for 68 h.
Trypan blue was used to stain the resultant cells. Dead cells allowed trypan blue to penetrate the cell membrane resulting in cells with blue colored stains. Thus, cell viability was visualized under an optical microscope. Trypan blue (0.4%, 0.5 mL) was prepared in an aqueous NaCl (0.81%) and K2HPO4 (0.06%) solution and was mixed with a specific media (0.5 mL). The mixture was added to the cells prepared above and was cultured for 72 h. The samples were incubated in an incubator containing 5% CO2 at 37 °C for 30 min. The resultant cells stained with Trypan blue were rinsed with an aqueous NaCl (0.9%) (1 mL × 3) solution and were fixed by paraformaldehyde (4%) prepared in aqueous NaCl (0.9%) solution for 30 min. The cells were rinsed with aqueous NaCl (0.9%, 1 mL × 3) solution and were treated with a blocking buffer (aqueous solution containing NaCl (0.9%), FBS (10%) and Triton X-100 (0.25%) at 37 °C for 30 min. The nuclei of the cells were stained with Hoechst 33342 dye (2 mg μL−1, 100 μL) and incubated at 37 °C for 30 min. The cells were rinsed with washing buffer containing NaCl (0.9%) and triton X-100 (0.25%) (1 mL × 3). Finally, the cells on glass cover slips were covered with a microscope slide deposited with a drop of glycerol (80%, 20 μL) was sealed with nail varnish. The samples were investigated under an optical microscope.
Model cells treated by BP-Fe3O4@RFMP MNPs
The effectiveness of using the as-prepared BP-Fe3O4@RFMP MNPs to treat cancer cells was conducted by using LNCaP, T-47D and Hep G2 cells were used as model cells and NIH/3T3 cells were selected as nontarget cells. The cells (20,000 cells mL−1, 0.1 mL) were cultured in a 96-well plate at 37 °C for 24 h. The cells treated with and without Fe3O4 MNPs, Fe3O4@RFMP MNPs and Fe3O4@RFMP MNPs loaded with different amounts of BP were examined. The model cells (20,000 cells mL−1, 0.1 mL) cultured in their specific media were incubated at 37 °C for 12 h prior to addition of different MNPs. The final concentration MNPs in the cell samples was 4 mg mL−1. The cells were cultured at 37 °C for 0.5, 1, 4, 24, 30 and 40 h. After incubation, the media were removed and new media (0.1 mL) were added followed by continuous incubation until the total incubation time reached 72 h. MTT assay was used to examine the viability of cells after treatment. After removing the old media from the cell samples, the resultant cells were incubated with MTT (1 mg mL−1, 0.1 mL) in 96-well plates for 4 h in an incubator (5% CO2) at 37 °C. After incubation, the old media were removed and DMSO (99.9%, 0.15 mL) was added to each well to release the dye. After standing for 30 s, the dye released from the wells was collected and examined by UV–Vis absorption spectroscopy. The absorbance difference between 560 and 630 nm (as background signal) obtained in the resultant absorption spectra of the samples was recorded and used to estimate cell viability.
Examination of activity of caspase 3
We further used caspase-3/CPP32 fluorometric assay kit to examine if the death of model cancer cells was related to activation of caspase 3. Briefly, LNCap, Hep G2, T-47D and NIH/3T3 cell (20,000 cells mL−1, 0.1 mL) were cultured in their specific media in a 96-well plate for 1 day. Ethanol (0.5%, 0.1 mL), BP (2.66 × 10−4 M contain 0.5% ethanol, 0.1 mL), Fe3O4 MNPs (4 mg mL−1, 0.1 mL), Fe3O4@RFMP MNPs (4 mg mL−1, 0.1 mL) and BP-Fe3O4@RFMP@BP MNPs (4 mg mL−1, 0.1 mL) prepared in specific media were added to the individual model cells and incubated at 37 °C for 4 h. Old media were removed, and new media (0.1 mL) were then added to the cell samples. The samples were continuously incubated in the same incubator for another 68 h. Three replicates were conducted.
To collect sufficient cells, three cell samples treated above were pooled together. The old media from the resultant cells (20,000 cells mL−1, 0.3 mL) were eliminated using centrifugation (2000 rpm, 5 min). The settled down cells were added with chilled cell lysis buffer (50 μL) and incubated in an ice bath for 10 min. Reaction buffer (50 μL) (containing 10 mM DTT) provided by the assay kit was twofold diluted and added to each cell sample. DEVD-AFC (AFC: 7-amino-4-trifluoromethyl coumarin) substrate (5 μL, 1 mM) was added to the samples above and incubate at 37°C for 2 h. After incubation, the samples were centrifuged at 2000 rpm for 5 min and the supernatant was examined by fluorescence spectroscopy (λex = 400 nm).
Results and discussion
Generation and characterization of the Fe3O4 MNPs and Fe3O4@RFMP MNPs
Fe3O4 MNPs were used as the nanocarriers to deliver drugs in this study. Thus, we first generated Fe3O4 MNPs by reacting aqueous FeCl3 with aqueous sodium sulfide under alkaline conditions. shows the TEM image of the as-prepared Fe3O4 MNPs, which were aggregated together under TEM observation. RFMP was used to trap BP on Fe3O4 MNPs. Thus, RFMP (0.2 mL, 10−4 M) was initially immobilized on the surface of the as-prepared Fe3O4 MNPs (∼0.1 mg) through metal-phosphate chelation under microwave-heating (power: 540 W) for 3 min [Citation44]. The as-prepared MNPs were rinsed to remove unbound species. The Kd of Fe3O4@RFMP MNPs was estimated as ∼6.14 × 10−6 M [Citation44]. Supplementary Figure S1 shows the fluorescence spectra (λex = 450 nm) of the supernatants containing RFMP obtained before and after incubation with Fe3O4 MNPs. The binding capacity of RFMP onto Fe3O4 MNPs at 530 nm rinsed with the solution from unbound RFMP was estimated to be ∼161.10 ± 3.10 nmol mg−1 is also shown in Supplementary Figure S1, This result was similar to that of a previous study [Citation44].
Figure 1(B) shows the TEM image of the as-prepared Fe3O4@RFMP MNPs. Similar to the image shown in Figure 1(A), Fe3O4@RFMP MNPs were aggregated.
Examination of stability of RFMP on Fe3O4 MNPs
RFMP was immobilized on the surface of Fe3O4 MNPs based on chelation. Thus, it is important to investigate the stability of RFMP on MNPs. In addition, given that we employed RFMP on Fe3O4 MNPs as the linker to bind BP onto the surface of Fe3O4@RFMP MNPs. Therefore, it is also necessary to examine the stability of RFMP on Fe3O4 MNPs. BP bound Fe3O4@RFMP MNPs since these were used to treat model cells in complex cell culture media. Thus, RPMI-1640, DMEM and MEM were used as solvents to examine the stability of RFMP on the as-prepared Fe3O4@RFMP in this complex medium. Supplementary Figure S2 shows the percentage of RFMP (RFMP%) that remained on Fe3O4@RFMP MNPs after incubated with Fe3O4@RFMP MNPs (0.1 mg) in different media (0.2 mL) including RPMI, DMEM and MEM at different times (1, 3, 5, 10, 20, 30, 60, 120, 180 and 240 min). RFMP was released right after Fe3O4@RFMP MNPs were incubated with these media. Nevertheless, ∼46.9 ± 1.9, 47.2 ± 1.5 and 48.9 ± 0.9% RFMP still retained on Fe3O4 MNPs after incubated with RPMI, DMEM and MEM, respectively, for 1 h. These results were understandable because the kinetic equilibrium of RFMP between the media and surface of Fe3O4 MNPs was reached after 1 h. The results also implied that BP on RFMP immobilized on Fe3O4 MNPs can be gradually released from the MNPs during incubation with model cells. Furthermore, ∼50% RFMP could still be retained on the surface of Fe3O4 MNPs after incubating in the complex media.
Figure 2. (A) Plot of the binding amount of BP (2.66 × 10–4 M, 0.2 mL) onto Fe3O4@RFMP MNPs (0.1 mg) versus the incubation time. (B) The corresponding UV–Vis absorption spectra of the sample containing BP obtained before and after binding with Fe3O4@RFMP MNPs followed by rinse with the solution containing 6 × 10−3% TFA (pH ∼3) two times (W1 and W2). The incubation time was 10 min.
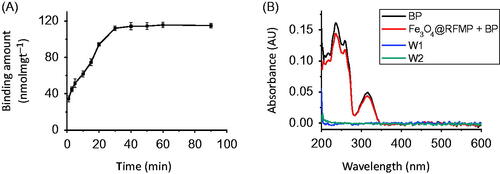
BP immobilized on Fe3O4@RFMP MNPs
BP contains an aromatic ring, which can interact with RFMP on Fe3O4@RFMP MNPs through π–π stacking with a Kd of ∼6.73 × 10−5 M [Citation44]. We immobilized BP onto the surface of Fe3O4@RFMP MNPs by shaking BP (2.66 × 10−6 M, 0.2 mL) with Fe3O4@RFMP MNP (0.1 mg) at different times. shows the resulting curve by plotting the binding amount of BP onto Fe3O4@RFMP MNPs obtained from different incubation times wherein this was conducted in triplicates. The maximum binding amount of BP onto Fe3O4@RFMP MNPs was 112.1 ± 2.6 nmol mg−1, which was achieved after 30 min. Supplementary Figure S3 shows the TEM image of BP-Fe3O4@RFMP MNPs. The as-prepared MNPs looked similar to that observed in . shows the representative absorption spectra of the samples obtained before (black) and after (red) shaking Fe3O4@RFMP MNPs (0.1 mg) with BP (2.66 × 10−6 M, 0.2 mL) for 10 min. The maximum binding amount of BP onto Fe3O4@RFMP was 61.9 ± 3.3 nmol mg−1, which was about 50% maximum binding amount of BP onto the surface of Fe3O4@RFMP MNPs.
Figure 3. Examination of cell cytotoxicity of BP-Fe3O4@RFMP MNPs. Cell viability obtained by incubating BP-Fe3O4@RFMP MNPs (4 mg mL−1) with (A) LNCaP cells (∼20000 cells mL–1, 0.1 mL), (B) Hep G2 cells (∼20000 cells mL–1, 0.1 mL), (C) T-47D cells (∼20000 cells mL–1, 0.1 mL) and (D) NIH/3T3 cells (∼20000 cells mL–1, 0.1 mL) for different times.
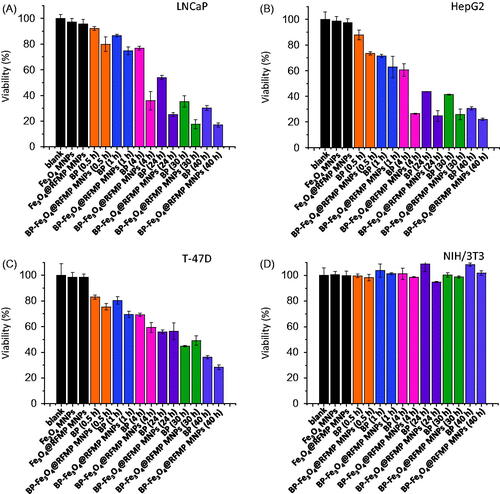
Cancer cells treated by BP-Fe3O4@RFMP MNPs
We proposed that RFMP and BP on the as-prepared BP-Fe3O4@RFMP MNPs can be used to probe and treat target cancer cells, respectively. Thus, we employed BP-Fe3O4@RFMP MNPs that were bound with ∼33, ∼58, ∼77 and ∼114 nmol mg−1 of BP, which corresponded to 30, 50, 70 and 100% to the maximum BP binding amounts, respectively, on MNPs, to treat model cells. LNCaP cells, which have been demonstrated to have RF receptors [Citation23] and sensitive to BP treatmet [Citation37], were selected as model cells. The cells treated with free BP and BP-Fe3O4@RFMP MNPs prepared in RPMI were conducted at 37 °C for 4 h followed by replacing old media with new one. The model cell samples treated with free BP and BP-Fe3O4@RFMP MNPs that both contained a similar amount of BP were conducted in parallel. All the samples were incubated at 37 °C for 72 h including the treatment time with BP/BP-Fe3O4@RFMP MNPs. MTT assay was used to evaluate the cell viability after treatment. Supplementary Figure S4 shows the bar graphs that represented the cell viability obtained after treating the model cells with BP and BP-Fe3O4@RFMP MNPs in parallel. Similar amounts of BP were used in each pair (marked with the same color). Apparently, the most visible difference of the cell viability treated by the pair of BP/BP-Fe3O4@RFMP MNPs was observed when BP concentration in the cell sample (0.1 mL) reached ∼2.32 × 10−4 M (orange bar), which corresponded to 50% maximum binding amount of BP (∼58 nmol mg−1) on the MNPs (0.4 mg). The cell viability was ∼35% after the cells (0.1 mL) were treated by BP-Fe3O4@RFMP MNPs (0.4 mg) that contained ∼23.2 nmol of BP. However, the cell viability was 70% when cells were treated with ∼2.66 × 10−4 M of free-BP (orange bar). Although the concentration of free form BP was slightly higher than that on the MNPs, the cell viability is higher than that obtained from BP-Fe3O4@RFMP MNPs containing ∼2.32 × 10−4 M of BP. The presence of free RFMP and a sufficient amount of BP on BP-Fe3O4@RFMP MNPs in the inhibition of the cell growth of the model cells are important. Moreover, BP-Fe3O4@RFMP MNPs were more effective than using only the free-form BP for cell treatment. No apparent cytotoxicity was observed towards the cells treated with Fe3O4@RFMP MNPs alone (blue bars, Supplementary Figure S4). According to the results shown in Supplementary Figure S4, the viability of the cells treated by BP-Fe3O4@RFMP MNPs was lower than those treated by free-form BP with a similar amount of BP. These results corresponded with our initial assumption that the presence of RFMP on MMPs can target cells such as LNCaP cells containing RF receptors and can help the MNPs to bind onto the target cells. Therefore, the required amount of BP in inhibiting cell growth can be reduced. On the basis of these results, BP-Fe3O4@RFMP MNPs containing 50% maximum BP binding amount on the MNPs were further used to treat selected model cells in the following experiments.
Figure 4. Bright field microscopic images obtained by treating LNCaP (A–H), Hep G2 (I–P), T-47D (Q–X) with media only (A, I and Q), Fe3O4 MNPs (∼4 mg mL−1) (B, J and R), Fe3O4@RFMP MNPs (∼4 mg mL−1) (C, K and S) and BP-Fe3O4@RFMP MNPs (∼4 mg mL−1) (D, L and T) for 4 h followed by culturing another 68 h after renewing the media, then rinsed with aqueous NaCl (0.9%,1 mL ×3) and stained with Trypan blue and Hoechst 33342 dye before observation under microscopy. Panels (E)–(H), (M)–(P) and (U)–(X) were the corresponding images obtained under fluorescence microscopy. The scale bar is 10 μm. The exposure time was 6 ms.
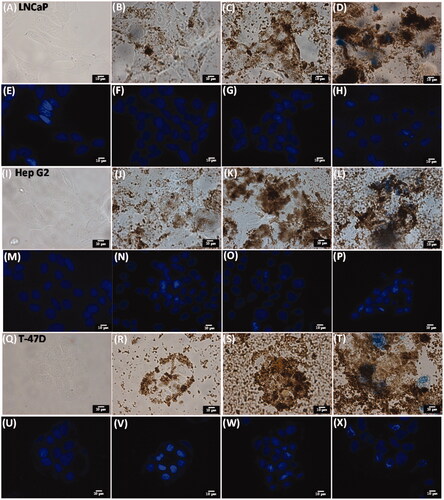
LNCaP, T-47D and Hep G2 cells reportedly have RF receptors [Citation22–24] and are also sensitive to the presence of BP treatment [Citation37,Citation40,Citation47], were selected as the model cells. However, no previous studies reported that NIH/3T3 cells have RF receptors or are sensitive toward BP treatment. Thus, NIH/3T3 was selected as non-target model cells. The cells were treated with different ways at different times (0.5–40 h) initially followed by renewing the media after treatment. However, the total incubation time and treatment time was the same, that is, 72 h. MTT assay was conducted to examine the cell viability after treatment. shows the results of cell viability of the LNCaP, Hep G2 and T-47D, respectively, after different treatments. The viability of the cells treated by Fe3O4 MNPs and Fe3O4@RFMP MNPs (black bars) was similar to the cells without treatment (marked as blank and black bar). However, the viability of the cells treated by BP-Fe3O4@RFMP MNPs was lower than those treated by free-form BP even though the concentration of BP in the cells was similar in both treatments. The most apparent difference of the cells treated by BP and BP-Fe3O4@RFMP MNPs occurred when the incubation time during the treatment was extended to 4 h (rose bars). The cell viability of LNCaP and HepG2 cells treated by BP-Fe3O4@RFMP MNPs was twofold lower than those treated by the same amount of free-form BP. Nevertheless, the effect towards T-47D was not so apparent. The viability of cells treated by BP and BP-Fe3O4@RFMP MNPs was reduced as the treatment time was extended more than 24 h. Nevertheless, the viability of the cells treated by BP-Fe3O4@RFMP MNPs was lower than those treated with similar amounts of BP in free form. In addition, the cell viability of NIH/3T3 remained 100% after treated by different ways (). NIH/3T3 cells were insensitive to the presence of BP, so BP did not cause apparent damage on the cells. The results indicated that BP-Fe3O4@RFMP MNPs were effective towards LNCaP and Hep G2 cells, while were less effective to T-47D cells. Moreover, BP and BP-Fe3O4@RFMP MNPs did not cause any apparent damage on NIH/3T3 cells, which do not possess RF receptors or any sensitivity to BP. We have successfully demonstrated that BP-Fe3O4@RFMP MNPs can be used as effective anticancer agents for certain model cells (LNCaP and Hep G2), which have RF receptors and sensitive to BP treatment. Moreover, the required amount of BP for cancer cell treatment can be reduced by using Fe3O4@RFMP MNPs as carriers.
Observation of cell viability of the cells stained by trypan blue
To further investigate the binding condition between functional MNPs with the cells and the cell viability with and without any treatments, optical microscopy was used to observe the cells incubated with functional MNPs. Cells (∼20,000 cells mL–1, 1 mL) including LNCaP, Hep G2, T-47D and NIH/3T3 cells were treated by different ways for 4 h. The media in the cell samples were renewed after 4 h, and the cell samples were continuously incubated for another 68 h. The resultant cells were stained with Trypan blue, and the cell nuclei were also stained with Hoechst 33342 dye. shows the resultant optical microscopic images of LNCaP cells alone and treated by Fe3O4 MNPs, Fe3O4@RFMP MNPs and BP-Fe3O4@RFMP MNPs, respectively, observed by optical microscopy under bright field. shows the corresponding fluorescence images. Apparently, the binding resulting from Fe3O4@RFMP MNP () attachments on the cells was more apparent than those from Fe3O4 MNPs (). The cell nuclei can be clearly observed in . Moreover, blue stained cells are observed in , which was obtained by treating LNCaP cells with BP-Fe3O4 MNPs. That is, the cells treated with BP-Fe3O4@RFMP MMPs were damaged. The results obtained from treating HepG2 () and T-47D () cells with the same treatments as LNCaP cells were also similar to the results shown in . These results show that more Fe3O4@RFMP and BP-Fe3O4@RFMP MNPs can bind to the model cells than unmodified Fe3O4 MNPs. Moreover, only BP-Fe3O4@RFMP MNPs can cause apparent damage on the model cells. Nevertheless, no apparent damage occurred on NIH/3T3 cells (Supplementary Figure S5) that had been treated with the same treatments as shown in . The binding affinity of different MNPs toward NIH/3T3 cells was similar (Supplementary Figures S5(B–D)). Moreover, no damage was found on cells treated with BP-Fe3O4@RFMP MNPs since no apparent blue staining was observed under optical microscopy. These results corresponded to that obtained in . BP-Fe3O4@RFMP MNPs caused damage on their target cells. The results suggested that BP-Fe3O4@RFMP MNPs can be used as anticancer agents to their target cells and do not cause apparent damage on non-target cells.
Figure 5. Investigation of caspase 3 activity. Bar graphs of (A) LNCap, (B) Hep G2, (C) T-47D and (D) NIH/3T3 cells obtained by plotting the ratio of the fluorescence intensity at 505 nm of the resultant fluorescence spectra obtained after conducting different treatments followed by the caspase 3 activity assay to that obtained from the control samples (blank) according to the results shown in Supplementary Figure S6.
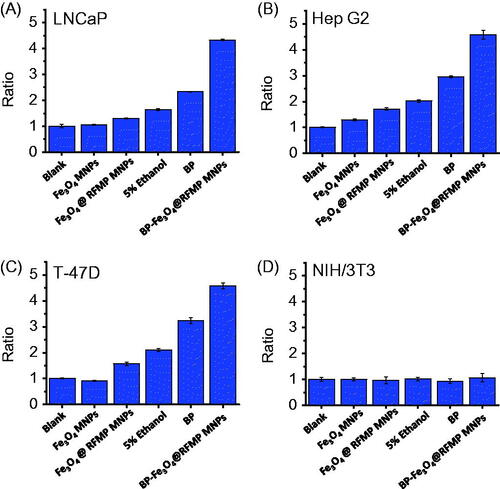
Cell apoptosis caused by functional MNPs
BP can induce their target cells to death through apoptosis pathway [Citation40,Citation41]. Caspase 3 is activated during apoptosis [Citation40,Citation41]. To confirm whether apoptosis was also involved in the cell death of the model cells using our treatments, we investigated the activity of caspase 3 using a caspase-3/CPP32 fluorometric assay kit. Caspase 3 can cleave the substrate, that is, blue fluorescent DEVD-AFC (AFC: 7-amino-4-trifluoromethyl coumarin) to release AFC and would obtain a yellow-green fluorescence (λmax = 505 nm) product under light excitation (λex = 400 nm) [Citation48,Citation49]. The increase of caspase 3 activity can be determined by comparing the fluorescence intensity of AFC at 505 nm from an apoptotic sample with the control cell sample without being treated, the increase of caspase 3 activity can be determined. Supplementary Figure S6(A–C) shows the resultant fluorescence spectra of the cell lysates of LNCaP, Hep G2 and T-47D, respectively, obtained after treatment of cells with ethanol (red), BP (blue), Fe3O4 MNPs (green), Fe3O4@RFMP MNPs (pink) and BP-Fe3O4@RFMP MNPs (olive green) followed by conducting caspase 3 activity assays. The fluorescence intensity of the cells without treatments (black) had the lowest intensity at 505 nm. The fluorescence spectra obtained from the cells treated by Fe3O4 MNPs (green) were overlapped with the control samples (black) without treatments, indicating that Fe3O4 MNPs were not harmful to these model cells. The maximum emission band of the fluorescence spectra of cell samples treated with Fe3O4@RFMP MNPs shifted to ∼515 nm. This shows that RFMP, with the maximum emission band at 530 nm, was released from the MNPs and contributed to the resultant fluorescence spectra during the assays. Model cells and BP samples were treated with 5% ethanol. The fluorescence intensity at 505 nm was higher (red) than that of the control samples (black). Nevertheless, the results were lower than that obtained by the cells treated with BP (blue). The samples treated by BP-Fe3O4@RFMP MNPs showed the highest fluorescence intensity at 515 nm (olive green). The caspase 3 activity was highest after the cells were treated by BP-Fe3O4@RFMP MNPs. However, no apparent effects occurred on NIH/3T3 cells after any treatment (Supplementary Figure S6(D)). Moreover, BP (2.66 × 10−4 M) used in the treatment still contains 5% ethanol. The level of the caspase 3 activity in the samples treated by BP was partly contributed by ethanol. This was confirmed by comparing the results obtained from cells treated with 5% ethanol.
Moreover, BP-Fe3O4@RFMP MNPs caused relatively high damage on these target model cells than BP alone through apoptosis. These results corresponded to the results obtained in and and Supplementary Figure S4. shows the summarized bar graphs by comparing the results from those obtained from LNCaP, Hep G2, T-47D and NIH/3T3 cells without conducting any treatments (marked blank). The ratio labeled on Y-axes in was obtained by dividing the fluorescence intensity at 505 nm obtained from different treatments to the blank obtained without any treatments. Evidently, the cell samples including LNCaP, Hep G2 and T-47D treated by BP-Fe3O4@RFMP MNPs had the highest ratio, indicating that the cell death was mainly caused through apoptosis.
Conclusions
Cancer treatment is usually costly. Thus, targeting-based drug delivery has been used to improve treatment efficiency and to reduce the cost. In this study, we have successfully demonstrated a targeting-based approach by using RF receptors that are usually overexpressed on several cancer cells including human prostate (e.g., LNCap), breast (e.g., T-47D) and hepatocellular carcinoma cells (e.g., Hep G2) as targeting sites. BP has been reported to have anti-cancer capability towards human prostate, breast and hepatocellular cancer cells. Thus, BP was selected as the anticancer agent, which was carried by RFMP immobilized on the as-prepared Fe3O4@RFMP MNPs. The generated BP-Fe3O4@RFMP MNPs can be used to effectively target and inhibit the cell growth of LNCaP and Hep G2 cells. However, they were less effective toward T-47D cells. Furthermore, the cell growth inhibition rate of BP-Fe3O4@RFMP MNPs is higher than that of free-form BP when a similar amount of BP was used to treat the same model cells. Moreover, our results demonstrated that the as-prepared functional MNPs have no apparent toxicity toward non-target cells, that is, NIH/3T3. That is, the developed approach can be specifically used to probe target cells and deliver BP to the target sites. In this way, the amount of BP used to treat cancer cells can be reduced, therefore reducing the cost of treatment. These results suggested that the developed BP-Fe3O4@RFMP MNPs have the potential to be used as anti-cancer agents. Nevertheless, in vivo studies should be examined to further realize the practice of the developed approach.
Supporting_Information_BP_AN_revision.docx
Download ()Acknowledgements
We thank Prof. Pawel Urban and Mr. Rakesh Prabhu for loaning us the optical microscope and helping us to obtain the optical images of cell samples.
Additional information
Funding
References
- Saini RK, Chouhan R, Bagri LP, et al. Strategies of targeting tumors and cancers. J Can Res Updates. 2012;1:129–152.
- Kalli KR, Oberg AL, Keeney GL, et al. Folate receptor alpha as a tumor target in epithelial ovarian cancer. Gynecol Oncol. 2008;108:619–626.
- Pillai MR, Chacko P, Kesari LA, et al. Expression of folate receptors and heterogeneous nuclear ribonucleoprotein E1 in women with human papillomavirus mediated transformation of cervical tissue to cancer. J Clin Pathol. 2003;56:569–574.
- Leone JP, Bhargava R, Theisen BK, et al. Expression of high affinity folate receptor in breast cancer brain metastasis. Oncotarget. 2015;6:30327–30333.
- Shi H, Guo J, Li C, et al. A current review of folate receptor alpha as a potential tumor target in non-small-cell lung cancer. Drug Des Dev Ther. 2015;9:4989–4996.
- Weitman SD, Lark RH, Coney LR, et al. Distribution of the folate receptor GP38 in normal and malignant cell lines and tissues. Cancer Res. 1992;52:3396–3401.
- Yang SJ, Lin FH, Tsai KC, et al. Acid-conjugated chitosan nanoparticles enhanced protoporphyrin IX accumulation in colorectal cancer cells. Bioconjugate Chem. 2010;21:679–689.
- Guo J, Schlich M, Cryan JF, et al. Targeted drug delivery via folate receptors for the treatment of brain cancer: can the promise deliver? J Pharm Sci. 2017;106:3413–3420.
- Lee RJ, Low PS. Folate-mediated tumor cell targeting of liposome-entrapped doxorubicin in vitro. Biochim Biophys Acta. 1995;1233:134–144.
- Lee JY, Termsarasab U, Park JH, et al. Dual CD44 and folate receptor-targeted nanoparticles for cancer diagnosis and anticancer drug delivery. J Control Release. 2016;236:38–46.
- Sega EI, Low PS. Tumor detection using folate receptor-targeted imaging agents. Cancer Metastasis Rev. 2008;27:655–664.
- Bhunia SK, Maity AR, Nandi S, et al. Imaging cancer cells expressing the folate receptor with carbon dots produced from folic acid. Chembiochem. 2016;17:614–619.
- Ocak M, Gillman AG, Bresee J, et al. Folate receptor-targeted multimodality imaging of ovarian cancer in a novel syngeneic mouse model. Mol Pharmaceutics. 2015;12:542–553.
- Zwicke GL, Mansoori GA, Jeffery CJ. Utilizing the folate receptor for active targeting of cancer nanotherapeutics. Nano Rev. 2012;3:18496. doi:10.3402/nano.v3i0.18496.
- Warburg O, Wind F, Negelein E. The metabolism of tumors in the body. J Gen Physiol. 1927;8:519–530.
- Warburg O. On the origin of cancer cells. Science. 1956;123:309–314.
- Altenberg B, Greulich KO. Genes of glycolysis are ubiquitously overexpressed in 24 cancer classes. Genomics. 2004;84:1014–1020.
- Seymour GJ, Walsh MD, Lavin MF, et al. Transferrin receptor expression by human bladder transitional cell carcinomas. Urol Res. 1987;15:341–344.
- Walker RA, Day SJ. Transferrin receptor expression in nonmalignant and malignant human breast tissue. J Pathol. 1986;148:217–224.
- Kondo K, Noguchi M, Mukai K, et al. Transferrin receptor expression in adenocarcinoma of the lung as a histopathologic indicator of prognosis. Chest. 1990;97:1367–1371.
- Zhang Y, Xiang J, Liu Y, et al. Constructing transferrin receptor targeted drug delivery system by using doxorubicin hydrochloride and vanadocene dichloride. Bioorg Med Chem Lett. 2011;21:5982–5986.
- Bareford LM, Phelps MA, Foraker AB, et al. Intracellular processing of riboflavin in human breast cancer cells. Mol Pharmaceutics. 2008;5:839–848.
- Johnson T, Ouhtit A, Gaur R, et al. Biochemical characterization of riboflavin carrier protein (RCP) in prostate cancer. Front Biosci. 2009;14:3634–3640.
- Rao PN, Crippin J, Levine E, et al. Elevation of serum riboflavin carrier protein in hepatocellular carcinoma. Hepatol Res. 2006;35:83–87.
- Riboflavin. Monograph. Altern Med Rev. 2008;13:334–340.
- Thomas TP, Choi SK, Li MH, et al. Design of riboflavin-presenting PAMAM dendrimers as a new nanoplatform for cancer-targeted delivery. Bioorg Med Chem. 2010;20:5191–5194.
- Guo D, Shi C, Wang X, et al. Riboflavin-containing telodendrimer nanocarriers for efficient doxorubicin delivery: high loading capacity, increased stability, and improved anticancer efficacy. Biomaterials. 2017;141:161–175.
- Singh S, Mehra NK, Jain NK. Development and characterization of the paclitaxel loaded riboflavin and thiamine conjugated carbon nanotubes for cancer treatment. Pharm Res. 2016;33:1769–1781.
- Huang MY, Shang EX, Tang YP, et al. Research on nourishing and tonifying blood effects of the herb pair consisting of Angelica sinensis and Ligusticum chuanxiong on the basis of drug interaction. Zhongguo Zhong Xi Yi Jie He Za Zhi. 2013;33:516–521.
- Chao WW, Lin BF. Bioactivities of major constituents isolated from Angelica sinensis (Danggui). Chin Med. 2011;6:29
- Amato P, Christophe S, Mellon PL. Estrogenic activity of herbs commonly used as remedies for menopausal symptoms. Menopause. 2002;9:145–150.
- Jayapaul J, Arns S, Lederle W, et al. Riboflavin carrier protein-targeted fluorescent USPIO for the assessment of vascular metabolism in tumors. Biomaterials. 2012;33:8822–8829.
- Jayapaul J, Arns S, Bunker M, et al. In vivo evaluation of riboflavin receptor targeted fluorescent USPIO in mice with prostate cancer xenografts. Nano Res. 2016;9:1319–1333.
- Witte AB, Leistra AN, Wong PT, et al. Atomic force microscopy probing of receptor − nanoparticle interactions for riboflavin receptor targeted gold − dendrimer nanocomposites. J Phys Chem B. 2014;118:2872–2882.
- Sau A, Sanyal S, Bera K, et al. DNA damage and apoptosis induction in cancer cells by chemically engineered thiolated riboflavin gold nanoassembly. ACS Appl Mater Interfaces. 2018;10:4582–4589.
- Beztsinna N, Tsvetkova Y, Bartneck M, et al. Amphiphilic phospholipid-based riboflavin derivatives for tumor targeting nanomedicines. Bioconjugate Chem. 2016;27:2048–2061.
- Chiu SC, Chen SP, Huang SY, et al. Induction of apoptosis coupled to endoplasmic reticulum stress in human prostate cancer cells by n-butylidenephthalide. PLoS One. 2012;7:e33742.
- Liu PY, Sheu JJ, Lin PC, et al. Expression of Nur77 induced by an n-butylidenephthalide derivative promotes apoptosis and inhibits cell growth in oral squamous cell carcinoma. Invest New Drugs. 2012;30:79–89.
- Wei CW, Lin CC, Yu YL, et al. n-Butylidenephthalide induced apoptosis in the A549 human lung adenocarcinoma cell line by coupled down-regulation of AP-2alpha and telomerase activity. Acta Pharmacol Sin. 2009;30:1297–1306.
- Chen YL, Jian MH, Lin CC, et al. The induction of orphan nuclear receptor Nur77 expression by n-butylenephthalide as pharmaceuticals on hepatocellular carcinoma cell therapy. Mol Pharmacol. 2008;74:1046–1058.
- Lin PC, Chen YL, Chiu SC, et al. Orphan nuclear receptor, Nurr-77 was a possible target gene of butylidenephthalide chemotherapy on glioblastoma multiform brain tumor. J Neurochem. 2008;106:1017–1026.
- Siddiqui M, Rajkumar SV. The high cost of cancer drugs and what we can do about it. Mayo Clin Proc. 2012;87:935–943.
- Huang J, Li Y, Orza A, et al. Magnetic nanoparticle facilitated drug delivery for cancer therapy with targeted and image‐guided approaches. Adv Funct Mater. 2016;26:3818–3836.
- Wu C-Y, Wu M-L, Chen Y-C. Selective extraction of n-butylidenephthalide from Angelica sinensis (Danggui) by using functionalized iron oxide magnetic nanoparticles as trapping probes. Anal Methods. 2018;10:1593–1601.
- Chen W-Y, Chen Y-C. Functional Fe3O4@ZnO magnetic nanoparticle-assisted enrichment and enzymatic digestion of phosphoproteins from saliva. Anal Bioanal Chem. 2010;398:2049–2057.
- Chen W-Y, Chen Y-C. MALDI MS analysis of oligonucleotides: desalting by functional magnetite beads using microwave-assisted extraction. Anal Chem. 2007;79:8061–8066.
- Cohen I, Tagliaferri M, Tripathy D. Traditional Chinese medicine in the treatment of breast cancer. Semin Oncol. 2002;29:563–574.
- Gurtu V, Kain SR, Zhang G. Fluorometric and colorimetric detection of caspase activity associated with apoptosis. Anal Biochem. 1997;251:98–102.
- Kominsky DJ, Bickel RJ, Tyler KL. Reovirus-induced apoptosis requires both death receptor- and mitochondrial-mediated caspase-dependent pathways of cell death. Cell Death Differ. 2002;9:926–933.