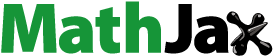
Abstract
Hesperidin, as a flavonone, is recognized as promising anti-inflammatory, antioxidant, and anticancer agent. Its poor bioavailability is crucial bottleneck for therapeutic efficacy. To enhance the stability and bioactive potentials, hesperidin -PLGA-Poloxamer 407 was successfully prepared to minimize or overcome problems associated with hesperidin absorption. The characteristics of nanohesperidin were testing by in vitro dissolution study, XRD, FTIR, PSA and SEM. Antioxidant effects of nanohesperidin were studied. The structure–activity relationship analysis with antioxidant pharmacophore has been performed by using density functional theory method and quantum chemical calculations. The structural properties were investigated using Becke three-parameter hybrid exchange and the Lee–Yang–Parr correction functional methods. Nanohesperidin was found to decrease the H2O2 activity–induced DNA instability. Blood compatibility on human erythrocytes was confirmed by haemolytic and in vitro toxicity assessments. The in vitro anticancer activity of nanohesperidin towards MCF-7 cells using various parameters was carried out. The nanohesperidin was found to exert cell growth arrest, activated DNA fragmentation and induced apoptotic cell death through caspase-3 and p53-dependent pathways. These findings showed that nanohesperidin play an important role in its anticancer effects, suggesting might be used for clinical trials and can represent driving formulation for novel chemotherapeutic agents.
Introduction
Cancer is major health threats in modern life that is annually affecting 12.7 million people worldwide regardless of ethnic or geographic boundaries and without body organ-specificity [Citation1,Citation2]. Breast cancer is the most common invasive cancer in women worldwide. And it comprises 22.9% of women invasive cancers and 16% of all female cancers [Citation3]. Genes that typically regulate processes such as cell growth, division and death as well as DNA repair might undergo mutations, resulting in cancer. This can occur either as accidental “misspell” of genetic code during cell replication or as environmentally-driven DNA damage caused by exposure to chemical, radiation or viral agents [Citation4].
Conventional cancer treatment includes options like surgical excision to remove cancerous parts, radiation therapy, and chemotherapy have their own limitations [Citation5,Citation6]. One of the increasingly attractive strategies to tackle the issue of elevated cancer prevalence worldwide is through prevention, suppression, or reversal of disease progression with chemo-preventive therapies such as dietary, natural or synthetic compounds [Citation7]. During past two decades, a plethora of scientific evidences showed that natural products especially plants and their poly-phenolic compounds have different beneficial effects on human health. Ample evidence from the past 20 years demonstrated various beneficial health effects for natural products, in particular plants and their poly phenolic compounds. Under both in vivo and in vivo experiments, flavonoids showed the most potent anticancer activities among other bioactive compounds [Citation8,Citation9].
Flavonoids are an important class of natural products; particularly, they belong to a class of plant secondary metabolites having a polyphenolic structure, widely found in fruits, vegetables and certain beverages [Citation8]. Flavonoids are composed of several classes including flavonols, flavonones, flavones, iso-flavonoids and antho-cyanidins [Citation10]. Hesperidin is a flavanone glycoside that is found abundantly in citrus fruits, hesperidin was first isolated from citrus peel by the French chemist Lebreton. Hesperidin is a β-7-rutinoside of hesperitin because it consists of an aglycone hesperitin and a disaccharide rutinose. Because of its various biological activities, hesperidin is also called a bioflavonoid [Citation11].
Various in vivo and in vitro reports recognized the potent activities of Hespiridin as an anti-carcinogenic, anti-inflammatory and anti-oxidant agent [Citation9,Citation11]. In parallel, a novel strategy for treating cancer by using antioxidants has recently received remarkable attention. Hesperidin, through the induction of extrinsic and intrinsic mechanisms, has been shown to promote apoptotic cell death of different cancer cells [Citation12,Citation13]. Hesperidin has been shown to induce cancer cell’s apoptosis through multiple chemopreventive mechanisms with involvement of pathways such as β-catenin, Aurora A kinase, PPARy, PI3K/AKT, mTOR NF-kB, caspases and p53 [Citation14–16].
Despite the health benefits produced by hesperidin, the therapeutic outcome is still dependent by the improvement of the pharmacokinetic profile of this compound after oral administration. Hesperidin has limited water solubility, poor bioavailability, and can be easily modified by environmental factors such as temperature, pH and light [Citation17]. A promising approach to overcome the low oral bioavailability of constituents is the development of nanosized drug carriers [Citation18]. Nanotechnology entities can be used to deliver conventional natural products that have poor solubility or a short half-life [Citation19]. Conventional natural products used with entities in nanometer sizes enable us to solve many of problems such as stability, solubility, and toxicity associated with natural products, and also provide a platform for targeted delivery to tumour sites [Citation20]. It has been the area of interest over the last decade for developing precise drug delivery systems as it offers numerous benefits to overcome the limitations of conventional formulations [Citation21,Citation22]. Thus, the present work was designed to synthesize hesperidin-encapsulated PLGA/Poloxamer 407 nanoparticles and evaluate their antioxidant and haemolysis to human red blood cells to explore the mechanisms of its toxicological effects. The cytotoxic potentials in human breast MCF-7 cancer cells were also assessed.
Materials and methods
Cell line, reagents and kits
The human breast (MCF-7) cancer cell line was obtained from Iraqi Center for Cancer and Medical Genetic Research, AL-Mustansiriyah University, Baghdad, Iraq. Standard DNA, D, L-lactide and glycolide, Poloxamer 407, dimethyl sulphoxide (DMSO), and hesperidin were purchased by Sigma-Aldrich (St. Louis, MO, USA). Ascorbic acid, foetal bovine serum, sulphorhadamine101, trypsin-EDTA, 2, 2-diphenyl-1-picrylhydrazyl (DPPH), 3– (4, 5-dimethylthiazal-z-yl)-2, 5-diphenylterazolium (MTT), and Gram's crystal violate stains were purchased from Sigma Chemical Co. (St. Louis, MO). RPMI-1640 medium was purchased from Gibco (USA). The anti-p53 monoclonal antibody (Clone DO-7, Cat.M7001, Dako Cytomation, USA), anti-Caspase 3 monoclonal antibody (Clone MIB-1, Cat.M7240, Dako Cytomation). FITC-conjugated Alexa flour 488 (F(ab’) fragment of goat anti-mouse IgG (H + L) Molecular Probe, USA). Antibiotics such as penicillin and streptomycin were added to RPMI-1640 medium to prevent microbial contamination (Biosource International, Nivelles, Belgium). All other chemicals and reagents were used at analytical grade level.
Preparation of hesperidin-PLGA/Poloxamer 407 nanoparticles
The modified hesperidin nanoparticles were optimized and developed by the nano participation technique described by Sulaiman et al. [Citation23] with some modifications. Briefly, 20 mg of native hesperidin were dissolved in 1.25 mL of dimethyl sulphoxide (DMSO) and sonicated for 20 min, Poly (D,L-lactic-co-glycolic acid) (PLGA) 50 mg were dissolved in 1.25 mL of DMSO and then mixed with the solution of hesperidin after sonication. After that, the solution was stirred at 1000 rpm for 30 min in room temperature to obtain a homogeneous solution. Poloxamer 407 (50 mg), used as a stabilizer, was dissolved in 5 mL of deionized distilled water. The organic phase contains the active constituent and PLGA was then added to the dispersing phase by means of a syringe positioned with the needle directly in the medium under moderate magnetic stirring. After this step, the emulsion was transferred to 20 mL deionized distilled water to facilitate diffusion and was stirred overnight at room temperature. Then, the solution was centrifuged at 14,000 rpm for 15 min to separate free active compounds and any unbound stabilizer in the solution. The amount of non-entrapped hesperidin in aqueous phase was determined using Hitachi U-2910 Spectrophotometer (Tokyo, Japan) at 283 nm. The percentage of hesperidin encapsulated on the nanoparticles was calculated using the following equations:
After this step, the pellet was re-dispersed in 20 mL of deionized distilled water for further assessments. The crystalline state of the samples was estimated with an X-ray diffractometer (XRD-6000, Shimadzu, Japan). The diffraction pattern was obtained using a Cu Kα incident beam (λ = 1.542 A°) at 2θ = 0°–65°. The voltage and current of X-ray tubes were 45 kV and 30 mA, respectively. FTIR analysis was carried out with an FTIR spectrometer (8400S, Shimadzu, Japan) in attenuated total reflection mode and spectral range of 4000–400 cm−1 with a resolution of 4 cm−1. Particle size was determined by photon correlation spectroscopy (PCS) by using a Zetasizer 5000 (Malvern Instruments Ltd., UK). Finally, the morphology and the uniformity of hesperidin nanoparticles were confirmed via scanning electron microscopy assay (Shimadzu AA-7000, Japan).
Antioxidant activities
The antioxidant activities of hesperidin nanoparticles were investigated using the DPPH assay according to the method introduced by Sulaiman et al. [Citation24]. Briefly, 0.5 mL of different concentrations of hesperidin or hesperidin nanoparticles (40, 80, 120, 160 and 200 µg mL−1) and 0.5 mL of DPPH was added to all tubes and incubated at room temperature for 30 min. The DPPH with absolute ethanol was used as negative control while, ascorbic acid (Vitamin C; 5 µg mL−1) was used as positive control. The absorbance was read at a wavelength of 517 nm in a UV/VIS spectrophotometer (Lambda 19, PerkinElmer, USA). The absorbance of the control (DPPH solution) was also read. The percentage inhibition of the sample was calculated using the following formula:
where Ac and As are the absorbance values for DPPH and test sample solvent, respectively.
Computational study
Gaussian 09 Revision A(0).02 (Pittsburgh, PA) was used to calculate the ground-state geometry of hesperidin and was fully optimized with hybrid density functional theory (DFT) by using basis set 6–31 G. The Becke three-parameter hybrid exchange functional in combination with the Lee–Yang–Parr correction functional was used to optimize the geometries functions. The highest occupied molecular orbital (HOMO) distribution and lowest unoccupied molecular orbital (LUMO) distribution of hesperidin radical molecules were also discussed in present study [Citation25].
Spectrophotometric analysis of DNA damage
The experimental part of this assay was done with standard vertebrate macromolecular DNA in same protocol of our previously study introduced by Sulaiman et al. [Citation23]. In brief, DNA sample that have absorbance ratio about A260/A280 was treated with different concentrations of native hesperidin or modified nanohesperidin (10, 20, 30, 40, 50) µg mL−1, equal volumes of either native hesperidin or modified nanohesperidin with standard DNA solution were mixed in presence of 1 × 10−5 M of hydrogen peroxide (H2O2) solution and then incubated at 37 °C for 10 min. After the incubation period, the absorbance was read at a wavelength of 260 nm with a UV/VIS spectrophotometer. In present assay, ascorbic acid (Vitamin C; 5 µg mL−1) was used as positive control.
Haemolysis assay of human blood samples
The haemolysis assay of human blood samples was performed on the prepared hesperidin nanoparticles following the previously reported of our protocol with some modifications [Citation23]. Briefly, a 200 µL sample of whole human blood was added to 1600 µL of phosphate-buffered saline (PBS). Then, 200 µL of either native hesperidin or hesperidin nanoparticles at five concentrations 20, 40, 80, 160 and 320 µg mL−1, were added to this diluted blood along with the deionized distilled water (positive control; 100% haemolysis) and PBS (negative controls; 0% haemolysis). All tested samples were prepared in triplicates, and the suspension was incubated at 37 °C for 1 h. Finally, the mixtures were centrifuged at 700 rpm for 5 min. The absorbance was measured at 541 nm by UV–Vis spectrophotometer, and the percentage of haemolysis was calculated using the following formula:
Percentage haemolysis = OD sample − OD (– ve) control/OD (+ ve) control − OD (− ve) control
After that, the samples were subjected for further investigations such as light microscopy and fluorescent microscopy analyses.
Clonogenicity and proliferation of MCF-7 cells
The MCF-7 cells were cultured in RPMI-1640 medium supplemented with 10% FBS, 2 mM L-glutamine, and 20 mM HEPES under a humidified atmosphere of 5% CO2 at 37 °C in tissue culture flasks (T 25 cm2; Falcon, USA). For colony formation assay, the cells were seeded at a density of 1000 cells mL−1 in flat-bottom culture plates (Thermo Scientific, USA) and incubated for 48 h. Then, cells were treated with pure hesperidin or modified nanohesperidin (at concentrations 5, 10, 20 and 40 µg mL−1) of each cell line in three independent experiments. When the non-treated cells were reached to a confluent monolayer, the medium was discarded, and the wells were washed with 2 mL of PBS solution. Then, the colonies in each well were stained with 200 µL of crystal violet stain for 2 min. After removing the stain, extensive washing was performed in tap water for 10 min. Then, the plates were tapped on paper towel, dried, and photographed. For more confirmation, the growth inhibition of MCF-7 cells induced by modified nanohesperidin was investigated in vitro. Briefly, 200 µL of MCF-7 cells were seeded in 24-well flat-bottom culture plates (Falcon, USA) at a density of 1 × 106 cells mL−1. After 48 h in the exponentially growing phase, the cells were treated with modified nanohesperidin at concentrations of 5, 10, and 15 µg mL−1 for 24 h. After incubation, 50 µL of the labeling stain consisting of crystal violet in PBS solution was added to each well. Incubation was continued for 10–15 min at 37 °C, and the stain was discarded and washed with tap water. Then, the plates were dried, and photographed using inverted microscope [Citation23].
Detection apoptosis by sulphorhodamine 101 stain
Sulphorhodamine 101 assay (SR 101) was measured to observe the morphological changes of MCF-7 cells by fluorescence microscopy. The cells were seeded at a density of 5 × 103 in six multiwell plates containing sterile coverslip on the bottom of each well and allowed to adhere for 48 h in a humidified atmosphere supplemented with 5% CO2 and 95% air at 37 °C. Then MCF-7 cells were treated with three concentrations (20, 40 and 60 µg mL−1) of modified nanohesperidin for 24 h. After that, the medium was discarded by aspiration, and cells were washed with filtered PBS. The PBS was removed, and cells were fixed with 96% ethanol for 30–60 min. Then, the fixative was removed and distilled water was added for an additional washing. Then the cells were stained with SR 101 solution for 30–60 min. After that, the stain was removed, and the coverslips were washed with distilled water, air dried, and applied on a slide. The morphological changes of cells were inspected under 40× power of a fluorescence microscope [Citation23].
Expression of P53 and caspase-3 assays
For P53 assay, the MCF-7 cells were exposed to 10 and 20 µg mL-1 concentrations of both hesperidin and hesperidin nanoparticles for 24 h, while, control cells were treated with medium only. After that, the media was removed and washed the cells with PBS, then the PBS was discarded and fixed the cells in 1 mL of fixative drop-wise with a continued mixing and brooded in ice bath about 30 min, and further incubation at 4 °C was carried out for overnight. After that, the cells were centrifuged 10 min at 1200 rpm to collect pellet. The fixed cells were suspended in washing buffer (1 mL) and collected by centrifugation about 10 min at 1200 rpm and permeabilized with permeabilization solution about 15 min at ambient temperature. After that, the cells were washed with 1 mL of washing buffer and collected the cells by centrifuge about 10 min at 1200 rpm and incubated with 100–200 μL of anti-p53 monoclonal antibody about 60 min away from the light at ambient temperature. After removing the free anti-p53 by washing the cells with permeabilization solution about 15 min at ambient temperature, the cells were incubated with 200 μL of FITC-conjugated Alexa flour 488 F(ab')2 pieces anti-mouse IgG about 60 min away from the light at ambient temperature. Finally, the cells were examined with fluorescent microscope instrument. For caspase-3, the assay was accomplished in accordance with the same procedure and conditions used in gene expression of p53 but by replacing the anti-p53 monoclonal antibody with anti- Caspase-3 monoclonal antibody.
Single-cell gel electrophoresis
The genotoxicity of with modified nanohesperidin was determined using single cell gel electrophoresis (alkaline comet assay). This assessment based on detect of DNA damage as strand breaks under electrophoresis current as technique has been adapted from that originally proposed by Benhusein et al. [Citation26]. MCF-7 cells were treated with modified nanohesperidin at concentrations of 1.0, 5.0, and 10.0 µg mL−1 for 24 h. Then, the cells were lysed by lysis solution, electrophoresed and the comet images were captured under fluorescence microscope.
Gel electrophoresis DNA-binding assay
Analysis of DNA fragments using agarose gel electrophoresis was performed according to Magnesia tissue culture cell DNA extraction Kit as manufacturer indicated. MCF-7 cells were seeded in suitable conditions and followed the same protocol introduced by Sulaiman et al. [Citation23]. This protocol provides a qualitative method for assessing cell death by detecting DNA fragmentation. In brief, the growing cells were treated with three concentrations (10, 20, 30 and 40 µg mL−1) of modified nanohesperidin for 24 h. Cells at density 1.5 × 106 cell mL−1 were then harvested and suspended in ice-cold PBS. The cell suspension was centrifuged (1200 rpm) at 4 °C for 10 min. The supernatant was removed and the cells was frozen (−20 °C) overnight. The DNA was dissolved with DNA loading buffer, and then applied to 0.8% agarose gel electrophoresis. After staining with ethidium bromide, the DNA was visualized by UV irradiation and photographed by gel documentation system (Sci-Plus, UK). Loading marker was loaded in line parallelled to that of the samples.
Statistical analysis
Data analysis was performed using SPSS statistical program (Version/18.0; SPSS Inc., Chicago, IL). Analysis of variance (ANOVA) was used to determine whether there are any statistically significant differences between the means of three independent experiments. A p values of <.05 was considered statistically significant.
Results and discussions
Synthesis and encapsulation efficiency
In the present investigation, hesperidin nanoparticles were synthesized by the nanoprecipitation technique using PLGA and Poloxamer 407 polymers. The photographs of pure hesperidin and nanohesperidin powders and their solubility in water were shown in and , respectively. The prepared nanohesperidin powder was a very fine dispersion and lightest when compared with pure hesperidin as presented in . When hesperidin nanoparticles were re-suspended in water, it was more soluble, unlike a native hesperidin, which was completely insoluble in water, with un-dissolved particles clearly visible in the solution. After 24 h at room temperature, the nanohesperidin was remained soluble in water, while a native hesperidin was completely precipitated (). Hesperidin nanoparticles were more stable at 4 °C and better than that at 37 °C. Thus, the nanoparticles were stored at 4 °C and used within 24 h of preparation for present experiments. The enhanced aqueous solubility of nanoparticles could be attributed to their larger surface area, which promotes dissolution. However, the reduction in the particle size of active ingredients to nanoparticle size has shown to improve the solubility, and bioavailability [Citation27]. Noyes and Whitney first formulated an equation to relate the dissolution rate of compounds, and, over the past decade, this equation has been used to explain the dissolution rate of hydrophobic compounds in numerous studies [Citation28]. The equation indicates that reducing particle size is a well-known and efficient method to enhance the dissolution percentage of hydrophobic compounds [Citation29]. Sustained release of the drug from nanoparticles is an important parameter for developing successful formulations [Citation30].
Figure 1. Photograph showing of (A) pure hesperidin and (B) modified nanohesperidin loaded in PLGA- Polixamar 407 powder.
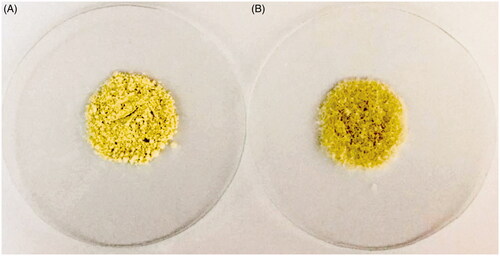
Figure 2. Photograph showing the solubility of (A) pure hesperidin and (B) modified nanohesperidin loaded in PLGA- Polixamar 407 in water after 5 min, 12 h and 24 h of preparation, respectively.
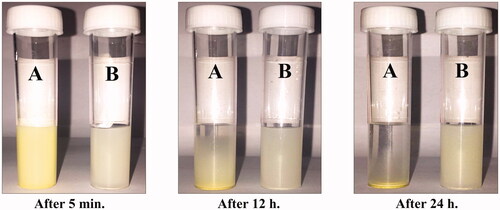
The in vitro release profile of hesperidin nanoparticles from PLGA are given in . The native hesperidin showed slow release of about only 3.5% within 72 h. While, hesperidin nanoparticles showed biphasic pattern of drug release from PLGA, This meaning there was an initial rapid release of about 35% of drug in the first 8 h, and followed by a sustained and slow release behavior of about 59% of drug in the next 72 h. The encapsulation efficiency and drug loading of hesperidin nanoparticles were 96.4% and 11%, respectively. The initial rapid release is due to the free hesperidin attached to the surface of the nanoparticles, and the sustained release is from the encapsulated drug [Citation31]. This biphasic release behaviour of hesperidin nanoparticles from PLGA was observed is similar to previous research [Citation32]. The increase in dissolution velocity may be attributed to its smaller particle size and increased surface area.
X-ray diffraction spectrometry assay
represents the XRD patterns of both hesperidin and hesperidin nanoparticles. Pure hesperidin has displayed the strong characteristic peaks of 2ϴ of 7.8°, 11.4°, 15.5°, 17.1°, 19.5°, 22.2°, 23.2°, 25.5° and 29.1°, indicating its high crystalline structure. Few patterns were detected in modified hesperidin nanoparticle which showed less peaks with different peak intensities. These findings provide evidence that hesperidin nanoparticle were either molecularly dispersed or in amorphous state in the matrix. XRD results confirmed those hesperidin nanoparticles which were formed were may lost its crystalline structure during preparation processes. Similar observation was made by Gao et al. [Citation33] who reported that hesperidin completely dispersed in PEG6000 and there were some interaction between hesperidin and PEG 6000. The full widths at half maximum (FWHM) values of the peaks of the nanohesperidin were higher than values that observed in native hesperidin (data not presented). According to the Scherrers equation, the larger FWHM values suggest smaller particle size [Citation23]. These changes in the physical state of pure hesperidin as a result of particle size reduction which has significant consequences on the solubility and bioavailability of poorly soluble drugs [Citation34,Citation35].
Fourier-transform infrared spectroscopy assay
The FTIR spectra of native hesperidin and nanohesperidin are presented in . The absorption band at 3421.83 cm−1 corresponding to hydroxyl group O–H stretching vibration. The band at 2945.40 cm−1 is due to alkane C–H stretching vibration. The FTIR spectrum peak of carbonyl C=O stretch appeared at 1761.04 and 1641.48 cm−1. The bands at 1516.10–1388.76 cm−1 are attributed to aromatic C=C stretch, and the aromatic C–O stretch at 1273.06, 1176.62, 1126.47 and 1089.82 cm−1. The FTIR spectra of native hesperidin showed characteristic bands because of existence of different functional groups like, 3471.91, 2928.04, 1645.33, 1516.10, 1064.74 cm−1 which could be attributed to O–H stretching vibration, C–H stretching, C=O stretching, C=C stretching and C–O stretching, respectively. Both spectra of hesperidin and nanohesperidin have the same characteristic peaks but with some difference due to the minor chemical interaction of native hesperidin and PLGA matrix. PLGA characteristic band of the carbonyl group at 1761.04 cm−1 confirmed the successful conjugation and peak intensities in hesperidin/PLGA nanoparticles. Consequently, we confirmed that the characteristic of hesperidin did not influence when it was loaded to PLGA. These results agreed with Cho et al. [Citation36] who confirmed that characteristics of final formulation of hesperidin did not change when it was added to PLGA, indicating that modified nanohesperidin can be incorporated in the PLGA without altering its individual structural identity.
Scanning electron microscopy assay
Particle morphology, size and shape of native hesperidin and hesperidin nanoparticles were evaluated by using SEM as shown in . , native hesperidin particles revealed no uniformity and the particles appear to be aggregated and have irregular arrangement, when compared with hesperidin nanoparticles (). Hesperidin nanoparticles showed well-separated and smooth particles with spherical shape with diameters ranging between 25 and 100 nm. All particles were almost distributed as single particles and clearly homogeneous. No agglomerated or aggregated particles were detected. This can be attributed to Poloxamer which adsorb strongly onto the surface of hydrophobic nanospheres [e.g. polystyrene, poly (methyl methacrylate), poly (phosphazene), poly (butyl 2-cyanoacrylate and poly (lactide-co-glycolide)) nanospheres] via their hydrophobic POP “polyoxypropylene” center block. This mode of adsorption leaves the hydrophilic POE “polyoxyethylene” side-arms in a mobile state because they extend outwards from the particle surface. These side-arms provide stability to the particle suspension by a repulsion effect through a steric mechanism of stabilization, involving both entropic and enthalpic contribution [Citation37].
Particle size analysis
shows the particle size distribution of hesperidin and nanohesperidin. From the dynamic light scattering results, the calculated average particle size distribution of formulated hesperidin nanoparticles was found to be around 55.5 nm. In contrast with native hesperidin, had a broad size distribution with an average particle size around 1320.9 nm. Interestingly, these nanoparticles have not displayed any adhesion or agglomeration. Likewise, decreasing of particle size will improve dissolution velocity. Increasing dissolution velocity can conduct to improve bioavailability especially to biopharmaceutical class system (BCS) II which dissolution velocity is the rate limiting step [Citation38].
Antioxidant activity
As shown in , the antioxidant potentials of hesperidin and hesperidin nanoparticles were monitored using five different concentrations. The results demonstrated a higher free radical scavenging activity of modified nanohesperidin than native hesperidin, and a concentration-dependent inhibition was observed. The results showed that native hesperidin significantly reduced the level of the DPPH• in a concentration dependent manner and 200 µgmL−1 was significantly better than the other concentrations which show 38.2% antioxidant activity while, positive control was 88.1%. In comparison with nanohesperidin activity, the nanohesperidin has a higher capacity of reducing the DPPH• radical than native hesperidin which show 62.7% antioxidant activity. The enhanced antioxidant activity of nanohesperidin could be attributed to the enhanced solubility and dissolution rate. Importantly, antioxidants can donate electrons to reactive radicals, converting them into more stable and unreactive species [Citation39]. For instance, flavonoids’ activity including hesperidin as antioxidants, refer to their ability to transfer a hydrogen atom or an electron and the possibility of their interactions with other antioxidants [Citation40]. In order to determine the relationship between hesperidin’s structure and antioxidant activities, the proposed reaction mechanisms based on the experiment of DPPH radicals and the molecule of hesperidin was calculated by using a model system. Four resonance limit structures were visualized in scheme of hesperidin as shown in . The antioxidant properties of hesperidin result from their chemical structure due to the presence of 3 and 5 hydroxylation. In fact, the hydroxyl radical scavenging activity of hesperidin can be attributed to the presence of hydroxyl group in association with the double bond C2–C3 on B-ring, which donates hydrogen and an electron to hydroxyl radicals, and making them stable and giving rise to a relatively stable flavonoid radical [Citation41]. Thus, the hydroxyl group attached to the C3 can easily undergo one- electron oxidation to stabilize hydroxyl radical (OH·) which allow electron delocalization in position 2 and in B ring. Also, the 4-carbonyl group of the C ring, and configuration of the 5-hydroxyl group and the double bond C5–C6 of the A ring. All the mentioned structural conditions may be found in a hesperidin molecule which, in the in vitro systems efficiently scavenges hydroxyl radical (OH•), superoxide anion radical (O2 •−), and nitrogen oxide (NO•) [Citation40].
HOMO and LUMO distribution of hesperidin
In order to obtain more electronic molecular insight into the antioxidant activity of hesperidin, the HOMO and the LUMO states were accomplished by using Density Function Theory (DFT) based quantum chemical descriptors as presented in . The HOMO energy demonstrated that charge transfer take place within the molecule in accordance with the capacity of the molecule to donate electrons, while the LUMO energy take place in accordance with the capacity of the molecule to accept electrons. The calculated frontier orbital distributions and energies of hesperidin and the electron volt values. According to the energy plots, the present experiment suggested that the antioxidant potential of hesperidin was verified by the high number of hydroxyl groups in the molecule and by the alleviation of formation of phenoxyl radicals generated from these groups. However, the LUMO energy plots clearly indicated that high electron density contributed between B and C rings and between C-2 and C-3, which clearly assured the stability of free radicals between rings. Hence, the study of the molecular and electronic properties is significant for understanding the mechanical functioning of these compounds as antioxidants [Citation42].
Protective activity assay against DNA damage
represents A260 histogram of DNA damage induced by H2O2 and effect of five concentrations (10, 20, 30, 40, and 50 µg mL−1) of hesperidin and nanohesperidin in reduction of this damage. From these data it was observed that hesperidin possessed significant hydrogen peroxide scavenging activity and this activity increased with concentration. However, scavenging activity of hesperidin nanoparticles was higher than native hesperidin and the effect was also a concentration dependent manner. The protective effect of hesperidin on DNA can be explained by its ability to scavenge ROS due to its property as an antioxidant. Previous study showed that hesperidin had chemopreventive activities and also anticancer effects. The authors proposed that the chemopreventive effects of hesperidin were related to its antioxidant activity. Also, it is reported that hesperidin was non-toxic against normal cells, but it is reported that hesperidin suppresses cell proliferation in several cancer types [Citation15,Citation43].
Haemolytic activity against red blood cells
The photographs of RBCs after exposure to five concentrations of hesperidin and modified nanohesperidin (20, 40, 80, 160 and 320 µg mL−1) for 1 h at 37 °C are illustrated in . Apparently, the percentage of the haemolysis of hesperidin was higher than that of nanohesperidin. The haemolysis data of nanohesperidin at the concentration 20 and 40 µg mL−1 were within the acceptable level of less than 4%; while, the 40 and 80 µg mL−1 caused less than 5% haemolysis (; lower lane) as compared with the corresponding concentrations of native hesperidin (; upper lane), only 20 µg mL−1 was within acceptable level (less than 5%). According to the criterion in the ASTM E2524-08 standard, percent haemolysis of more than 5% indicates that the test nanoparticle damaged the RBCs [Citation44]. According to ASTM standard, hesperidin and hesperidin nanoparticles are haemocompatible and can be used safely inside the body only with low concentrations. Light microscope image of RBCs represent in and shows impact of hesperidin and hesperidin nanoparticles at lower and higher concentration in similar way to the results in . The haemolysis assay showed no deleterious activity on the RBCs morphology over the studied lower concentration range especially at modified nanohesperidin. While native hesperidin showed more defective effects against RBCs which causes cell membrane destruction. Similar blood compatibility activates were observed when used nanochrysin at low concentrations [Citation23]. However, the results of current study illustrating the excellent blood compatibility of the prepared nanoparticles at low concentrations as well as it is potential used in different applications, particularly in toxicity applications.
Colony forming activity and cytotoxicity assay
The result of clonogenic survival assay is presented in . Consistent with the proliferation assays, The effect of hesperidin nanoparticles (; lower lane) reduced significantly the number of colonies of MCF-7 cells and was more potent than the effect of native hesperidin (; upper lane). The higher cytotoxic action of hesperidin nanoparticles could be due to slow cellular uptake and intracellular distribution of hesperidin nanoparticles because of its slow release behavior. Flavanones such as hesperidin and hesperitin relying on the cancer cell line and exposure conditions can stimulate apoptosis, DNA damage, diminish the invasive potential and reduce NF-κB activity [Citation45]. A study on the mechanism by which hesperidin nanoparticles induces cell death is needed and will be extended for further investigations. The cytotoxicity effect of hesperidin nanoparticles on MCF-7 cells was also confirmed by using a crystal violet assay as represented in . As mentioned above the hesperidin nanoparticles exert strong cytotoxicity against MCF-7 cells and this effect was concentration dependent. The hesperidin nanoparticles induced numerous morphological alterations including cell shape changes, treated cells appeared to be clustered with a few cellular extensions and inhibition of cell communication . These effects were increased at higher concentration (), no such alterations were seen in non-treated cells (). Nanoparticles due to their smaller size have the capacity to penetrate the cells more effectively than micro-particles, which simplify administration of large amounts of drug to gain better performance [Citation23]. Other studies showed that hesperidin has considerable cytotoxic effect against chosen human cancer cell lines including cervix, liver, larynx, and breast. Furthermore, studies revealed that hesperidin possessed chemopreventive potential, an apoptotic effect in human colon cancer cells and inhibit colon carcinogenesis in rats [Citation13,Citation46].
Morphological study using SR 101 assay
Morphological changes of MCF-7 cells were examined by using SR 101 staining method to evaluate if the cytotoxic effect of hesperidin nanoparticles was due to apoptosis or necrosis. Fluorescence microscopic images of MCF-7 cells after treating with different concentrations of hesperidin nanoparticles are shown in . The apoptosis features such as nuclear fragmentation, changes in size and shape of the cells, in addition to decrease the cells population. All these features were observed in the cells that treated with hesperidin nanoparticles and 60 µg mL−1 was more potent in this regard. While, the control cells showed a normal nuclear morphology with normal shape and size, these results indicate that the mechanism of cell death may be due to the apoptosis. Apoptosis is a dominant mode of programmed cell death and is a main therapeutic mechanism in radiotherapy and chemotherapy [Citation47]. Hesperidin has been reported to stimulate apoptotic cell death in diverse cancer cells by stimulating both intrinsic and extrinsic pathways. Estimation of apoptotic efficacy of hesperidin in human mammary carcinoma cell line (MCF-7) demonstrated that hesperidin at the high concentrations significantly provoked the cell detachment, shrinking of cells, formation of plasma membrane blebs and vacuolation [Citation9].
Expression of p53 and caspase-3 in MCF-7 cells
This method was performed to evaluate whether hesperidin nanoparticles induce apoptosis due to enhance the p53 and caspase-3 pathways in MCF-7 cell line and the results were illustrated in . For the p53, the hesperidin nanoparticles caused an increased expression of p53 during the 24 h of treatment. The treated cells with 10 µg mL−1, a slight increased expression of p53 was recorded, but after treatment with 20 µg mL−1 the expression was higher than that observed in previous concentration. With respect to caspase -3, the expression was also increased in the same picture observed in p53. However, the two proteins were significantly higher than the expression of non-treated cells. The p53 play a significant role in apoptosis through the mitochondrial mediated apoptotic pathway by stimulating caspase-8 activity, leading to the release of apoptogenic factors like cytochrome-C (Cyt-c). It is reported that after the release of cytochrome-C from mitochondria, this triggers the activation of caspase-3 and the consequent rapid split of PARP, which is a substrate of caspase-3 [Citation48]. Therefore, accumulation of p53 and caspase-3 proteins in MCF-7 cells indicated that hesperidin nanoparticles may enhance the susceptibility of MCF-7 cells to trigger apoptosis by stimulating the singling pathways of tumor suppressor protein and caspase-3. Another studies elucidated the cytotoxicity of hesperidin on other types of cancer cell lines, hesperidin seems to have cytotoxicity on colon cancer SNU-C4 cells in dose-dependent manner and induce specific intracellular death-related pathways, lead to caspase-3 activation and stimulating apoptosis. Furthermore, hesperidin induced p53 expression at the mRNA level in NALM-6 cells after being exposed to various concentrations of hesperidin for 24 h [Citation14,Citation49].
Alkaline comet assay
Alkaline comet assay was accomplished to investigate whether the hesperidin nanoparticles can induce DNA damage in MCF-7 cells. represents different DNA damage categories. Treatment of MCF-7 cells with hesperidin nanoparticles for 24 h revealed induction of DNA single strand breaks as compared with non-treated cells and the effect was a dose-dependent cytotoxicity. Depending upon the seriousness of DNA damage and tail length in MCF-7 cells, the results were classified to four categories or classes and damage frequency at the three concentrations tested was greater than non-treated cells. In treated cells with hesperidin nanopartilces, the length of the comet tail and the percentage of DNA damage (genotoxicity) were increased, thereby indicating that level of the DNA damage increased in a dose-dependent manner.
Figure 18. Comet assay for MCF-7 cells treated with modified nanohesperidin loaded in PLGA- Polixamar 407. Class 0: intact cell with normal nucleus, Class 1: halo around the nucleus, Class 2 and Class 3: Gradual increases in the length of comet tail indicate more damage with a decrease in nuclear DNA content.
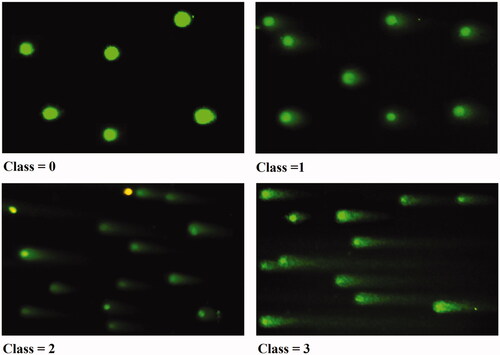
In control cells, Class 0 was recorded and cells were intact and round nuclei without any damaged DNA. Class 1 showed halo around nuclei which represents low DNA damage, followed by class 2 and class 3 which showed the gradual increase in the tail length of well-formed comet and increase in DNA migration from nucleus. Since, DNA migration can also resulting from cytotoxicity, apoptosis or necrosis, these data indicate that hesperidin nanoparticles induced DNA fragmentation and has genotoxicity potential in DNA of cancer cells. There are several mechanisms offered for the cytotoxicity of flavonoids including the inhibition of topoisomerases, kinases and their pro-oxidant action. Unexpected genotoxic behavior of these compounds could explain, in part, by their pro-oxidant activity [Citation50]. However, the hesperidin genotoxicity recorded in present study can be explained as a result of the production of reactive oxygen species by redox cycling. Phenolic compounds give rise to the superoxide anion by auto-oxidation, which in turn may lead to the formation of H2O2 [Citation51]. The H2O2 can kill any living cells and abort their development at any stage and results in DNA damage along with protein and lipid components, which may lead to cell death by the hydroxyl radical generated via Fenton reaction. When nanoparticles possess a large surface area to mass ratio, this enabled it to be highly reactive. Consequently, it is possible that nanoparticles may adsorb free radicals on their surface and also releasing free radicals [Citation52].
Gel electrophoresis DNA-binding assay
To further evaluate the ability of nanohesperidin induced DNA damage, the agarose gel electrophoresis assay was performed in MCF-7 cells. represents a DNA fragmentation in MCF-7 cells after treatment with 10, 20, 30 and 40 µg mL−1 concentrations of modified nanohesperidin and exhibited an apoptotic DNA smears and the effect was dose dependent. However, DNA fragmentation ladders were not observed in controls. A proposed mechanism of apoptosis induced by hesperidin nanoparticles induced DNA fragmentation is may through by activation of free radicals of ROS. When the DNA exposed to ROS, this lead to production of a compound called 8-hydroxy-2′-deoxyguanosine (8-OHdG), the presence of this compound alters the guanosine base and increasing the chance of G to T mutation during DNA replication. 8-OHdG considers as a marker of DNA damage, a site of increased hazard for mutagenicity and a biomarker of oxidative stress [Citation53]. Induction of apoptosis has been considered to be the major mechanism of anticancer drug discovery, and the development of anti-cancer drugs that inhibit abnormal cancer cell proliferation and induce cell death through apoptosis is a fundamental objective of cancer research [Citation22,Citation54].
Conclusion
The present results revealed that the modified nanohesperidin was cytocompatible and more potent as compared with the native hesperidin. It exhibited anti-oxidant activity and protective effect towards DNA damage under of H2O2 activity. Moreover, the modified nanohesperidin revealed higher inhibitory activity on the breast cancer cells by inducing cell death through apoptotic pathway of p53 and Caspase-3. From the present investigation, it is concluded that the nanohesperidin fabrication using nanoprecipitation method, was more successful and can be used in delivery systems for bioactive compounds which will be worth trying to study for therapeutic efficiency, including cancers. However, the anti-tumor activities of hesperidin nanoparticles on breast cancer in vivo and the underlying molecular mechanisms require further investigation, but hesperidin nanoparticles has the potential to be developed as a chemotherapeutic agent for human breast cancer.
Disclosure statement
No potential conflict of interest was reported by the authors.
References
- Bharali DJ, Mousa SA. Emerging nanomedicines for early cancer detection and improved treatment: current perspective and future promise. Pharmacol Ther. 2010;128:324–335.
- Sutradhar KB, Amin L. Nanotechnology in cancer drug delivery and selective targeting. ISRN Nanotechnol. 2014;2014:1–12.
- Abdollahi A, Ali-Bakhshi A, Farahani Z. Concentration study of high sensitive c - reactive protein and some serum trace elements in patients with benign and malignant breast tumor. Int J Hematol-Oncol Stem Cell Res. 2015;9:180–184.
- Broustas CG, Lieberman HB. DNA damage response genes and the development of cancer metastasis. Radiat Res. 2014;181:111–130.
- Singh OP, Nehru RM. Nanotechnology and cancer treatment. Asian J Exp Sci. 2008;22:45–50.
- Chidambaram M, Manavalan R, Kathiresan K. Nanotherapeutics to overcome conventional cancer chemotherapy limitations. J Pharm Pharm Sci. 2011;14:67–77.
- Batra P, Sharma AK. Anti-cancer potential of flavonoids: recent trends and future perspectives. 3 Biotechnology. 2013;3:439–459.
- Del Rio D, Rodriguez-Mateos A, Spencer JP, et al. Dietary (Poly) phenolics in human health: structures, bioavailability, and evidence of protective effects against chronic diseases. Antioxidants Redox Signal. 2013;18:1818–1892.
- Devi KP, Rajavel T, Nabavi SF, et al. Hesperidin: a promising anticancer agent from nature. Indust Crops Prod. 2015;76:582–589.
- Panche AN, Diwan AD, Chandra SR. Flavonoids: an overview. J Nutr Sci. 2016;5:e47.
- Li C, Schluesener H. Health-promoting effects of the citrus flavanone hesperidin. Crit Rev Food Sci Nutr. 2017;57:613–631.
- Banjerdpongchai R, Wudtiwai B, Khaw-on P, et al. Hesperidin from citrus seed induces human hepatocellular carcinoma hepg2 cell apoptosis via both mitochondrial and death receptor pathways. Tumor Biol. 2016;37:227–237.
- Wang Y, Yu H, Zhang J, et al. Hesperidin inhibits HeLa cell proliferation through apoptosis mediated by endoplasmic reticulum stress pathways and cell cycle arrest. BMC Cancer. 2015;15:682.
- Ghorbani A, Nazari M, Jeddi-Tehrani M, et al. The citrus flavonoid hesperidin induces p53 and inhibits NF-kB activation in order to trigger apoptosis in NALM-6 cells: involvement of PPARγ - dependent mechanism. Eur J Nutr. 2012;51:39–46.
- Lee KA, Lee SH, Lee YJ, et al. Hesperidin induces apoptosis by inhibiting Sp1 and its regulatory protein in MSTO-211H cells. Biomol Ther. 2012;20:273–279.
- Saiprasad G, Chitra P, Manikandan R, et al. Hesperidin induces apoptosis and triggers autophagic markers through inhibition of Aurora-A mediated phosphoinositide-3-Kinase/Akt/Mammalian target of rapamycin and glycogen synthase kinase-3 beta signalling cascades in experimental colon carcinogenesis. Eur J Cancer. 2014;50:2489–2507.
- Majumdar S, Srirangam R. Solubility, stability, physicochemical characteristics and in vitro ocular tissue permeability of hesperidin: a natural bioflavonoid. Pharm Res. 2009;26:1217–1225.
- Loganathan N, Sellappan M. Designing of nanosized bioflavonoids using biodegradable polymeric nanoparticles by plackett burman method. Int Curr Pharm J. 2017;6:9–15.
- Bilia AR, Isacchi B, Righeschi C, et al. Flavonoids loaded in nanocarriers: an opportunity to increase oral bioavailability and bioefficacy. Fns. 2014;05:1212–1227.
- Bharali DJ, Siddiqui IA, Adhami VM, et al. Nanoparticle delivery of natural products in the prevention and treatment of cancers: current status and future prospects. Cancer. 2011;3:4024–4045.
- Malam Y, Loizidou M, Seifalian AM. Liposomes and nanoparticles: nanosized vehicles for drug delivery in cancer. Trends Pharmacol Sci. 2009;30:592–599.
- Sutradhar KB, Amin ML. Nanoemulsions: increasing possibilities in drug delivery. Eur J Nanomed. 2013;5:97–110.
- Sulaiman GM, Jabir MS, Hameed AH. Nanoscale modification of chrysin for improved of therapeutic efficiency and cytotoxicity. Artif Cells, Nanomed Biotechnol. 2018;1–13. DOI: 10.1080/21691401.2018.1434661.
- Sulaiman GM, Al Sammarrae KW, Ad’hiah AH, et al. Chemical characterization of Iraqi propolis samples and assessing their antioxidant potentials. Food Chem Toxicol. 2011;49:2415–2421.
- Frisch MJ, Trucks GW, Schlegel HB, et al. Gaussian 09, Revision A.02, Gaussian Inc., Wallingford, CT, USA; 2009.
- Benhusein GM, Mutch E, Aburawi S, et al. Genotoxic effect induced by hydrogen peroxide in human hepatoma cells using comet assay. Libyan J Med. 2010;5:1-6. DOI: 10.3402/ljm.v5i0.4637.
- Abd El-Rahman SN, Al-Jameel SS. Protection of curcumin and curcumin nanoparticles against cisplatin induced nephrotoxicity in male rats. Scholars Acad J Biosci. 2014;2:214–223.
- Dokoumetzidis A, Macheras P. A century of dissolution research: from noyes and whitney to the biopharmaceutics classification system. Int J Pharm. 2006;321:1–11.
- Tzeng CW, Yen FL, Wu TH, et al. Enhancement of dissolution and antioxidant activity of kaempferol using a nanoparticle engineering process. J Agric Food Chem. 2011;59:5073–5080.
- Gurushankar K, Gohulkumar M, Prasad NR, et al. Synthesis, characterization and in vitro anti-cancer evaluation of hesperetin-loaded nanoparticles in human oral carcinoma (KB) cells. Adv Nat Sci: Nanosci Nanotechnol. 2014;5:015006.
- Rejinold NS, Muthunarayanan M, Chennazhi KP, et al. 5-fluorouracil loaded fibrinogen nanoparticles for cancer drug delivery applications. Int J Biol Macromol. 2011;48:98–105.
- Krishnakumar N, Sulfikkarali N, Rajendra Prasad N, et al. Enhanced anticancer activity of naringenin-loaded nanoparticles in human cervical (HeLa) cancer cells. Biomed Prev Nutr. 2011;1:223–231.
- Gao H, Chen Y, Ma H, et al. Preparation and characterization of hesperidin - PEG 6000 complex. J Chem Soc Pakistan. 2014;36:848–851.
- Torrado G, Fraile S, Torrado S, et al. Process-induced crystallite size and dissolution changes elucidated by a variety of analytical methods. Int J Pharm. 1998;166:55–63.
- Khadka P, Ro J, Kim H, et al. Pharmaceutical particle technologies: an approach to improve drug solubility, dissolution and bioavailability. Asian J Pharm Sci. 2014;9:304–316.
- Cho SA, Cha SR, Park SM, et al. Effects of hesperidin loaded poly (lacticco-glycolic acid) scaffolds on growth behavior of costal cartilage cells in vitro and in vivo. J Biomaterials Sci Polym Ed. 2014;25:625–640.
- Moghimi SM, Hunter AC. Poloxamers and poloxamines in nanoparticle engineering and experimental medicine. Trends Biotechnol. 2000;18:412–420.
- Mauludin R, Muller RH. Physicochemical properties of hesperidin nanocrystal. Int J Pharm Pharmaceu Sci. 2013;5:954–960.
- Sulaiman GM. Molecular structure and anti-proliferative effect of galangin in HCT-116 cells: in vitro study. Food Sci Biotechnol. 2016;25:247–252.
- Mishra K. Structure-activity relationship of antioxidative property of hesperidin. Int J Pharm Erud. 2013;2:40–53.
- Kalpana KB, Srinivasan M, Menon VP. Evaluation of antioxidant activity of hesperidin and its protective effect on H2O2 induced oxidative damage on pBR322 DNA and RBC cellular membrane. Mol Cell Biochem. 2009;323:21–29.
- Cai W, Chen Y, Xie L, et al. Characterization and density functional theory study of the antioxidant activity of quercetin and its sugar-containing analogues. Eur Food Res Technol. 2014;238:121–128.
- Hosseinimehr SJ, Jalayer Z, Naghshvar F, et al. Hesperidin inhibits cyclophosphamide-induced tumor growth delay in mice. Integr Cancer Ther. 2012;11:251–256.
- Choi J, Reipa V, Hitchins VM, et al. Physicochemical characterization and in vitro hemolysis evaluation of silver nanoparticles. Toxicol Sci. 2011;123:133–143.
- Coutinho L, Oliveira H, Pacheco AR, et al. Hesperetin-etoposide combinations induce cytotoxicity in U2OS cells: implications on therapeutic developments for osteosarcoma. DNA Repair (Amst). 2017;50:36–42.
- Al-Ashaal HA, El-Sheltawy ST. Antioxidant capacity of hesperidin from citrus peel using electron spin resonance and cytotoxic activity against human carcinoma cell lines. Pharm Biol. 2011;49:276–282.
- Makin G, Hickman JA. Apoptosis and cancer chemotherapy. Cell Tissue Res. 2000;301:143–152.
- Natarajan N, Thamaraiselvan R, Lingaiah H, et al. Effect of flavonone hesperidin on the apoptosis of human mammary carcinoma cell line MCF-7. Biomed Prev Nutr. 2011;1:207–215.
- Park HJ, Kim MJ, Ha E, et al. Apoptotic effect of hesperidin through caspase3 activation in human colon cancer cells, SNU-C4. Phytomedicine. 2008;15:147–151.
- Chahar MK, Sharma N, Dobhal MP, et al. Flavonoids: a versatile source of anticancer drugs. Phcog Rev. 2011;5:1–12.
- Gaspar J, Laires A, Monteiro M, et al. Quercetin and the mutagenicity of wines. Mutagenesis. 1993;8:51–55.
- Flower NA, Brabu B, Revathy M, et al. Characterization of synthesized silver nanoparticles and assessment of its genotoxicity potentials using the alkaline comet assay. Mutat Res. 2012;742:61–65.
- Valavanidis A, Vlachogianni T, Fiotakis C. 8-hydroxy-2'-deoxyguanosine (8-OHdG): A critical biomarker of oxidative stress and carcinogenesis. J Environ Sci Health, Part C: Environ Carcinogen Ecotoxicol Rev. 2009;27:120–139.
- Baskar R, Lee KA, Yeo R, et al. Cancer and radiation therapy: current advances and future directions. Int J Med Sci. 2012;9:193–199.