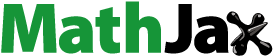
Abstract
Spinal cord injury (SCI) can lead to permanent and severe functional impairment below the lesion level and is still one of the most challenging clinical problems. The treatment of SCI has progressed with the development of tissue engineering techniques. Insulin-like growth factor 1 (IGF-1) and brain-derived neurotrophic factor (BDNF) are growth factors closely related to nerve regeneration. In this study, IGF-1 and BDNF were successfully immobilized on biodegradable graphene oxide (GO)-incorporated PLGA (PLGA/GO) electrospun nanofibres. The effect of PLGA/GO nanofibres with immobilized IGF-1 and BDNF on neurogenesis was investigated in vitro and in vivo utilizing MTT assays, immunofluorescence, motor function detection and histology observations. We demonstrated that PLGA/GO nanofibres loaded with IGF-1 and BDNF not only protected NSCs from oxidative stress induced by H2O2 but also enhanced NSC proliferation and neuronal differentiation in vitro. The in vivo study of an SCI animal model demonstrated that local delivery of IGF-1 and BDNF immobilized to PLGA/GO nanofibres significantly improved functional locomotor recovery, reduced cavity formation and increased the number of neurons at the injury site. Our study indicated that PLGA/GO is an effective carrier for IGF-1 and BDNF delivery and that immobilization of IGF-1 and BDNF onto PLGA/GO nanofibres has a great potential as a nerve implant for spinal cord injury applications.
Introduction
Spinal cord injury (SCI) can lead to permanent and severe functional impairment below the lesion level and is still one of the most challenging clinical problems [Citation1]. Although endogenous neural stem cells (NSCs) are immediately activated after SCI and migrate towards the injured site, the majority of endogenous NSCs differentiate into astrocytes that can form a glial scar, which blocks axonal regeneration, only a few newly generated neurons from activated NSCs have been identified after SCI [Citation2,Citation3]. In addition, the hostile pathological microenvironment is unfavourable for endogenous NSC and neuronal survival, which is considered to be another reason for the failure of endogenous mechanisms of self-repair [Citation4,Citation5]. Recently, increasing research has been performed to promote the survival of endogenous NSCs and their differentiation into neurons by transplanting biomaterials combined with different biological factors to spinal cord injury sites [Citation6–8]. Among the most commonly used biomaterials, such as chitosan, PLGA, and collagen, PLGA has attracted great interest because of its excellent biodegradability, mechanical properties, low immunogenicity and adjustable degradation rate [Citation9,Citation10]. However, the hydrophobicity of PLGA does not promote cell adhesion and PLGA does not have bioactivity on neurogenesis. Therefore, combining biomaterials with growth factors to enhance their neural regeneration performance is a promising strategy to address this problem.
Insulin-like growth factor 1 (IGF-1) is a mitogenic factor that can strongly enhance NSC proliferation both in vitro and in vivo [Citation11,Citation12]. Evidence indicates that IGF-1 can promote cell survival by inhibiting apoptosis, preventing neuronal death in neurodegenerative diseases and restraining oxidative stress and DNA damage, which demonstrates that IGF-1 has a clear neuroprotective effect [Citation13–15]. In addition, IGF is also involved in the regulation of NSC migration mediated by the activation of the PI3K pathway and by the phosphorylation of the reelin signal transducer [Citation16]. Although IGF-1 can also promote the differentiation of NSCs, it cannot induce neuronal differentiation effectively, mainly in astrocytes [Citation17]. In particular, studies have shown that IGF-1 significantly promoted the differentiation of NSCs into astrocytes after a nerve injury caused by ischaemia [Citation18]. Astrocytes can greatly increase the formation of glial scars after SCI, blocking nerve regeneration and circuit formation. Brain-derived neurotrophic factor (BDNF) is extensively distributed in the developing and mature nervous system, which mainly contributes to neuronal differentiation and survival. BDNF can facilitate the differentiation rate of newly generated neurons, mature neurons and interneurons and can reduce astrocyte differentiation to some extent [Citation19]. Furthermore, BDNF plays a direct role in NSC antioxidant defences by enhancing the activity of antioxidant enzymes in contrast to other common neurotrophins, such as NGF and NT-3, which is beneficial for neuronal viability [Citation20]. To enable more endogenous NSC survival, proliferation, and neuronal differentiation after SCI, we chose a combination of IGF-1 and BDNF for this study to achieve an improved therapeutic effect.
The typical procedure of combining growth factors or drugs into polymer materials involves blending together growth factors and polymer materials during polymer production. However, the bioactivity of proteins can be impaired by using organic solvents and strong electric fields during production. Several new methods for the consistent immobilization of growth factors have been investigated, such as photoreactive gelatine and poly (dopamine) coatings. Graphene oxide (GO) nanosheets, derived from the oxidation of graphene, are a unique monolayer of sp2- and sp3-hybridized carbon atoms organized in a two-dimensional lattice. GO has great application potential in a variety of fields due to its distinct physicochemical characteristics, including large loading volume, hydrophilic functional groups, low toxicity, excellent biocompatibility and extraordinary mechanical properties [Citation21]. Moreover, GO can bind tightly with different proteins via electrostatic interactions caused by its distinct structure [Citation22,Citation23], which has already been applied in the field of osteogenesis by combining GO and bone morphogenetic protein-2 [Citation22]. Recent studies have also shown that GO has great utilization potential in the field of neuroscience. GO not only stimulates the differentiation of NSCs into neurons [Citation24,Citation25] but also promotes neuronal axonal regeneration and orientation [Citation26,Citation27]. Therefore, implanting graphene-polymer hybrid scaffolds combined with IGF-1 and BDNF at the injury site may be a promising method to treat spinal cord injury.
In this study, we produced graphene oxide-incorporated PLGA electrospun nanofibres loaded with IGF-1 and BDNF. The influence of different nanofibres on NSC survival, proliferation and differentiation was investigated in vitro. Then, we used a T9 spinal cord hemisection rat model and implanted graphene-polymer hybrid nanofibres combined with IGF-1 and BDNF into the injury site. Finally, motor function assessment and histology detection were employed to evaluate the therapeutic efficacy.
Materials and methods
Fabrication of PLGA/GO electrospun nanofibres
PLGA (lactide/glycolide ratio = 80/20, Mn = 85,000) was purchased from Changchun Institute of Applied Chemistry, China, and GO was purchased from Chengdu Organic Chemicals Co. Ltd, China (thickness = 0.55–1.2 nm and diameter = 0.5–3 μm). PLGA/GO nanofibres were prepared by electrospinning the admixture of PLGA and GO. Briefly, 0.05 g GO was dissolved in 1 ml 1,1,1,3,3,3-hexafluoroisopropanol (HFIP) to prepare the GO suspension, and 0.95 g PLGA was dissolved in 9 ml HFIP to prepare the PLGA solution. Then, the GO suspension was added to PLGA solution drop-wise and the resulting solution was gently stirred overnight at room temperature to obtain a homogenous suspension. The mixed solution was directly electrospun onto an aluminium foil-covered collector (applied voltage = 40 kV, air gap distance = 10 cm, inner diameter of spinneret = 0.4 mm and flow rate of the solution = 0.07–0.10 ml min−1). The collected electrospun mats were dried in vacuum at room temperature for approximately 48 h to remove the residual organic solvent.
Characterization
Scanning electron microscopy (SEM, XL30 ESEM-FEG, FEI, USA) was used to observe the surface morphology of the nanofibres. X-ray diffraction (XRD, D8 ADVANCE, Bruker, Germany) was used to determine the elemental composition of the electrospun nanofibres. To analyze the surface hydrophobicity, a static water contact angle measurement was obtained to evaluate the surface wettability of nanofibres using the sessile drop method on a contact angle system (VCA 2000, AST, USA).
Immobilization of IGF-1 and BDNF onto electrospun nanofibres
PLGA and PLGA/GO nanofibres were placed in a 24-well plate. A total of 2 ml IGF-1 (PeproTech, USA) and BDNF (PeproTech) with a concentration of 100 ng/mL was added into each well collectively for 6 h. PLGA/GO nanofibres were completely dried under vacuum for 1 d and prepared for use in the following experiments. The nanofibres were then washed with distilled water three times to remove unattached peptides. Furthermore, samples with immobilized BDNF alone onto electrospun nanofibres were also prepared and treated under similar conditions with the same concentration.
Characterization of immobilized growth factors
The electrospun nanofibres (PLGA and PLGA/GO) were placed into a 24-well plate. A total of 2 ml IGF-1 (100 ng/mL in PBS) was added to each well for 6 h at room temperature on a shaker. The supernatants were collected and then IGF-1 immobilized nanofibres were washed with PBS three times. The washing liquid was collected and mixed with the previously collected supernatants. The binding efficiency and release profiles of IGF-1 were obtained by an enzyme-linked immunosorbent assay (ELISA) kit. The binding efficiency of IGF-1 was evaluated according to the following formula:
where Wa and Wb are the weight of IGF-1 in PBS solution before and after incubation of the different nanofibres, respectively. The release profiles of IGF-1 were obtained by ELISA for 21 successive days according to the manufacturer’s instructions using a microplate reader (Tecan Infinite M200, Switzerland). All experiments were performed in triplicate and the quantitative values were expressed as the mean ± standard deviation. The determination of immobilized BDNF alone was conducted using the same protocol.
Neural stem cell cultures
Neural stem cells were isolated from the cerebral cortex of embryonic mice (E11.5) as previously described [Citation28]. The dissociated cells were cultured in a defined medium and purified at a density of 50,000 cells/cm2 in T25 culture flasks (Corning, NY, USA) and incubated at 37 °C in a 5% CO2 humidified incubator. The growth media contained neurobasal media (GIBCO/Invitrogen, USA), B27 neural supplement (GIBCO/Invitrogen), 100 ng/mL penicillin-streptomycin (Gibco-Invitrogen), 20 ng/mL epidermal growth factor (EGF, Sigma) and 20 ng/mL basic fibroblast growth factor (bFGF, Sigma). NSCs were passaged every 5 d, and cells between passages 2 to 6 were used for experiments.
Cell proliferation assay
The nanofibres were first transferred to a 24-well plate and incubated in 1 ml 10 ng/ml poly-D-lysine (Sigma) overnight in an incubator. NSCs were dissociated and seeded at a density of 20,000 cells/cm3 on the nanofibres. 3–(4,5-dimethyl-2-thiazolyl)-2,5-diphenyl-2- H-tetrazolium bromide (MTT) was used to assess cell proliferation according to the manufacturer’s instructions. Briefly, 50 μL of MTT (Sigma) stock solution diluted in PBS was added to each well, and the cells were incubated at 37 °C for 4 h. Then, the medium was removed, and 750 μL of acidified isopropanol was added to each well. The optical density (OD) was measured at a wavelength of 540 nm on a full wavelength microplate reader (Infinite M200, TECAN).
Cell survival assay
NSCs were dissociated using Accutase (GIBCO/Invitrogen) to a single-cell suspension and seeded on different nanofibre samples in a 24-well plate containing growth media at a density of 30000 cells/cm3. After incubation for 24 h, H2O2 was added into each well at a concentration of 100 μM, followed by incubation for an additional 24 h at 37 °C in a 5% CO2 humidified incubator. An MTT assay was performed to evaluate cell viability according to the protocol described above. The absorbance was measured using a microplate reader at a wavelength of 540 nm, and the surviving cell number was correlated with the optical density.
Immunofluorescence staining
Immunofluorescence staining was performed to evaluate NSC differentiation on different electrospun nanofibre samples. Differentiation of NSCs was induced by differentiation medium containing 1% foetal bovine serum (FBS, Life Technologies) without bFGF and EGF. NSCs were cultured on the nanofibres for 7 d under differentiation conditions. Then, the cells were washed with PBS, fixed in 4% paraformaldehyde for 60 min, extracted with 0.1% TritonX-100 (Sigma) for 10 min and blocked with 10% goat serum (Sigma) for 2 h. Then, primary antibodies were added and incubated overnight at 4 °C. Afterwards, secondary antibodies were added and incubated for 2 h at room temperature, followed by 4′,6-diamidino-2-phenylindole (DAPI) staining. Primary antibodies included Nestin as a marker for NSCs (1:200 dilution, Abcam), Tuj-1 as a marker for neurons (1:200 dilution, Abcam) and GFAP as a marker for astrocytes (1:400 dilution, Abcam). Protein expression of the stained cells was observed on a confocal laser scanning microscope (LSM 780, ZEISS).
Quantitative real-time PCR analysis
NSCs cultured on the various nanofibres for 7 d were also collected for the evaluation of gene expression. Total RNA was extracted using TRIzol Reagent (Invitrogen) according to the manufacturer’s protocol. The total RNA concentration and purity were measured by a Nanodrop (Tecan M200). RNA samples were reverse-transcribed into cDNA for qPCR using the PrimeScriptTM reagent kit with gDNA Eraser (Takara). The primer sequences specific for the target genes used for qRT-PCR are listed in . Then, qPCR amplification was performed as follows: initial denaturation at 95 °C for 10 min, 40 cycles at 95 °C for 30 s, 58 °C for 1 min and 72 °C for 1 min. Each gene expression value was normalized to the glyceraldehyde-3-phosphate dehydrogenase (GAPDH) housekeeping gene.
Table 1. List of genes and primer nucleotide sequences.
Spinal cord injury model
All animal procedures complied with the Guidelines for the Care and Use of Laboratory Animals and were under the supervision of the Institutional Animal Care and Use Committee of Jilin University. Female SD rats (200–250 g) were purchased from the Jilin University animal centre. Female SD rats were randomly divided into the following groups: SCI treated with vehicle PLGA membranes (n = 10), SCI treated with PLGA/GO membranes (n = 10), SCI treated with PLGA/GO/IGF-1 membranes (n = 10), SCI treated with PLGA/GO/BDNF membranes (n = 10) and SCI treated with PLGA/GO/IGF-1 + BDNF membranes (n = 10). The rat spinal cord transection model was established as described previously [Citation7]. Briefly, the rats were anaesthetized with 7% chloralic hydras (300 mg/kg bodyweight). A longitudinal incision was made on the midline of the back, and the paravertebral muscles were moved to expose the spinal column. A dorsal laminectomy of the thoracic vertebra (T9-T10) was performed using fine rongeurs, and spinal cord tissue was hemisected with fine microdissection scissors. A piece of an electrospun nanofibre mat was placed on the surface of the corresponding groups to cover the hemisected area. Cotton swabs were used to stop bleeding. Then, the soft tissues were sutured, and the skin was closed.
Assessment of locomotor recovery
The BBB (Basso, Beattie, and Bresnahan) locomotor rating scale was adopted to evaluate the functional recovery of hindlimbs. Animals were assigned new identification codes after surgery to ensure blind rating of behaviour performances. BBB is a 21-point scale designed to assess hindlimb locomotor recovery after spinal cord injury. Because animals were subjected to hemisection injury, right (ipsilateral) and left (contralateral) hindlimbs were assessed separately by two examiners.
Motor evoked potential (MEP) detection
MEP detection was conducted using electromyography to evaluate the response of muscles. Rats were anaesthetized and then fixed in the prone position for recording motor evoked potential (MEP) detection. For MEP recordings, the stimulating electrodes were placed in the cortical motor area, and the reference electrode was inserted subcutaneously. The recording electrodes were placed in the gastrocnemius muscle, and waveforms were recorded on evoked potential equipment (stimulus intensity = 48.0 V).
Histology and immunofluorescence
At 4 weeks after surgery, the spinal cord from T6-T10 was retrieved and fixed in 4% (v/v) formaldehyde for 48 h. The segments were then cut into 15 mm thick sections (Leica Microsystems) and embedded in OCT. Tissue sections obtained by a cryostat were then subjected to haematoxylin and eosin staining and immunofluorescent staining following standard protocols. Image-Pro Plus software (Media Cybernatics LP, Maryland, USA) was used to screen and calculate the cavity area. In brief, the scale of the picture was determined before analysis. Then, the images were converted to 8 bits and were processed in the menu “Adjust - threshold”. Finally, “Analyze - measure” in the software automatically calculated the selected areas. For immunofluorescence staining, the spinal cord tissue 2 mm from the lesion centre was chosen for our following immunofluorescence analysis to extract equivalent regions from different rats spinal cord. The sections were subjected to heat-mediated antigen retrieval using citric acid and blocked using normal horse serum/T-PBS (1:20) for 15 min. Primary antibodies were applied to the sections and incubated overnight at 4 °C. The primary antibodies were against the following proteins: NeuN (1:500 dilution, Abcam) and GFAP (1:800 dilution, Abcam). Cell nuclei were stained with DAPI (1:1000 dilution, Sigma), and images were acquired using a Leica TCS SP8 confocal microscope (Leica Microsystems, Germany). Image-Pro Plus software was used to quantify positive immunostained cells and immunostained positive area under 40x magnification in 6 fields of one section.
Statistical analysis
All quantitative data were analysed with GraphPad Prism 5.0 software and presented as the mean ± standard deviation. Multiple group comparisons were made using one-way analysis of variance (ANOVA) followed by Bonferroni post-hoc test. Two-group comparisons were tested by Student’s test. In addition, two-way repeated-measures ANOVA was specifically used for BBB score evaluation. P < .05 was considered to indicate a statistically significant difference.
Results
Characterization of PLGA/GO nanofibres
The microstructure and average fibre diameter of the PLGA and PLGA/GO electrospun nanofibres were observed by SEM. As shown in , uniform and smooth nanofibres with random orientation were fabricated from PLGA by electrospinning. Although the microstructure of PLGA/GO fibres did not significantly change, the mean fibre diameter decreased from 1256.8 ± 387.2 nm in PLGA nanofibres to 998.6 ± 217.3 nm in PLGA/GO nanofibres. The XRD patterns of PLGA and PLGA/GO nanofibres were collected to determine the surface chemical properties. The GO pattern showed a characteristic peak at 2θ ≍ 10° and an interlayer spacing of approximately 0.80 nm, which is the typical separation of layered GO (). The XRD patterns of PLGA/GO nanofibres exhibited a similar diffraction peak at 2θ ≍ 10° (). Contact angles of the PLGA and PLGA/GO samples were performed to observe the hydrophilicity of the nanofibre surface. As shown in , the contact angles of PLGA and PLGA/GO were 98.7 ± 7.9 and 79.2 ± 3.6, respectively, which indicates that the addition of GO to PLGA can enhance nanofibre hydrophilicity.
Binding efficiency and release profiles of IGF-1 and BDNF from nanofibres
The binding efficiency and release profiles of IGF-1 and BDNF were identified by ELISA. As shown in , the binding efficiencies of IGF-1 and BDNF on PLGA/GO nanofibres were approximately 2.9 and 2.2 times greater than those on PLGA nanofibres, respectively. This result demonstrated that the addition of GO to the surface of nanofibres significantly enhanced growth factor adsorption. Furthermore, the release profile showed that there was a burst release of 35% IGF-1 and 40% BDNF from the PLGA/GO nanofibres, respectively, within 3 d. The release rate subsequently decreased with approximately 68% IGF-1 and 64% BDNF released after 21 d. However, for the pure PLGA nanofibres, a burst release of 79% and 74% of IGF-1 and BDNF, respectively, was observed on the first day. At 21 d, approximately 93% IGF-1 and 95% BDNF were released from the PLGA nanofibres.
Proliferation of NSCs on the nanofibres
NSCs have a strong self-renewal ability and can differentiate into neurons and glial cells, which is appropriate for the evaluation of the neurogenesis potential of nerve-related biomaterials in vitro. Since Nestin is a key marker for NSCs, the purity of neurospheres was assessed by Nestin staining, as shown in . The results indicated that NSCs were positive for Nestin and could be used for subsequent studies. Enhancement of NSC proliferation on the nanofibres is a crucial factor for promoting neurogenesis. NSC proliferation was investigated to evaluate how the added GO and growth factors affected cellular metabolism. As shown in , NSC proliferation was quantitatively monitored by an MTT assay to measure the metabolic activity of the total population of cells at 1, 3 and 7 d. The OD value of NSCs on different samples increased gradually with prolonged culture time. The cell viability showed the greatest OD value in the PLGA/GO/IGF + BDNF group at 1, 3 and 7 d. The OD value was significantly greater in the PLGA/GO/IGF groups than that in the PLGA/GO/BDNF groups at 3 and 7 d (p < .05, ), although there was no significant difference between these two groups at 1 d. Furthermore, we found that the OD value in the PLGA/GO group was higher than that in the PLGA group at 7 d (p < .05, ), which may be due to the increased hydrophilicity of PLGA/GO nanofibres.
Survival rate of NSCs on the nanofibres
The overproduction of reactive oxygen species (ROS) and inflammation in the microenvironment after SCI is one of the most important reasons for failed nerve regeneration. Therefore, the neuroprotective effect of grafts is important for its application in SCI. In our study, H2O2 was used to simulate the microenvironment after SCI. The improvement of various nanofibres for the survival of NSCs was evaluated by an MTT assay. As shown in , PLGA/GO with IGF-1 and/or BDNF immobilization significantly improved the survival rate compared to the PLGA and PLGA/GO groups. PLGA/GO/IGF-1 protected 58% of NSCs from H2O2-induced cell losses, while PLGA/GO/BDNF prevented 51% of cell death. Furthermore, the survival rate in PLGA/GO/IGF + BDNF was dramatically greater than that in the other groups, which showed a synergistic effect between IGF-1 and BDNF. Although GO can promote NSC proliferation, GO did not appear to affect NSC survival under harsh environmental conditions based on our results.
Differentiation of NSCs on the nanofibres
The potential of different nanofibres to affect NSC differentiation was evaluated by RT-PCR and immunofluorescence staining. The Tuj-1 primary antibody, a marker for neurons, and GFAP, a marker for astrocytes, were chosen in our study. As shown in , the mRNA level of Tuj-1 was significantly greater for cells on PLGA/GO nanofibres than that for cells on PLGA nanofibres (p < .05, ), which indicated that GO incorporation enhanced neuronal differentiation. After IGF-1 and BDNF immobilization, cells on modified nanofibres showed greater expression levels of Tuj-1 than cells on pure PLGA nanofibres, while the Tuj-1 level of cells in the PLGA/GO/BDNF group was greater than that in the PLGA/GO/IGF group. An obvious increase in Tuj-1 mRNA expression was observed for cells in the PLGA/GO/IGF + BDNF compared with that in the other groups. GFAP gene expression in the PLGA, PLGA/GO and PLGA/GO/BDNF groups was observed without a significant difference. Furthermore, GFAP gene expression of cells on PLGA/GO/IGF was significantly greater than that of cells on PLGA/GO/BDNF and PLGA/GO/IGF + BDNF after the cells were cultured for 7 d (p < 05, ). To better observe NSC differentiation, the protein expression of Tuj-1 and GFAP in NSCs was evaluated by immunofluorescence staining. As shown in , after 7 d of culture, Tuj-1 protein expression was evident in the PLGA/GO/IGF + BDNF groups, while the PLGA/GO/IGF groups had the most significant GFAP expression.
Figure 8. Qualitative and quantitative measurement of NSCs differentiation. Immunocytochemical staining of differentiated neurons and astrocytes on pure PLGA nanofibres (A), PLGA/GO nanofibres (B), PLGA/GO/BDNF nanofibres (C), PLGA/GO/IGF nanofibres (D), and PLGA/GO/IGF + BDNF nanofibres (E). Immunostaining makers are Tuj-1 for neurons, GFAP for astrocytes and DAPI for nuclei. The Scale bar was 100 µm. Quantitative real-time PCR analysis of Tuj-1 (F) and GFAP (G) after NSCs cultured for 7 days. (∗, p < .05, n = 4).
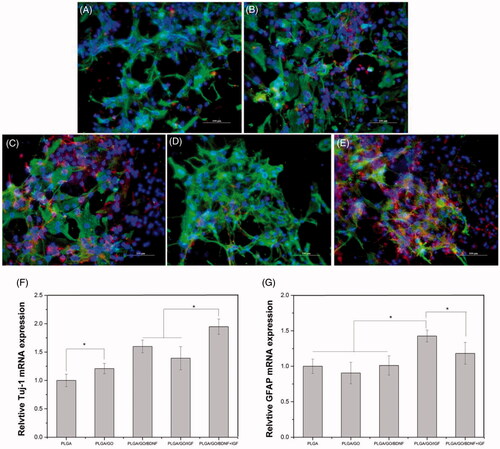
Motor function evaluation
After evaluating nanofibres in vitro, rat motor function was assessed using the BBB locomotor rating scale and electrophysiological measurements for up to 4 weeks. All rats became paralyzed in the left hindlimb first after SCI and then displayed gradual partial recovery within days. Compared with PLGA or PLGA/GO nanofibres, the implantation of PLGA/GO immobilized with growth factors significantly increased the BBB scores (p < .05, ). Furthermore, PLGA/GO/IGF + BDNF membrane-treated rats showed the best outcome among all groups. To confirm motor functional regeneration, electrophysiological analysis was also performed at 4 weeks post-injury. For MEP, the amplitude was dramatically greater in the PLGA/GO/IGF + BDNF, PLGA/GO/IGF and PLGA/GO/BDNF groups compared to that in the PLGA and PLGA/GO groups (p < .05, ).
Histological analysis
In our study, haematoxylin and eosin staining on longitudinal sections after SCI was conducted to investigate the effects of different nanofibres on reducing cavity formation. As shown in , visible cavities of varying sizes were observed in the injury site of the spinal cord four weeks after nanofibre implantation. This observation was further confirmed by a quantitative assessment of the longitudinal serial sections of the injury site. The average cavity area in the PLGA/GO/IGF and PLGA/GO/BDNF groups was significantly smaller than that in the PLGA and PLGA/GO groups (p < .05, ), which indicated that the stable release of IGF or BDNF could be beneficial to decreasing the cavity size in the lesioned site. Furthermore, the minimum mean cavity area of spinal cord tissue was determined in the PLGA/GO/IGF + BDNF groups.
Figure 10. Longitudinal HE staining of spinal cord tissue after 4 weeks post-operation. (A) PLGA group. (B) PLGA/GO group. (C) PLGA/GO/IGF group. (D) PLGA/GO/BDNF group. (E) PLGA/GO/IGF/BDNF group. The Scale bar was 500 µm. (F) Quantification of the cavity areas of spinal cord tissue. (∗, p < .05, n = 4).
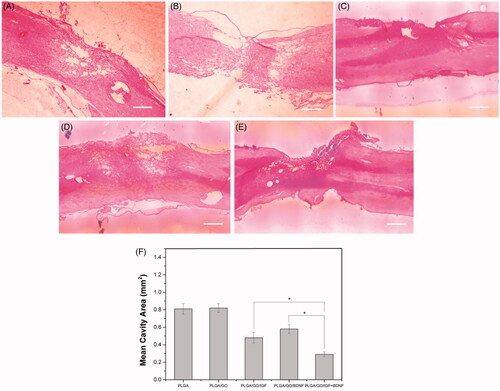
Immunofluorescence analysis
NeuN immunoreactivity was examined as a mature neuron-specific marker in the spinal cord tissue. The transection of the spinal cord segments manifested a considerable enhancement in NeuN-positive neurons by implantation of PLGA/GO nanofibres binding with single and multiple growth factors compared to PLGA or PLGA/GO nanofibres. NeuN-positive neurons were found to be significantly more prevalent in the lesion area in the PLGA/GO/IGF + BDNF group than those in the PLGA/GO/IGF or PLGA/GO/BDNF groups (p < .05, ). GFAP is a specific cellular marker for differentiated astrocytes, which is also one of the main components of a glial scar. As shown in , although rats treated with PLGA/GO nanofibres with immobilized IGF-1 and BDNF had a lower GFAP-positive area than rats treated with either PLGA or PLGA/GO nanofibers, the GFAP-positive area had no significant difference among all of the five groups (p > .05, ).
Figure 11. Qualitative and quantitative evaluation of neurons in the injury site after SCI. Immunocytochemical staining of neurons on pure PLGA nanofibers (A), PLGA/GO nanofibers (B), PLGA/GO/IGF nanofibers (C), PLGA/GO/BDNF nanofibers (D) and PLGA/GO/IGF + BDNF nanofibers (E). Immunostaining makers are NeuN for neurons and DAPI for nuclei. The Scale bar was 100 µm. (F) Mean NeuN positive cells per visual field. (∗, p < .05, n = 3).
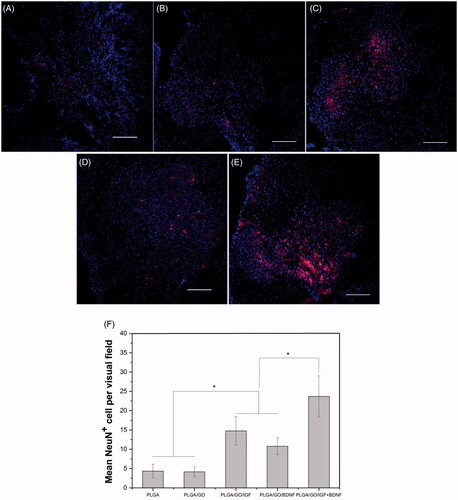
Figure 12. Qualitative and quantitative evaluation of astrocytes in the injury site after SCI. Immunocytochemical staining of astrocytes on pure PLGA nanofibers (A), PLGA/GO nanofibers (B), PLGA/GO/IGF nanofibers (C), PLGA/GO/BDNF nanofibers (D) and PLGA/GO/IGF + BDNF nanofibers (E). Immunostaining makers are GFAP for astrocytes and DAPI for nuclei. The Scale bar was 200 µm. (F) The percentage of GFAP positive area per visual field.
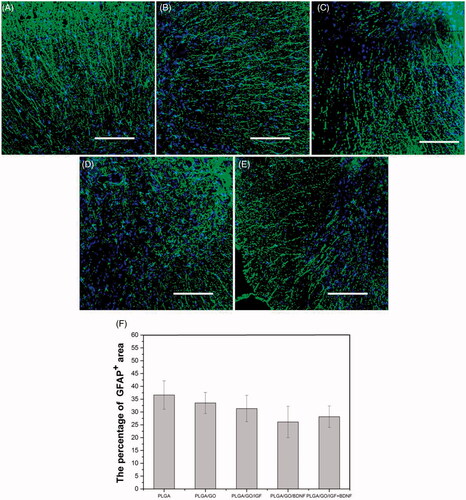
Discussion
The treatment of SCI is still one of the greatest challenges in spinal surgery. In this study, we demonstrated that local delivery of IGF-1 and BDNF immobilized to PLGA/GO nanofibres promotes SCI recovery. First, we fabricated PLGA/GO electrospun fibres and evaluated their characteristics. The SEM results showed that the mean fibre diameter decreased in the PLGA/GO group compared to that in the PLGA group, which was likely due to the change in solution conductivity and viscosity caused by the GO. The XRD patterns of PLGA and PLGA/GO nanofibres were obtained to determine the surface chemical properties, and our results suggested that GO was successfully embedded in the nanofibres. The contact angle in the PLGA/GO group was significantly less than that in the PLGA group, which indicates that GO enhanced nanofibre hydrophilicity. The hydrophilicity of materials plays an important role in cell adhesion and protein adsorption. Our contact angle results demonstrated that GO dramatically improved the hydrophilicity of nanofibre surfaces and favoured cell adhesion and growth factor delivery.
To determine the binding efficiency and release profile of growth factors, an ELISA assay was performed in this study. Recent studies have shown that GO has strong capabilities to adsorb various proteins, including cytochrome c, bovine serum albumin, ribonuclease A, and protein kinase A [Citation29,Citation30]. Our results further demonstrated that GO effectively adsorbed growth factors, such as IGF-1 and BDNF, which indicated that GO incorporation in the biomaterial could facilitate the delivery of growth factors. The ability for GO to bind proteins is related to its distinct structure and functional groups. The π-electron cloud of GO can interact with the hydrophobic core of proteins [Citation31]. In addition, GO can non-covalently bind proteins because of H-bonding and electrostatic interactions [Citation32]. Combined with the results of the contact angle measurements, we suspect that GO increased the hydrophilicity of the nanofibre surface, which is favourable for protein immobilization [Citation33]. Based on the release profile results, PLGA/GO not only reduced the burst release of IGF-1 and BDNF but also maintained a stable growth factor release rate. These results demonstrate that PLGA/GO nanofibres are effective carriers for growth factor delivery and have the potential to be used for SCI treatment.
To investigate NSC growth on the various nanofibres under physiological and inflammatory conditions, we used an MTT assay to evaluate cell viability. Our results indicated that PLGA/GO/IGF + BDNF effectively enhanced NSC proliferation. Enhancement of NSC proliferation on the nanofibres is a crucial factor for promoting neurogenesis. IGF-1 and BDNF are important biologically active substances in the nervous system and play vital roles in maintaining normal growth and promoting regeneration of the nervous system. Our results strongly corroborated previous reports, indicating that IGF-1 and BDNF treatment enhance the proliferation of NSCs in vitro [Citation34–36]. Although these two growth factors can both improve NSC proliferation, nanofibres immobilized with IGF-1 had an improved effect on the proliferation of NSCs on their surface, suggesting that IGF-1 played a more important role in NSC proliferation than BDNF at a concentration of 100 ng/ml. Among the various nanofibres, the PLGA/GO/IGF + BDNF nanofibres were the most effective at enhancing NSC proliferation in this study, demonstrating that the combination of IGF-1 and BDNF had a synergistic effect on cell proliferation. Furthermore, GO enhanced cell proliferation at 7 d. We speculate that having more proteins in the culture medium to bind to the PLGA/GO surface as well as increasing biomaterial hydrophilicity improved NSC adhesion and may be responsible for improved NSC proliferation in PLGA/GO nanofibres rather than pure PLGA nanofibres at 7 d. Our results showed that dual immobilization of IGF-1 and BDNF on PLGA/GO nanofibres dramatically promoted NSC proliferation, which is important for the initial phase of neurogenesis.
Oxidative stress plays an important role in the pathogenesis of SCI [Citation37,Citation38]. High levels of ROS, including superoxide, hydroxyl radicals, and hydrogen peroxide, can lead to lipid peroxidation, oxidative chain reactions and damage to cellular macromolecules, which leads to the death of host nerve cells [Citation39]. H2O2 treatment can induce oxidative stress in vitro, which can be used to imitate the microenvironment after SCI injury [Citation40]. In the present study, we aimed to assess the neuroprotective effect of different nanofibres against oxidative stress. Recent studies have shown that IGF-1 and BDNF are both growth factors that promote the survival of mature neurons [Citation41,Citation42]. Based on our results, the utility of IGF-1 and BDNF as survival factors for NSCs was also demonstrated. However, IGF-1 was apparently more potent than BDNF in protecting NSCs against H2O2-induced cell death. Several types of studies revealed that IGF-1 prevented neuronal death more effectively than BDNF [Citation43,Citation44], and we suggest that this mechanism also applies to NSCs. Furthermore, synergistic effects between IGF-1 and BDNF on protecting NSCs from oxidative stress were also confirmed. Therefore, we demonstrated that PLGA/GO/IGF + BDNF had the best effect on NSC proliferation and neuroprotection among the nanofibres investigated in our study.
Although NSCs have the potential to differentiate into neurons and astrocytes, most endogenous NSCs spontaneously differentiate into astrocytes after SCI and form glial scars after SCI, thus inhibiting nerve regeneration and circuit formation. Therefore, the induced neuronal differentiation capability of implanted biomaterials is an important factor for the evaluation of the application value of implanted biomaterials [Citation45,Citation46]. In this study, the potential of different nanofibres to affect NSC differentiation was evaluated by RT-PCR and immunofluorescence staining. Our results showed that GO had a positive effect on guiding NSCs to differentiate into neurons, which is consistent with previous research [Citation47,Citation48]. GO incorporation in PLGA improved surface properties, which may provide a unique microenvironment to permit neuronal formation. Nevertheless, the intracellular signalling pathways related to GO-induced neuronal differentiation still need to be explored. After BDNF immobilization on the surface of the nanofibres, NSCs demonstrated a clear neuronal differentiation trend. However, IGF-1 immobilization on the graphene-based nanofibres enhanced the NSC differentiation rate of both neurons and astrocytes, especially in the astrocyte direction. Our results indicate that BDNF had a specific effect on NSC neuronal differentiation, and IGF-1 had a specific effect on astrocyte differentiation. Our study also revealed that BDNF combined with IGF-1 immobilization induced the development of significantly more neurons than single growth factors. Furthermore, multiple growth factors immobilized in the nanofibres only slightly increased astrocyte formation. NSC differentiation requires the synergistic effects of multiple neurotrophic factors working in the microenvironment to form a complex regulatory network. Our findings are important in the context of spinal tissue repair after SCI, implying that immobilizing both BDNF and IGF-1 on graphene-based nanofibres had synergistic effects to enhance neuronal differentiation of NSCs.
After evaluating nanofibres in vitro, a rat spinal cord hemisection model was established and treated with different nanofibres. Our results showed that PLGA/GO/IGF + BDNF membrane treatment promoted motor function, reduced cystic cavity and improved neurogenesis in the injury site. In this study, BBB scoring and MEP evaluation both indicated that local implantation of PLGA/GO/IGF + BDNF nanofibres improved motor functional recovery in SCI rats. Several studies have demonstrated that IGF-1 and BDNF can be used to treat spinal cord ischaemia-reperfusion injury and traumatic brain injury through intravenous and intraperitoneal injections [Citation49–51]. Our results further demonstrated that IGF-1 and BDNF incorporation with PLGA/GO nanofibres at the injury site significantly improved functional recovery. The cystic cavity in spinal cord tissue is a typical pathological feature after SCI, which forms a natural physical obstacle for nerve regeneration. A smaller cavity volume is more beneficial for nerve regeneration. Our results indicate that localized delivery of IGF-1 and BDNF after SCI synergistically enhanced filling the cavity of the injury site and therefore, provided conditions for spinal tissue regeneration. Combined with our in vitro results, we speculated that immobilized growth factors on nanofibres had a neuroprotective effect, providing an improved microenvironment for spinal cord tissue growth.
Finally, neurons and astrocytes in the injury region were observed by immunofluorescence analysis. After SCI, endogenous NSCs differentiated into neurons with host neurons migrating towards the SCI site, causing neural network reconstruction and nerve injury repair [Citation6,Citation52]. A strong correlation was found between the number of neurons in the injury region and neurogenesis [Citation8,Citation53,Citation54]. Therefore, the quantity of neurons in the SCI site was an important factor for the evaluation of the therapeutic effect of the grafting biomaterial. Our results indicated that single and combined growth factor therapy increased neuronal viability after SCI and that there was an obvious synergistic effect between IGF and BDNF. The survival rate of host nerve cells and the neuronal differentiation rate of endogenous NSCs in the SCI site were two dominant factors that affected neuron quantity in the injury site. Our in vitro results demonstrated that PLGA/GO combined with IGF and/or BDNF demonstrated a clear neuroprotective effect and promoted NSC neuronal differentiation, which may have been responsible for the growing number of neurons in the lesioned site. Although PLGA/GO could not protect cells in the inflammatory microenvironment induced by H2O2 based on our results of cell experiments, PLGA/GO was able to dramatically improve NSC neuronal differentiation. However, NeuN-positive cells in the PLGA and PLGA/GO groups demonstrated no significant difference in the tissue after SCI. In addition, the PLGA/GO/IGF group demonstrated improved neuroprotection but a lower neuronal differentiation rate than the PLGA/GO/BDNF group in vitro. More NeuN-positive cells in the injury site after PLGA/GO/IGF implantation for 4 weeks were observed than PLGA/GO/BDNF implantation. Therefore, we concluded that between the two dominant factors closely related to neuronal viability, as mentioned above, the neuroprotective effect of biomaterials seemed to play a leading role in increasing the amount of neurons after SCI in an early phase within one month. After SCI occurred, in addition to most endogenous NSCs in the wounded site naturally differentiating into astrocytes, the original astrocytes near the injury site could also activate and migrate into the injury site, which eventually leads to glial scar formation [Citation55,Citation56]. Hence, reducing the size of glial scars was crucial for axonal regeneration and functional recovery after SCI. Our results in vitro showed that PLGA/GO/BDNF nanofibres maximally inhibited NSCs to differentiate into astrocytes among these five groups. However, our results in vivo indicated that local delivery of IGF-1 and/or BDNF from PLGA/GO nanofibres showed no significant difference in reducing the size of glial scars compared to PLGA/GO group. GFAP expression in vivo can be affected by not only newly born astrocytes originating from NSCs but also the original astrocytes migrated in the injured site, which may lead to the difference changed trend of astrocytes in vitro and vivo.
However, there were some limitations in this study. Firstly, our result in vitro showed that NSCs on the PLAG/GO nanofibres can proliferate better than that one the PLGA nanofibres, which indicated addition of GO into PLAG/GO nanofibres did not cause obvious cytotoxicity. However, the toxicity responses to PLAG/GO nanofibres in vivo should also be considered from a clinical perspective. Several studies had demonstrated that scaffolds composed of GO implanted in the injured rat spinal cord could not lead to inflammatory response or adverse reaction of the tissue near the implanted material, which concluded GO scaffolds had a good biocompatibility in vivo [Citation57,Citation58]. Although our study focused on the functional recovery after nanofibres implantation, a systematic evaluation of PLAG/GO nanofibres biocompatibility should also be inclusive in our further studies. Besides, a longer observation period after biomaterial implantation should be conducted, and the molecular mechanisms underlying neurogenesis following the application of this treatment need to be investigated in the future.
Conclusion
In summary, this report demonstrated that PLGA/GO electrospun nanofibres immobilized with IGF-1 and BDNF not only protected NSCs from oxidative stress induced by H2O2 but also enhanced NSC proliferation and neuronal differentiation in vitro. Localized delivery of IGF-1 and BDNF from PLGA/GO nanofibres improved functional locomotor recovery, reduced cavity formation and increased the number of neurons at the injury site. We demonstrated that PLGA/GO is an effective carrier for IGF-1 and BDNF delivery and that immobilization of IGF-1 and BDNF onto PLGA/GO nanofibres has great potential as a nerve implant for spinal cord injury applications.
Disclosure statement
No potential conflict of interest was reported by the authors.
Correction Statement
This article was originally published with errors, which have now been corrected in the online version. Please see Correction (http://dx.doi.org/10.1080/21691401.2020.1738654)
Additional information
Funding
References
- Sezer N, Akkus S, Ugurlu FG. Chronic complications of spinal cord injury. WJO. 2015;6:24–33.
- Wang B, Xiao Z, Chen B, et al. Nogo-66 promotes the differentiation of neural progenitors into astroglial lineage cells through mTOR-STAT3 pathway. PloS One. 2008;3:e1856.
- Li X, Dai J. Bridging the gap with functional collagen scaffolds: tuning endogenous neural stem cells for severe spinal cord injury repair. Biomater Sci. 2018;6:265–271.
- Yang Z, Zhang A, Duan H, et al. NT3-chitosan elicits robust endogenous neurogenesis to enable functional recovery after spinal cord injury. Proc Natl Acad Sci USA. 2015;112:13354–13359.
- Kim HM, Lee HJ, Lee MY, et al. Organotypic spinal cord slice culture to study neural stem/progenitor cell microenvironment in the injured spinal cord. Exp Neurobiol. 2010;19:106–113.
- Li X, Zhao Y, Cheng S, et al. Cetuximab modified collagen scaffold directs neurogenesis of injury-activated endogenous neural stem cells for acute spinal cord injury repair. Biomaterials. 2017;137:73–86.
- Wang J, Wang J, Lu P, et al. Local delivery of FTY720 in PCL membrane improves SCI functional recovery by reducing reactive astrogliosis. Biomaterials. 2015;62:76–87.
- Fan C, Li X, Xiao Z, et al. A modified collagen scaffold facilitates endogenous neurogenesis for acute spinal cord injury repair. Acta Biomaterialia. 2017;51:304–316.
- Du B-l, Zeng C-g, Zhang W, et al. A comparative study of gelatin sponge scaffolds and PLGA scaffolds transplanted to completely transected spinal cord of rat. J Biomed Mater Res. 2014;102:1715–1725.
- Chan EWC, Bennet D, Baek P, et al. Electrospun polythiophene phenylenes for tissue engineering. Biomacromolecules. 2018;19:1456–1468.
- Zhao B, Zheng Z. Insulin growth factor 1 protects neural stem cells against apoptosis induced by hypoxia through Akt/mitogen-activated protein kinase/extracellular signal-regulated kinase (Akt/MAPK/ERK) pathway in hypoxia-ishchemic encephalopathy. Med Sci Monit. 2017;23:1872–1879.
- Hu Q, Lee SY, O'Kusky JR, et al. Signalling through the type 1 insulin-like growth factor receptor (IGF1R) interacts with canonical Wnt signalling to promote neural proliferation in developing brain. ASN Neuro. 2012;4(5):e00092.
- Lunn JS, Sakowski SA, McGinley LM, et al. Autocrine production of IGF-I increases stem cell-mediated neuroprotection. Stem Cells. 2015;33:1480–1489.
- Liu W, D'Ercole JA, Ye P. Blunting type 1 insulin-like growth factor receptor expression exacerbates neuronal apoptosis following hypoxic/ischemic injury. BMC Neurosci. 2011;12:64.
- Carlson SW, Madathil SK, Sama DM, et al. Conditional overexpression of insulin-like growth factor-1 enhances hippocampal neurogenesis and restores immature neuron dendritic processes after traumatic brain injury. J Neuropathol Exp Neurol. 2014;73:734–746.
- Hurtado-Chong A, Yusta-Boyo MJ, Vergaño-Vera E, et al. IGF-I promotes neuronal migration and positioning in the olfactory bulb and the exit of neuroblasts from the subventricular zone. Eur J Neurosci. 2009;30:742–755.
- Ye P, Popken GJ, Kemper A, et al. Astrocyte-specific overexpression of insulin-like growth factor-I promotes brain overgrowth and glial fibrillary acidic protein expression. J Neurosci Res. 2004;78:472–484.
- Cao Y, Gunn AJ, Bennet L, et al. Insulin-like growth factor (IGF)-1 suppresses oligodendrocyte caspase-3 activation and increases glial proliferation after ischemia in near-term fetal sheep. J Cereb Blood Flow Metab. 2003;23:739–747.
- Islam O, Loo TX, Heese K. Brain-derived neurotrophic factor (BDNF) has proliferative effects on neural stem cells through the truncated TRK-B receptor, MAP kinase, AKT, and STAT-3 signaling pathways. CNR. 2009;6:42–53.
- Kirschenbaum B, Goldman SA. Brain-derived neurotrophic factor promotes the survival of neurons arising from the adult rat forebrain subependymal zone. Proc Natl Acad Sci USA. 1995;92:210–214.
- Jiang Z, et al. Enhanced migration of neural stem cells by microglia grown on a three-dimensional graphene scaffold. ACS Appl Mater Interfaces. 2016;8:25069–25077.
- Lai P-X, Chen C-W, Wei S-C, et al. Ultrastrong trapping of VEGF by graphene oxide: anti-angiogenesis application. Biomaterials. 2016;109:12–22.
- Fu C, Yang X, Tan S, et al. Enhancing cell proliferation and osteogenic differentiation of MC3T3-E1 pre-osteoblasts by BMP-2 delivery in graphene oxide-incorporated PLGA/HA biodegradable microcarriers. Sci Rep. 2017;7:12549.
- Garcia-Alegria E, Iluit M, Stefanska M, et al. Corrigendum: graphene oxide promotes embryonic stem cell differentiation to haematopoietic lineage. Sci Rep. 2016;6:28723.
- Shah S, Yin PT, Uehara TM, et al. Guiding stem cell differentiation into oligodendrocytes using graphene-nanofiber hybrid scaffolds. Adv Mater. 2014;26:3673–3680.
- Defterali C, Verdejo R, Peponi L, et al. Thermally reduced graphene is a permissive material for neurons and astrocytes and de novo neurogenesis in the adult olfactory bulb in vivo. Biomaterials. 2016;82:84–93.
- Solanki A, Chueng S-TD, Yin PT, et al. Axonal alignment and enhanced neuronal differentiation of neural stem cells on graphene-nanoparticle hybrid structures. Adv Mater. 2013;25:5477–5482.
- Farrell K, Joshi J, Kothapalli CR. Injectable uncrosslinked biomimetic hydrogels as candidate scaffolds for neural stem cell delivery. J Biomed Mater Res. 2017;105:790–805.
- Patila M, Pavlidis IV, Kouloumpis A, et al. Graphene oxide derivatives with variable alkyl chain length and terminal functional groups as supports for stabilization of cytochrome C. Int J Biol Macromol. 2016;84:227–235.
- Shen H, et al. PEGylated graphene oxide-mediated protein delivery for cell function regulation. ACS Appl Mater Interfaces. 2012;4:6317–6323.
- Lee WC, Lim CHYX, Shi H, et al. Origin of enhanced stem cell growth and differentiation on graphene and graphene oxide. ACS Nano. 2011;5:7334–7341.
- Quarles LD, Yohay DA, Lever LW, et al. Distinct proliferative and differentiated stages of murine MC3T3-E1 cells in culture: an in vitro model of osteoblast development. J Bone Miner Res. 2009;7:683–692.
- Wilson CJ, Clegg RE, Leavesley DI, et al. Mediation of biomaterial-cell interactions by adsorbed proteins: a review. Tissue Engineering. 2005;11:1–18.
- Chen SQ, Cai Q, Shen YY, et al. Combined use of NGF/BDNF/bFGF promotes proliferation and differentiation of neural stem cells in vitro. Int J Dev Neurosci. 2014;38:74–78.
- Liu F, Xuan A, Chen Y, et al. Combined effect of nerve growth factor and brainderived neurotrophic factor on neuronal differentiation of neural stem cells and the potential molecular mechanisms. Mol Med Rep. 2014;10:1739–1745.
- Huat TJ, Khan AA, Pati S, et al. IGF-1 enhances cell proliferation and survival during early differentiation of mesenchymal stem cells to neural progenitor-like cells. BMC Neurosci. 2014;15:91.
- Tator CH, Fehlings MG. Review of the secondary injury theory of acute spinal cord trauma with emphasis on vascular mechanisms. J Neurosurg. 1991;75:15–26.
- Anderson DK, Hall ED. Pathophysiology of spinal cord trauma. Ann Emerg Med. 1993;22:987–992.
- Hall ED. Antioxidant therapies for acute spinal cord injury. Neurotherapeutics. 2011;8:152–167.
- Hachem LD, Mothe AJ, Tator CH. Effect of BDNF and other potential survival factors in models of in vitro oxidative stress on adult spinal cord-derived neural stem/progenitor cells. Biores Open Access. 2015;4:146–159.
- Aburto MR, Magarinos M, Leon Y, et al. AKT signaling mediates IGF-I survival actions on otic neural progenitors. PloS One. 2012;7:e30790.
- Croci L, Barili V, Chia D, et al. Local insulin-like growth factor I expression is essential for Purkinje neuron survival at birth. Cell Death Differ. 2011;18:48–59.
- Yamada M, Tanabe K, Wada K, et al. Differences in survival-promoting effects and intracellular signaling properties of BDNF and IGF-1 in cultured cerebral cortical neurons. J Neurochem. 2001;78:940–951.
- Zheng WH, Quirion R. Comparative signaling pathways of insulin-like growth factor-1 and brain-derived neurotrophic factor in hippocampal neurons and the role of the PI3 kinase pathway in cell survival. J Neurochem. 2004;89:844–852.
- Liu X, Miller AL 2nd, Park S, et al. Functionalized carbon nanotube and graphene oxide embedded electrically conductive hydrogel synergistically stimulates nerve cell differentiation. ACS Appl Mater Interfaces. 2017;9:14677–14690.
- Fuhrmann T, Anandakumaran PN, Shoichet MS. Combinatorial therapies after spinal cord injury: how can biomaterials help? Adv Healthc Mater. 2017;6.
- Park SY, Park J, Sim SH, et al. Enhanced differentiation of human neural stem cells into neurons on graphene. Adv Mater. 2011;23:H263–H267.
- Yang D, Li T, Xu M, et al. Graphene oxide promotes the differentiation of mouse embryonic stem cells to dopamine neurons. Nanomedicine. 2014;9:2445–2455.
- Utada K, Ishida K, Tohyama S, et al. The combination of insulin-like growth factor 1 and erythropoietin protects against ischemic spinal cord injury in rabbits. J Anesth. 2015;29:741–748.
- Nakao Y, Otani H, Yamamura T, et al. Insulin-like growth factor 1 prevents neuronal cell death and paraplegia in the rabbit model of spinal cord ischemia. J Thorac Cardiovasc Surg. 2001;122:136–143.
- Keefe KM, Sheikh IS, Smith GM. Targeting neurotrophins to specific populations of neurons: NGF, BDNF, and NT-3 and their relevance for treatment of spinal cord injury. Int J Mol Sci. 2017;18:E548.
- Downing TL, Wang A, Yan Z-Q, et al. Drug-eluting microfibrous patches for the local delivery of rolipram in spinal cord repair. J Control Release. 2012;161:910–917.
- Wang C, Sun C, Hu Z, et al. Improved neural regeneration with olfactory ensheathing cell inoculated PLGA scaffolds in spinal cord injury adult rats. Neuro-Signals. 2017;25:1–14.
- Sensharma P, Madhumathi G, Jayant RD, et al. Biomaterials and cells for neural tissue engineering: CURRENT choices. Mater Sci Eng C. 2017;77:1302–1315.
- Sykova E, Jendelova P, Urdzikova L, et al. Bone marrow stem cells and polymer hydrogels–two strategies for spinal cord injury repair. Cell Mol Neurobiol. 2006;26:1113–1129.
- Magaki SD, Williams CK, Vinters HV. Glial function (and dysfunction) in the normal and ischemic brain. Neuropharmacology. 2018;134:218–225.
- Lopez-Dolado E, et al. Immunomodulatory and angiogenic responses induced by graphene oxide scaffolds in chronic spinal hemisected rats. Biomaterials. 2016;99:72–81.
- Palejwala AH, et al. Biocompatibility of reduced graphene oxide nanoscaffolds following acute spinal cord injury in rats. Surg Neurol Int. 2016;7:75.