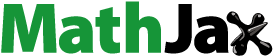
Abstract
Mannosylation of nanovaccine is an appropriate strategy for targeting the mannose receptors on DCs. Here, HBsAg and mannose loaded on the surface of iron oxide nanoparticles to increases HBsAg vaccine potency. Nanoparticles are made by co-precipitation method and bonded to the HBsAg and mannose by chemical bonding. The physicochemical properties of nano-vaccines, their toxicity and antigenicity were determined. The synthesized nano-vaccine showed spherical shape with a mean particle size of 60 nm, a zeta potential of −44 mV, an antigen-binding efficiency of around 100% and for mannose 78%. In vitro release of nanoparticles exhibited about 30% at the first day and about 60% until the third day. SDSPAGE analysis confirmed structural integrity of HBsAg loaded on nanoparticles. The HBsAg-loaded LCMNP and MLCMNP nanoparticles had no toxic effects on HEK293 cell line. The quantification of the intracellular Fe by ICP-OES as a criterion of nano-vaccine uptake revealed mannose intensify uptake of MLCMNP. In addition, mannose in the structure of MLCMNP improved IL-6, TNF-α and IFN-γ (>16 fold) cytokines genes expression by macrophage/dendritic cells after exposure in 12 h. Immunization of experimental mice (subcutaneously, two times with 2-week intervals) with 5 µg of HBsAg loaded on MLCMNP nanoparticles increased specific total IgG and IgG2a/IgG1 ratio. In addition, TNF-α, IL-12, IL-2 and IL-4 cytokines in mannosylated nano-vaccine increased versus nano-vaccine group while lymphocyte proliferation and IFN-γ responses in the targeted nano-vaccine group show a tiny increase versus the nano-vaccine group. The results show that mannosylated nano-vaccine promotes higher level of cellular and humoural immune responses against HBsAg nano-vaccine.
Introduction
Hepatitis B virus (HBV) infection is one of the most serious chronic viral infections around the world. It is estimated that more than 350 million people are chronic carriers and about which 5% develop cirrhosis and hepatocellular carcinoma. In some cases, HBV causes acute infection leading to acute liver failure [Citation1,Citation2]. Vaccination is the most effective way to prevent hepatitis B infection. However, 1–10% of vaccinated individuals fail to produce enough anti-HBs after three or more times of vaccinations and are considered to be non-responders. Risk factors such as smoking, increasing age, immunosuppressive disease, male sex, infections, obesity and Genetic factors could cause a non-response to the HBV vaccination [Citation3,Citation4]. Various methods have been investigated to increase the vaccine potency [Citation5]. One of the strategies to improve the vaccines immunogenicity is targeting nano-sized materials through carrying different ligands versus receptors on antigen presenting cells (APCs), such as macrophages and dendritic cells (DCs). Among all ligands, mannose sugar has attracted attentions [Citation6,Citation7]. Absorption of antigen by mannose receptor allows processing and presentation of antigen through the MHC class I and II pathways [Citation8,Citation9]. In this regard, mannosylation of several antigen delivery vehicles, such as liposomes [Citation10], nanoparticles, nano polymers [Citation5,Citation11] and inorganic nanoparticles such as Ag, Au, SiO2, C and Fe3O4 as adjuvants [Citation12–16], increases more efficient and specific delivery of antigens to DCs. Magnetic nanoparticles have been recently considered by researchers due to their unique properties (low toxicity, low cost of production, ability to immobilize biological materials on their surfaces) [Citation17], high applications in medicine and also their use in vaccines [Citation18]. Many types of iron oxide nanoparticles have been approved for clinical use including contrast agents for magnetic resonance imaging (MRI), iron-deficiency treatment and drug delivery systems by the United States Food and Drug Administration (FDA) [Citation18,Citation19]. Some other bio-applications of these nanoparticles consist targeted delivery of drugs [Citation20], hyperthermia [Citation21], bacteria detection [Citation22] and enzyme immobilization carriers [Citation23]. In addition, nanoparticles as delivery system can usually deliver antigen to immune system or act as immune potentiator to enhance antigen processing [Citation24]. To prevent agglomeration and oxidization of magnetic nanoparticles, after synthesis, they can be coated with monolayer ligands, polymers, combinations of polymers and biomolecules (phospholipids and carbohydrates) and inorganic materials (silica and gold) [Citation25]. Not only these coatings provide stability to nanoparticles in solution but also they help binding several biological ligands (antibodies, proteins, transferring, folic acid) needed for medical applications on the nanoparticle surface. These ligands can be attached to the polymer surfaces coating the magnetic nanoparticles by chemical coupling to make the particles target-specific [Citation25]. Here, we hypothesized that may be chemical adsorption of mannose and HBsAg on the surface of iron oxide nanoparticle, by targeting of immune cells result in increase of vaccine potency. So, after vaccine development and characterization, cellular and humoural immune responses were assessed in the experimental mice and showed positive effect of immuno-targeting by mannose in the improvement of various aspects of immune responses.
Materials and materials
Materials
FeCl2·4H2O, FeCl3·6H2O, pure ethanol, ammonium hydroxide (25%), acetone, tri-sodium citrate, l-cysteine, triethoxyvinylsilane and azobisisobutyronitrile (AIBN) were purchased from Merck (Kenilworth, NJ). Tetraethyl orthosilicate (TEOS), N-(3-dimethylaminopropyl)-N′-ethylcarbodiimide hydrochloride (EDC), N-hydroxysuccinimide (NHS), chloroform and dimethyl sulphoxide (DMSO) were purchased from Sigma-Aldrich (St. Louis, MO). Primers for real-time PCR (SinaClon, Karaj, Iran), RNA extraction kits (Qiagen, Germantown, MD) and cDNA synthesis kits (Fermentas, Vilnius, LA) prepared for cytokines genes expression assay. Commercial HBV vaccine and HBsAg were supplied by Pasteur Institute of Iran. Other materials, buffer solution, distilled deionized water and cytokine kits (Mabtech, Nacka Strand, Sweden) were prepared commercially.
Preparation of Fe3O4 magnetic nanoparticles (MNPs)
Iron oxide nanoparticles were prepared via co-precipitation of ferrous and ferric ions in the presence of ammonium hydroxide and sodium citrate using a procedure in the literature [Citation26]. In a three-necked flask, a mixture of FeCl2.4H2O (10 mmol, 2 g) and FeCl3.6H2O (20 mmol, 5.4 g) was dissolved in 120 mL of deionized H2O. Then 10 mL ammonium hydroxide (25%) was added quickly under rapid mechanical stirring (600 rpm) at 60 °C. The mixture was stirred in this condition for 1 h. The nanoparticles were isolated from the solution by magnetic separation and dissolved in 200 mL tri-sodium citrate solution (0.3 molar). Later, the mixture was stirred for 1 h under a mechanical stirrer at 80 °C. It should be noted that all these steps were carried out in the presence of N2 gas. Finally, the Fe3O4 magnetic nanoparticles were separated by a magnet and were washed with acetone to remove extra tri-sodium citrate and dried at 60 °C for 24 h.
Preparation of silica-coated MNPs
Iron oxide nanoparticles were coated by silica layer using tetraethyl orthosilicate (TEOS) described in the previous study [Citation26,Citation27] with some modifications. For this purpose, 2 g of Fe3O4 magnetic nanoparticles from previous step was added to 100 mL deionized water and then the solution was sonicated for 1 h. The sonicated solution with 280 mL of ethanol and 10 mL of ammonia were poured into the flask. The mixture was stirred by mechanical stirrer at 48 °C. Then 2 mL TEOS in 20 mL ethanol was added drop-wise to the mixture. The solution was stirred for 6 h at 48 °C and Silica-coated magnetic nanoparticles were washed three times with ethanol and collected by magnet. Eventually, the product was dried under vacuum at 40 °C for 2 h.
Preparation of l-cysteine magnetic nanoparticles (LCMNP)
Iron oxide nanoparticles were functionalized with l-cysteine according to the reported literature procedure [Citation28]. About 1.8 g final product of the previous step was added to 14 mL chloroform. Then, 1 mL trimethoxy (vinyl) saline (0.02 mol) was added drop-wise to the solution at room temperature. The solution was stirred for 12 h at the refluxing temperature of chloroform. Then vinyl magnetic nanoparticles were dried in a vacuum at 50 °C. After that 2 g of this product was added to 13 mL chloroform. Next 0.42 g of l-cysteine was added to prepared solution in the presence of AIBN(1 mmol). The solution was stirred for 12 h at the refluxing temperature of chloroform. l-Cysteine magnetic nanoparticles washed with water and separated with magnet and dried in vacuum at 50 °C.
Conjugation of mannose to the surface of LCMNP (MLCMNP)
Attachment of mannose on the surface of the LCMNP is done by chemical reaction as previously reported [Citation29] with some modification. In this method, 100 mg of LCMNP dispersed in 10 mL acidic DMSO solution (pH 5) then 0.1 g N-(3-dimethylaminopropyl)-N′-ethylcarbodiimide hydrochloride (EDC) and 0.15 g N hydroxysuccinimide (NHS) was added to the solution, after that 3 mg mannose was added. The solution was then stirred at room temperature overnight. The excess reagents and DMSO were then removed from product with magnet and wash with distilled deionized water three times and dried in a vacuum oven. The MLCMNP powder was analyzed by phenol–sulphuric acid method, Fourier-transform infrared spectroscopy (FTIR), X-ray powder diffraction (XRD) and X-ray analysis (EDX).
Conjugation of hepatitis B surface antigen to the surface of MLCMNP
The HBsAg-MLCMNP conjugates were prepared using EDC and NHS covalent coupling chemistry. For this purpose, 50 mg MLCMNP (5 mL of high solution before drying) with carboxyl and amine groups on its surface were activated by incubating with 0.05 g EDC and 0.1 g NHS for 30 min in distilled deionized water (pH = 6), after which (375 μg) of HBsAg was added and the final volume of the solution was adjusted to 5 mL. Then shacked thoroughly, and incubated for 3 h at room temperature. After that for further tests, it was stored at 4 °C.
Characterization, shape and surface morphology of nanoparticles
The morphology and size of nanoparticles were studied by Field Emission Scanning Electron Microscopy (FESEM) (MIRA III, Tescan Company, Czech Republic). In order to confirm the successful attachment of the mannose and HBsAg on to nanoparticles, dynamic light scattering (DLS) using a Zeta sizer Nano range system (Malvern ZEN3600, Worcestershire, UK) was used to measure the respective zeta potential of nanoparticles. To determine the presence of mannose on the surface of nanoparticles, FTIR, EDX and XRD was done. FTIR spectra of MLCMNP were recorded and analyzed in the wave number 400–4000 cm−1 (AVATAR, Thermo Company, Waltham, MA). X-ray powder diffraction (XRD) was obtained by using PHILLIPS PW1730 X-ray diffractometer with Cu Kα radiation (40 kV and 30 mA), and the samples were scanned in the Bragg angle, 2θ range of 5–80°. The element composition form X-ray analysis (EDX) spectrum was determined.
Evaluation loading of HBsAg on iron oxide nanoparticles
To determine loading of HBsAg, the nanoparticles were separated by external magnet. The amount of free HBsAg in the supernatant was determined by Bradford protein assay [Citation30,Citation31]. The total amount of HBsAg (μg/ml) in supernatant was compared with that of standards and their absorption was read at 595 nm using a spectrophotometer and the quantity was calculated utilizing the equation: [(Ttotal−Tsupernatant)/Ttotal] ×100.
Evaluation loading of mannose on iron oxide nanoparticles
To quantify the amount of the mannose conjugated to the nanoparticles, phenol–sulphuric acid method was used [Citation32,Citation33]. To obtain the absorption standards and supernatant of samples using the wavelength of 490 nm, a microplate reader was used. The total amount of mannose in the supernatant was compared with that of standards and this amount was calculated utilizing the equation: [(Ttotal−Tsupernatant)/Ttotal] ×100.
In vitro release study
Antigen release from iron oxide nanoparticles was determined as follows: 10 mg of nano-vaccines and 10 mL of distilled deionized water (pH = 7.4) containing thimerosal (0.01%) was transferred to tubes and shaker incubator (37 °C and 100 rpm). One sample at scheduled times of 0, 1, 3, 7, 14, 21, 28 and 35 days was picked up and separated by external magnet, then the amount of released HBsAg was obtained using Bradford assay.
Toxicity of nano-vaccines for HEK293 cell line
In vitro cytotoxicity of MLCMNP–HBsAg nanoparticles and LCMNP–HBsAg nanoparticles against HEK293 cell line (human embryonic kidney cells 293) over 24 h with various concentrations were assessed [Citation18,Citation34]. The cells were seeded in 96-well plates at the density of 2 × 104cells/well in DMEM media supplemented with 10% FBS, 1% Pen/Strep, 2 mM l-glutamine, 1 mM non-essential amino acid and 1 mM sodium pyruvate. The cells were treated with different concentrations of MLCMNP-HBsAg and LCMNP-HBsAg (200, 100, 50, 25, 12.5, 6.25, 3.1, 1.55 and 0.775 μg/ml of HBs Ag loaded to the surface of nanoparticles) and incubated at 37 °C in 5% CO2 incubator. After 24 h incubation times, 20 μL of MTT solution (3–(4,5-dimethylthiazol-2yl)-2,5-diphenyltetrazolium bromide) (5 mg/mL) was added to each well and the cells were incubated for another 4 h in dark. The medium removed and 100 μL of DMSO added and plate was incubated for 5 min at room temperature. The absorbance at 570 nm was measured by ELISA plate reader. Un-treated cells were used as the control and were considered as 100% cell viability. Cell viability was calculated with the following formula:
Characterization of structural integrity of HBsAg
Stability and integrity of the entrapped antigen in nanoparticles were characterized by sodium dodecyl sulphate-polyacrylamide gel electrophoresis (SDS–PAGE) on 12% gel. The samples were run including 5 µg, 10 µg and 20 µg HBsAg, protein markers, MNP + HBsAg supernatant, MNP + HBsAg precipitate, MNP + HBsAg + mannose supernatant and MNP + HBsAg + mannose precipitate which examined for HBsAg and the gel was stained with Coomassie staining method to detect the bands [Citation35].
Macrophage uptake experiments of nanoparticles by inductively coupled plasma (ICP)
In order to study the potency of macrophage in the uptake of different formulations of nanovaccine, 5 × 105 J774 cells in complete DMEM medium were plated in culture dish with 200 μg/mL of MNP, MNP + mannose, MNP + HBsAg and MNP + mannose + HBsAg and also mere cells were used as control. The culture dishes incubated at an incubator with 5% CO2 and 37 °C for 24 h to allow uptake of different formulations of vaccine in this condition. After incubation time, the cell in each plate harvested with trypsin method and mg/l of iron uptake in each formulation assessed by inductively coupled plasma optical emission spectroscopy (ICP-OES). The iron uptake capacity was calculated by dividing iron of cell exposed to nano-vaccines/iron of mere cells and considered as Relative Uptake for each nano-vaccine formulation.
IFN-γ, TNF-α, TGF-β, IL-10 and IL-6 cytokines genes expression by macrophage/dendritic cells after uptake of nano-vaccines
Heparinized peripheral blood samples were obtained from three healthy volunteers. The samples diluted ½ with PBS and loaded on Ficoll-Hypaque density gradient and after 20 mins’ centrifugation at 4000 rpm at the room temperature, the buffy coat layer was isolated and washed twice with cold PBS. The cells counted with trypan blue and resuspended in complete RPMI-1640 culture medium (RPMI-1640, foetal bovine serum 10%, l-glutamine 2 mM, penicillin 100 U/mL and streptomycin 100 µg/mL). The cell suspension adjusted to 2 × 107 cell/mL and 1 mL of cell suspension added to culture dishes and the dishes incubated for 2 h at 37 °C with 5% CO2 to attach monocytes and DCs. After this time, non-adherent cells discarded and thoroughly rinse the dishes three times to eliminate all non-adherent cells. The attached cells considered as macrophage/dendritic cells and stimulated with different formulations of nano-vaccines (MNP, MNP + mannose, MNP + HBsAg, MNP + mannose + HBsAg) at a concentration of 1 µg/mL of HBsAg (1 µg/mL is based on HBsAg content but based on nanoparticles for each experimental vaccine formulation is 200 µg/mL) for 12 h. In addition, mere cells were considered as non-treatment control and LPS-stimulated cells (500 ng/mL) were considered as positive control.
After in vitro stimulation, gene expression of cytokines of IFNγ, TNFα, TGFβ, IL-10 and IL-6 was analyzed using real-time PCR. In brief, RNA of stimulated cells was extracted at 3, 6 and 12 h post stimulation using an RNA extraction kit (Qiagen, Germantown, MD) and reverse transcribed into cDNA using Revert AidTM First Strand cDNA Synthesis Kit (Fermentas, Vilnius, LA); the reaction mixture contained 4 µL of the 5× reaction buffer, 1 µg of the extracted RNA, 1 µL of a random hexamer primer, 1 µL of the deoxynucleotide triphosphate mixture (10 mM), 1 µL of RNase enzyme inhibitor (20 units/µL), 1 µLof reverse transcriptase enzyme and double-distilled water (up to a final volume of 20 µL). The temperature program was set as follows: 25 °C for 5 min (for primer annealing); 42 °C for 1 h; and 75 °C for 5 min. The primers sequence of the target genes, including IFNγ, TNFα, TGFβ, IL-10, IL-6 and β-actin (internal control), is given in .
Table 1. The primer sequences of target genes.
Finally, the real-time PCR reaction was performed using a real-time PCR System (Applied Biosystems, Foster City, CA, Step One) at a temperature program as follows: 95 °C for 1 min; 95 °C for 20 s; and 55 °C for 60 s. Primers for TNFα, TGF-β, IL-6, IFN-γ and IL-10 were used at 10 nM. Analysis of gene expression was performed by the ΔΔCT method [Citation40]. Briefly, each sample was normalized to an endogenous control, β-actin, and fold change for each gene was determined via the Ct.
Experimental groups and immunization
Female BALB/c mice (6–8 weeks old, n = 51, purchased from Pasteur Institute of Karaj, Iran) were divided into seven groups (). The mice were kept for 7 d before beginning of the experiments with access to food and water observing standard conditions including equal light/darkness and temperature ranging between 20 and 22 °C. All experiments on animals carried out according to the Animal Care Protocol of Pasteur Institute of Iran. In order to vaccination, groups 1 and 2 were administrated with 5 µg of HBsAg formulated in MLCMNP-HBsAg and LCMNP-HBsAg, respectively, while group 3 received commercial HBsAg vaccine. Group 4 received HBsAg alone. The experimental mice received two doses of injection with 2 week intervals on days 0 and 14 by subcutaneous route. Also, controls groups for MNP, MNP-mannose and PBS were considered.
Table 2. Experimental groups with vaccine formulations.
ELISA of specific total IgG versus HBsAg and IgG2a/IgG1 ratio
Specific total IgG antibodies were evaluated using an optimized indirect ELISA method 7 days after final immunization. Briefly, 100 µL of HBsAg (5 µg/mL) in PBS (pH = 7.2–7.4) were coated in 96-well ELISA Maxisorp plates (Greiner, Pleidelsheim, Germany) followed by overnight incubation at 4 °C. The wells were washed three times with PBS containing 0.05% Tween 20 (washing buffer) and blocked for 1 h at 37 °C with 2% skimmed milk in PBS buffer (blocking buffer). Serial dilutions of sera in order of 1/10–1/1280 were prepared in 0.5% PBS-skimmed milk. After washing the wells, 100 µL of each dilution was added to each well in duplicate and incubated at 37 °C for 90 min. Then the wells were washed five times with washing buffer and incubated for 90 min at 37 °C. After incubation, the wells were washed five times with washing buffer and 100 µL of 1/10,000 dilution of anti-mouse conjugated to horseradish peroxidase Horseradish Peroxidase (HRP) (Sigma, St. Louis, MO) in phosphate-buffered saline with 0.5% skimmed milk added and incubated for 90 min at 37 °C. Later, the wells were washed five times with washing buffer and 100 µL of TMB substrate was added, and incubated for 30 min in the dark. The reaction was stopped using 100 μL of 2 N H2SO4 and colour density was measured at A450/630 nm with ELISA plate reader (AWARENESS, Portsmouth, VA). In addition, specific IgG1 and IgG2a isotypes were assessed by ELISA using specific anti-mouse IgG1 and anti-mouse IgG2a HRP-conjugate (Sigma, St. Louis, MO) and IgG2a/IgG1 ratio calculated for individual mice using their row data.
Lymphocyte proliferation assay
Two weeks after the final immunization, the spleens of experimental mice were dissected and suspended in the sterile cold PBS containing 2% FBS. RBCs were lysed with lysis buffer and suspension was centrifuged at 300g/5 min at 4 °C. The cell pellet was resuspended in RPMI-1640 supplemented with 10% FBS, 4 mM l-glutamine, 1 mM sodium pyruvate, 1 mM non-essential amino acid, 50 µm 2ME, 100 µg/mL streptomycin and 100 IU/mL penicillin. Then 100 µL of the cell suspension containing 4 × 105 cells was dispensed into 96-well-flat-bottom culture plates and antigen recall carried out using 5 µg/mL of HBsAg protein. Un-stimulated wells were used as negative control, Concanavalin A stimulated wells as positive control and culture medium considered as blank. After 72 h of antigen recall, 20 µL BrdU labelling solution was added into each well and culture continued for 18 h. The plates were then centrifuged at 300g/10 min and supernatant were removed and the plates were dried at 60 °C for 30 min. The cells were permeabilized by adding 200 µL of fix/denaturation buffer and then 100 µL of anti-BrdU antibody was added to each well for 90 min. After six times washing the plates with PBS, tetra methyl benzidine (TMB) solution was added to each well as a substrate and the reaction stopped by adding 100 µL of 2 N H2SO4. Absorbance was measured using a spectrophotometric plate reader (AWARENESS, Portsmouth, VA) at 450 nm.
IL-2, IL-4, IL-12, TNF-α and IFN-γ cytokines assay of experimental mice
Two weeks after the last immunization, the total number of 4 × 106 spleen cells/ml which were re-suspended in complete RPMI-1640, put in each well of 24-well plate and stimulated with 5 μg/mL of HBsAg for 72 h at 37 °C in 5% CO2. The supernatants were then collected and assessed for IFN-γ, TNF-α, IL-2, IL-4 and IL-12 cytokines by using commercial ELISA Kits (Mabtech, Nacka Strand, Sweden) according to the manufacturer’s manual. The quantity of each cytokine calculated according to its standard curve and reported as pg/ml.
Statistical analysis
All the data were presented as mean ± standard deviation (SD). The statistical significance of differences was analyzed using two-way ANOVA (Graph Pad Prism 6.01 software, GraphPad Software, La Jolla, CA). p Values of <.05 were considered as significant differences.
Results
Preparation and characterization of MLCMNP
The novel conjugates of LCMNP-mannose were synthesized and then characterized by FTIR () and XRD (). The FTIR and XRD results confirmed the conjugation of silica, l-cysteine linker and mannose to MNP nanoparticles. Shape and morphology of LCMNP, MLCMNP and MLCMNP-rHBsAg nanoparticles were evaluated using SEM (). Nanoparticles were found to be monodisperse and nearly spherical in shape. In addition, VSM analysis was performed to investigate the magnetic properties of nanoparticles. The ζ-potential measurement using QELS was used to determine the surface properties of nanoparticles (). The present results demonstrated that, the zeta potential of LCMNP, MLCMNP, MLCMNP-HBsAg and LCMNP-HBsAg were −79.15 ± 0.21 mV, −22.85 ± 0.21 mV, −44.5 ± 0.57 mV and −54.4 ± 0.71 mV, respectively. The results suggested that the conjugation of mannose and HBsAg led to an increase in the zeta potential of the nanoparticles.
Figure 1. FTIR spectra MNP nanoparticles (a), MNP–silica-coated nanoparticles (b), LCMNP (c), MLCMNP (d), l-cysteine (e) and mannose (f).
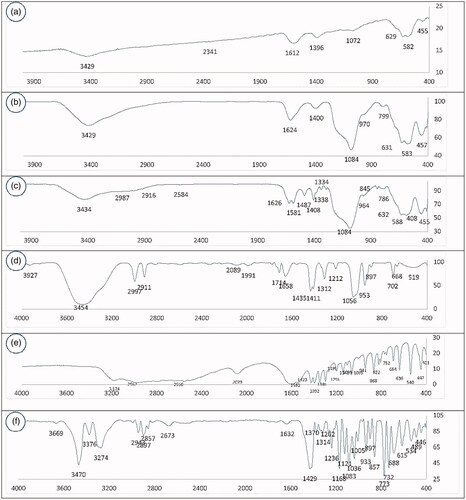
Table 3. Nanoparticles characterization.
Efficiency of mannose and antigen binding to the nanoparticles
The results showed that magnetic nanoparticles had an antigen-binding efficiency of around 100% while they had mannose-binding efficiency of around 78%.
In vitro antigen release
Antigen-release from the nanoparticles is shown in . MLCMNP-HBsAg showed the same release pattern as LCMNP-HBsAg. The release profile exhibited an initial fast release about 30% in the first day and about 60% until the third day, followed by a slow release of 15% for 35 days.
Structural integrity of HBsAg
In this study, SDS-PAGE analysis of HBsAg was exhibited bonds at position related to HBsAg (∼24 kDa) at the lanes related to MNP + HBsAg precipitate and MNP + HBsAg + mannose precipitate () and confirms the attachment of HBsAg to the surface of MNP nanoparticles.
Cell viability study
Cytotoxicity assay was investigated on HEK293 cell line as a sensitive cell for evaluating the toxicity of nanoparticles [Citation5,Citation34]. The experiment had no signs of toxicity, even at high concentrations of HBsAg in nano-vaccine formulations ().
Quantification of nano-vaccines uptake
The quantification of the intracellular fraction of elemental Fe by ICP-OES as a criterion of nano-vaccine uptake revealed an uptake of the nano-particles in all experiment groups over the 24 h. ICP-OES results () that remarkably more iron content was present in cells incubated with MLCMNP + HBsAg than in cells incubated with other groups. Our work shows the crucial role of mannose in enhancing the endocytic uptake of nano-vaccine.
Nano-vaccines uptake induced pro-inflammatory cytokines production by macrophage/dendritic cells
Macrophage/dendritic cells isolated from peripheral blood of healthy volunteers exposed to different nano-vaccines over a 12 h period, and the expression of IL-6, IL-10, TNF-α, IFN-γ and TGF-β cytokine genes was monitored by real time-PCR. Results show that LCMNP + HBsAg nanoparticles increased the production of IL-6, TNF-α and IFN-γ by >5-fold as compared with β-actin (control) at 12 h (). While MLCMNP + HBsAg nanoparticles increased the expression of IL-6, TNF-α and IFN-γ (>16 fold) as compared with β-actin (control) at 12 h (). Our results showed that the expression of cytokines in mere nanoparticles group and in nanoparticles with mannose without antigen was very low, but the expression of IL-6, TNF-α and IFN-γ was negligibly increased compared with the beta actin (). LPS-stimulated cells induced high-level expression of all inflammatory cytokines assayed, with the exception of TGF-β and IL-10 versus to β-actin (control) ().
Figure 8. Real-time-PCR quantification of IFN-γ, TNF-α, TGF-β, IL-10 and IL-6 cytokine gene expressions in macrophage/dendritic cells MNP (A), MNP + mannose-stimulated cells (B), MNP + HBsAg-stimulated cells (C), MNP + HBsAg + mannose-stimulated cells (D), and LPS-stimulated cells compared with β-actin gene (E). Expression was monitored over a 12-h period.
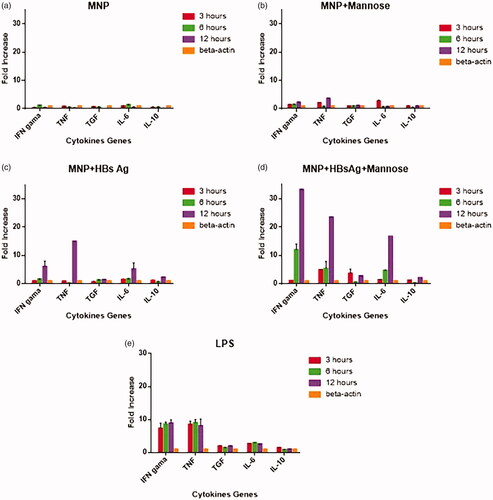
Results of total IgG and IgG2a/IgG1ratio
Results show that immunization with MLCMNP-HBsAg (at dilutions 1/10 up to 1/320), LCMNP-HBsAg (at dilutions1/10 up to 1/40) and commercial HBs Ag/Alum vaccine (at dilutions 1/10 up to 1/80) increased total IgG antibodies as compared with control groups (p < .0363) (). Immunization with MLCMNP-HBsAg showed a significant increase (at dilution 1/10) versus commercial vaccine (p = .0363). Also, results show that the addition of mannose in the MLCMNP-HBsAg group resulted in a significant increase (at dilutions 1/10 up to 1/320) versus the LCMNP-HBsAg group (p < .0363).
Figure 9. Specific total IgG responses after immunization course. Conjugation of nano-vaccine with mannose increased antibody responses versus nano-vaccine without mannose.
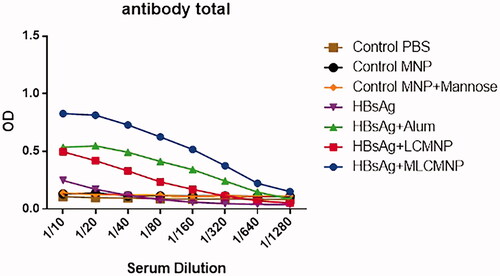
The results of IgG1 and IgG2a isotypes indicated that immunization with MLCMNP-HBsAg vaccine increased IgG2a/IgG1 ratio compared with LCMNP-HBsAg (p = .0032), commercial vaccine (p = .0003) and mere HBsAg group (p = .0051) ().
Results of lymphocyte proliferation
Results of lymphocyte proliferation show that immunization with MLCMNP-HBsAg and LCMNP-HBsAg increased lymphocyte proliferation compared with commercial vaccine, mere HBsAg and the control groups (p < .0001). Although immunization of mice using MLCMNP-HBsAg formulation increased the lymphocyte proliferation in comparison with the group that immunized with LCMNP-HBsAg (around 10% increase) but statistically was not significant (p = .9673) ().
Figure 11. Results of lymphocyte proliferation. Experimental mice were immunized with MLCMNP-HBsAg, LCMNP-HBsAg, commercial vaccine, HBs Ag alone and control mice were injected with MNP, MNP + mannose and PBS. Two weeks after last immunization, lymphocyte proliferation was assessed with BrdU method as mentioned in the method section. The results presented as mean ± SD of 10 mice per group. Immunization with MLCMNP-HBsAg and LCMNP-HBsAg significantly increased lymphocyte proliferation versus commercial vaccine, HBs Ag alone and control groups.
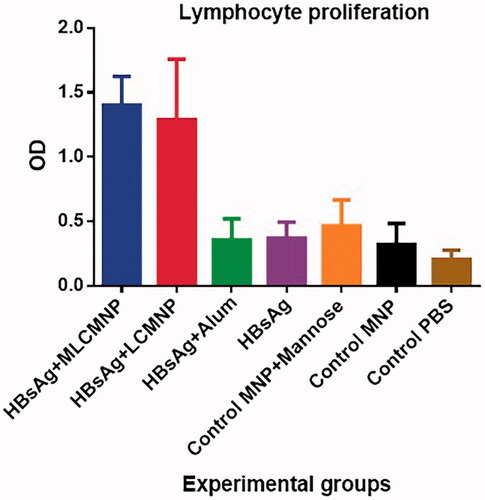
IFN-γ, TNF-α, IL-2, IL-4 and IL-12 cytokines of experimental mice
Results of IFN-γ cytokine show a significant increase in the vaccinated groups in comparison to all three control groups (p ≤ .0005). In addition, the results have no significant differences in IFN-γ cytokine response among MLCMNP-HBsAg, LCMNP-HBsAg, commercial HBsAg vaccine and mere HBsAg groups (p > .5203). However, adding mannose to the formulation of LCMNP-HBsAg increased IFN-γ response around 7% but statistically does not show significant difference versus LCMNP-HBsAg group ().
Figure 12. Results of IFN-γ cytokine assay. Mice were immunized two times with two weeks’ interval with MLCMNP-HBsAg, LCMNP-HBsAg formulation, commercial vaccine and HBs Ag alone along with proper control groups. Two weeks after last immunization of experimental mice, IFN-γ cytokine level was assessed using commercial quantitative ELISA kit. The results presented as mean ± SD of ten mice per group. Immunization with MLCMNP-HBsAg, LCMNP-HBsAg formulation, commercial vaccine and HBs Ag alone significantly increased IFN-γ cytokine versus control groups.
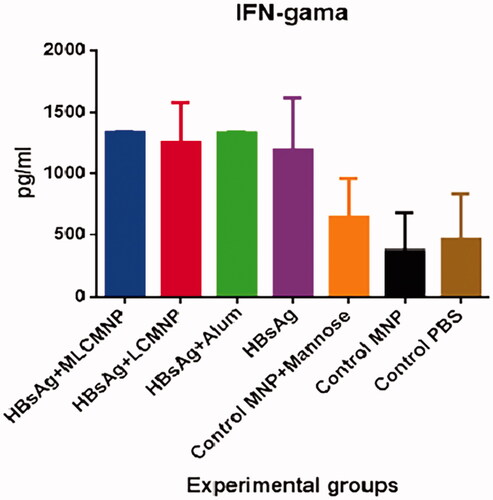
Results of TNF-α cytokine show that mice immunized with MLCMNP-HBsAg vaccine experienced a significant increase in comparison with mere HBsAg (p < .0001), LCMNP-HBsAg vaccine (p ≤ .0107) and all control groups (p ≤ .0007). But there was no a significant difference in TNF-α response between groups of mice that immunized with the MLCMNP-HBsAg vaccine and the commercial HBsAg vaccine group (p = .9) ().
Figure 13. Results of TNF-α cytokine assay. Data presented as mean ± SD of 10 mice per group. Immunization with MLCMNP-HBsAg significantly increased TNF-α cytokine versus the LCMNP-HBsAg group.
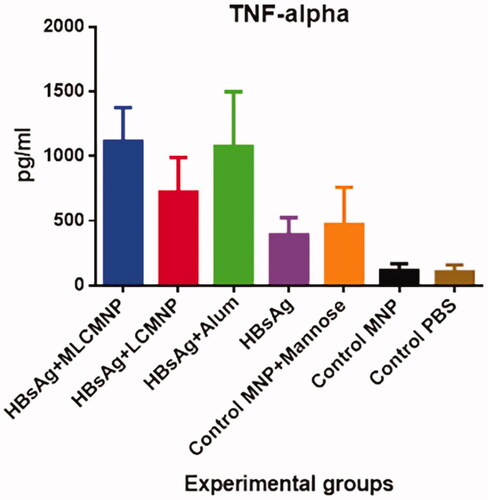
Results of IL-2 cytokine show that immunization with MLCMNP-HBsAg formulation increased IL-2 secretion as compared with control groups (p < .0032) and versus commercial HBsAg group (p = .0035) and mere HBsAg group (p = .0008). Also, the addition of mannose to the formulation of nano-vaccine in MLCMNP-HBsAg group shows a significant increase of IL-2 secretion versus the LCMNP-HBsAg group (p = .0002) ().
Figure 14. Results of IL-2 cytokine assay. After immunization course of experimental mice, IL-2 cytokine level was assessed with a quantitative commercial ELISA kit. Immunization of mice by MLCMNP-HBsAg formulation shows a significant increase of IL-2 cytokine production versus other experimental groups.
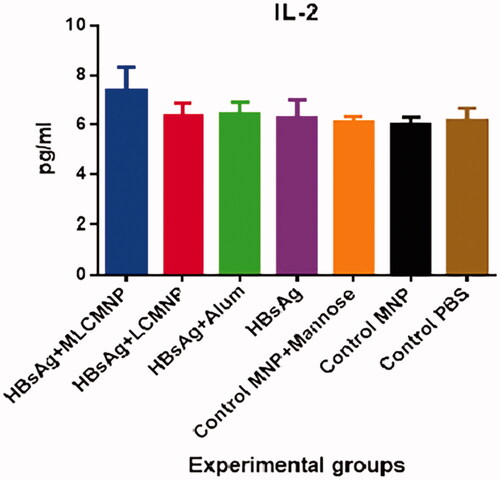
Result of IL-4 cytokine assay showed that immunization with MLCMNP-HBsAg vaccine increased IL-4 cytokine compared with control groups (p < .0001), commercial HBs Ag vaccine (p < .0001), mere HBsAg (p < .0001) and LCMNP-HBsAg vaccine (p = .0120) ().
Figure 15. Results of IL-4 cytokine assay. After immunization course of experimental mice, IL-4 cytokine level was assessed with a quantitative commercial ELISA kit. The results presented as mean ± SD of 10 mice per group. Immunization with MLCMNP-HBsAg, LCMNP-HBsAg formulation significantly increased IL-4 cytokine secretion versus commercial vaccine, HBs Ag alone and control groups.
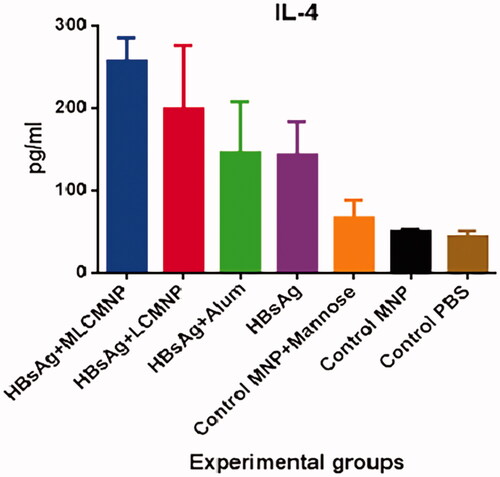
A study on IL-12 cytokine showed that injection of mice with MLCMNP-HBsAg vaccine remarkably increased level of the IL-12 cytokine in comparison with other experimental groups (p < .0133) ().
Discussion
An increase of vaccine potency is a hot topic in vaccinology. One strategy in this area is targeting of immune cells with ligand of their receptors. Dendritic cell act as a professional cell in the antigen presenting to T cells and is a vital player in the triggering of acquired immune responses against pathogens. So, many researchers targeted DCs via ligands of its receptors such as DEC205 and mannose in order to targeting delivery of antigens to this cell. Mannosylated nano-carriers capable of carrying various active agents, such as nucleic acids, peptides or proteins, are noticeable in various delivery systems [Citation11,Citation32,Citation41]. Magnetic iron oxide (IO) nanoparticles with long-term maintenance in blood and biodegradation and less toxicity have transpired as one of the primary nanomaterials for biomedical applications [Citation42]. Here, at first, MNP nanoparticles were synthesized, then coated with silica and finally were conjugated with mannose by l-cysteine linker and HBsAg for targeted delivery vaccine (). The LCMNP nanoparticles exhibited a ζ-potential of −79.15 ± 0.21 mV. In contrast, the MLCMNP, MLCMNP-HBsAg and LCMNP-HBsAg resulted in a more positive potential (−22.85 ± 0.21 mV, −44.5 ± 0.57 mV and −54.4 ± 0.71 mV, respectively) than LCMNP nanoparticles. This is due to the reaction between the carboxyl group of l-cysteine and the OH group of mannose, which, with the involvement of carboxyl groups, decreases the negative charge of the nanoparticle [Citation43]. HBsAg have made the nanoparticle burden a little more positive by reacting both with amine groups and with carboxylic acid groups on l -cysteine of nanoparticles. The zeta potential value can indicate the stability of the nanoparticles in the solution. Nanoparticles with a zeta potential greater than +30 mV or less than −30 mV exhibit high levels of stability. Therefore, in our study according to the past studies negative ζ-potential (−44.5 ± 0.57 mV, −54.4 ± 0.71 mV) increases the electrostatic repulsion and increases the dispersion of magnetic nanoparticles in water and reduces aggregation of magnetic nanoparticles [Citation44,Citation45].
In order to quantitatively determine successful HBsAg and mannose conjugation to the nanoparticle surface, the nano-vaccines analyzed by XPS [Citation46]. The surface composition of LCMNP, MLCMNP, MLCMNP-HBsAg and LCMNP-HBsAg was designated by energy dispersive X-ray spectroscopy as shown in . The presence of HBsAg and mannose is confirmed by the increase in carbon and oxygen atomic.
Table 4. X-ray photoelectron spectroscopy (XPS) analysis of atomic percentages of elements present on LCMNP, MLCMNP, MLCMNP-HBsAg and LCMNP-HBsAg.
Furthermore, indicates the FTIR spectra of the MNP nanoparticles and MNP-silica coated nanoparticles before and after l-cysteine linker and mannose sugar conjugation. The sharp and revealing peak at around 580–640 cm−6 can be observed in (a), (b), (c) and (d) spectra is relates to the absorption peak Fe–O–Fe bond of MNP nanoparticles. Silica presence on the surface of MNP nanoparticles is proven by the bands at 970 and 1084 cm−cm that dedicated to the Si–O stretching vibrations and the broad band at 3429 cm−c that is assigned to the O–H stretching vibration on the surface of Fe3O4 magnetic nanoparticles modified by TEOS. The peak intensity of 1624 is related to the stretching vibration C = C of the vinyl groups on the surface of the modified magnetic nanoparticles, while the stretching vibration of C = C–H in the region of 3050 cm−1 is observed in a short peak ().
Adsorption of l-cysteine linker on silica-coated MNPs resulted in the disappearance of the absorption band at 2079 cm−1, which has been removed by binding to the vinyl groups on the surface and in the case of S–C bonds, , characteristic to carbonyl stretching vibrations (C = 0 (in carboxylic groups and (N–H (amino groups in adsorbed l-cysteine linker, respectively, and appearance of them characteristic bands at 1626 and 1581 cm−1 (). The presence of the CH2 of l-cysteine linker was confirmed by C–H bending vibrations that appeared at 1408 cm−1.
Mannose is attached on LCMNP due to the presence of the hydroxyl group through the formation of an ester bond. The stretching vibration of the C = O– bonds appeared at 1658 cm−1. C–O stretching vibration of the hydroxyl groups of mannose as a broad peak in 1056 cm−1 was appeared and also, due to the increase in mannose hydroxyl groups, the stretching vibration of the O–H groups of hydroxyl groups at 3454 cm−1 appeared as a broad and strong peak ().
depicts the X-ray diffraction (XRD) patterns of LCMNP and MLCMNP. XRD patterns of the synthesized LCMNP (a) and MLCMNP (b) display relative diffraction peaks in the 2θ region of 5–80°. The six characteristic diffraction peaks in can be indexed to (220), (311), (400), (422), (511) and (440), which will agree with the database of magnetite in the Joint Committee on Powder Diffraction Standards [JCPDS] (JCPDS card: 19–629) file [Citation47–50]. Also, wide diffraction peaks at 19.2°can be viewed from LCMNP and MLCMNP which is a typical feature of amorphous SiO2 [Citation47]. After coating mannose, appeared broad XRD peak at a low diffraction angle of 10–30°, which is probably related to mannose binding to nanoparticles.
The magnetic properties of LCMNP, LCMNP-HBsAg and MLCMNP–HBsAg were measured by VSM at room temperature (). The magnetic saturation values are 43, 42 and 40 emu/g for LCMNP, MLCMNP–HBsAg and LCMNP-HBsAg. The decrease in the overall magnetization values indicates that the MNP surface is covered with non-magnetic materials such as mannose and HBsAg. Toxicity study of MLCMNP–HBsAg nano vaccine did not have any toxicity on sensitive HEK293 cell line. Our result is parallel to literatures that studied different concentration of iron oxide nanoparticles and HBsAg on sensitive cell lines [Citation51,Citation52].
The HBsAg release profile showed that maximum release was approximately 30% in the first 24 h of both MLCMNP–HBsAg and LCMNP–HBsAg nanoparticles, indicating that mannose attachment on the surface of nanovaccine did not interfere with the release of antigen. On the third day of antigen release test, about 60% release was observed. In comparison to the other nano-vaccines antigen release kinetic [Citation53], it seems that our nanovaccine releases the antigen rapidly and this property may affect the immune response pattern and acceleration. In the next, nano-vaccine uptake by macrophage/dendritic cells with ICP-OES method confirmed nano-vaccines uptake and adding of mannose to the nano-vaccine improved the uptake. This finding is parallel to other vaccine models that used mannose to target mannose receptor on macrophage/dendritic cells [Citation11]. The targeting of vaccine to mannose receptor not only guaranties efficient delivery of HBsAg to the immune cells but also the receiver cells efficiently activates through engagement of mannose receptor which trigger various signalling pathways [Citation11,Citation54]. To confirm this claim, pro-inflammatory cytokines genes expression in the encountered cells was studied.
Our data showed that targeting of macrophage/dendritic cells with LCMNP–HBsAg induced pro-inflammatory cytokines gene expression but when mannose was added to the nano-vaccine as MLCMNP–HBsAg, the highest level of IL-6, TNF-α and IFN-γ gene expression was observed. This finding confirm our hypothesis in which targeting of macrophage/dendritic cells with a nano-vaccine containing mannose can efficiently stimulate innate immune cells [Citation55] and thereby can affect acquired immune responses. DCs, known as professional antigen presenting cells, could efficiently ingest, process and deliver antigen. Therefore, they are at the centre of initiation, regulation and maintenance of immune responses. So, by targeting a vaccine to DCs can manipulate immune responses [Citation11]. Various studies confirm that targeting of DCs with vaccines conjugated to mannose via targeting of mannose receptor can modulate cytokine response of DCs [Citation55]. After in vitro characterization, the potency of MLCMNP–HBsAg nano-vaccine was examined in the mouse model.
Results of specific humoural immune responses showed that our targeted nano-vaccine, which was linked to mannose, was more successful than nano-vaccine without mannose in the inducing antibody responses and even in relation to the commercial vaccine. This can be due to the targeted and effective delivery of the antigen to dendritic cells, which promotes T cell stimulation and subsequently, cytokine released from T cells provide more help to B cell for differentiation to plasma cells and antibody secretion [Citation56]. Previous studies also confirm our finding in antibody responses in various vaccine models [Citation55,Citation57]. The results of IgG2a/IgG1 ratio indicated a significant increase in MLCMNP-HBsAg group compared with LCMNP-HBsAg, commercial vaccine and mere HBsAg groups. This finding confirms the potency of targeting with mannose in the induction of Th1 pattern as reported with other researcher’s [Citation55,Citation58].
Results of lymphocyte proliferation showed that immunization with MLCMNP-HBsAg and LCMNP-HBsAg increased lymphocyte proliferation compared with commercial vaccine and mere HBsAg. Adding mannose to MLCMNP-HBsAg increased lymphocyte proliferation versus LCMNP-HBsAg around 10% but statistically was not significant. However, various studies have shown that mannosylation of a vaccine can increase lymphocyte proliferation [Citation59]. But we did not achieve such results and this may relate to the nature of antigen or condition of formulation.
Results of cytokine assay show that immunization with MLCMNP-HBsAg vaccine increased TNF-α, IL-2, IL-12, IL-4 but not IFN-γ cytokines versus the non-mannosylated nano-vaccine group (LCMNP-HBsAg). These findings show that adding mannose to the structure of nano-vaccine can modulate cytokines patterns toward pro-inflammatory responses as reported in other works [Citation55], however, does not change IFN-γ response but according to increase of IL-2, IL-12 and IL-4, it can be concluded both Th1 and Th2 are highly induced when mannose was added to the vaccine formulation. In addition, immunization with MLCMNP-HBsAg increased IL-2, IL-12 and IL-4 versus commercial vaccine, while immunization with LCMNP-HBsAg does not show such responses. These findings show that HBsAg in the format of nano-vaccine which conjugated with mannose is more potent than commercial alum based vaccine in the induction of Th1/Th2 immune responses as confirmed by antibody response. Various studies show that mannose in the structure of nano-vaccine can shifted the immune response toward a Th1 pattern and also improvement of humoural immune responses [Citation60]. In overall, results of the present study show that mannose in the structure of HBsAg nano-vaccine can target the vaccine to APCs and thereby trigger higher level of cellular and humoural immune responses and this strategy can be used as an alternative vaccine delivery for HBsAg vaccine.
Acknowledgements
The authors want to thank Dr Maryam Khatami, Dr Hassan Noorbazargan, Miss Fatemeh Asgarhalvaei, Mr Mohammad Mahdi Ghahari, Mohammad Mehdi Adibzadeh and Dr Razieh Ghasemi for their technical supports.
Disclosure statement
The authors declare that there is no conflict of interest.
Additional information
Funding
References
- Cardell K, Akerlind B, Sallberg M, et al. Excellent response rate to a double dose of the combined hepatitis A and B vaccine in previous nonresponders to hepatitis B vaccine. J Infect Dis. 2008;198:299–304.
- Mishra D, Mishra PK, Dabadghao S, et al. Comparative evaluation of hepatitis B surface antigen-loaded elastic liposomes and ethosomes for human dendritic cell uptake and immune response. Nanomed: Nanotechnol Biol Med. 2010;6:110–118.
- Kristina Cardell AFBN. Intradermal hepatitis B vaccination in health care workers. Response rate and experiences from vaccination in clinical practise. Scand J Infect Dis. 1999;31:197–200.
- Filippelli M, Lionetti E, Gennaro A, et al. Hepatitis B vaccine by intradermal route in non responder patients: an update. World J Gastroenterol. 2014;20:10383–10394.
- Mehrabi M, Dounighi NM, Rezayat SM, et al. Novel approach to improve vaccine immunogenicity: mannosylated chitosan nanoparticles loaded with recombinant hepatitis B antigen as a targeted vaccine delivery system. J Drug Deliv Sci Technol. 2018;44:19–26.
- Keler T, Ramakrishna V, Fanger MW. Mannose receptor-targeted vaccines. Expert Opin Biol Ther. 2004;4:1953–1962.
- Apostolopoulos V, Thalhammer T, Tzakos AG, et al. Targeting antigens to dendritic cell receptors for vaccine development. J Drug Deliv. 2013;2013:869718.
- Apostolopoulos V, Pietersz GA, Gordon S, et al. Aldehyde-mannan antigen complexes target the MHC class I antigen-presentation pathway. Eur J Immunol. 2000;30:1714–1723.
- Tan MC, Mommaas AM, Drijfhout JW, et al. Mannose receptor-mediated uptake of antigens strongly enhances HLA class II-restricted antigen presentation by cultured dendritic cells. Eur J Immunol. 1997;27:2426–2435.
- Alireza H, Amin Z, Mahboubeh E, et al. Targeting Pattern Recognition Receptors (PRRs) in nano- adjuvants: current perspectives. Curr Bionanotechnol. 2016;2:47–59.
- Hamdy S, Haddadi A, Shayeganpour A, et al. Activation of antigen-specific T cell-responses by mannan-decorated PLGA nanoparticles. Pharm Res. 2011;28:2288–2301.
- Wang T, Zou M, Jiang H, et al. Synthesis of a novel kind of carbon nanoparticle with large mesopores and macropores and its application as an oral vaccine adjuvant. Eur J Pharm Sci: Off J Eur Federation Pharm Sci. 2011;44:653–659.
- Chen YS, Hung YC, Lin WH, et al. Assessment of gold nanoparticles as a size-dependent vaccine carrier for enhancing the antibody response against synthetic foot-and-mouth disease virus peptide. Nanotechnology. 2010;21:195101.
- Niut Y, Popatt A, Yu M, et al. Recent advances in the rational design of silica-based nanoparticles for gene therapy. Ther Deliv. 2012;3:1217–1237.
- Zhao L, Seth A, Wibowo N, et al. Nanoparticle vaccines. Vaccine. 2014;32:327–337.
- Al-Rhman RMA. Impact of silver nanoparticles on immune responses as vaccine against activated Staphylococcus aureus bacteria. Int J Sci Res. 2017;6(9):280–284.
- Scherer F, Anton M, Schillinger U, et al. Magnetofection: enhancing and targeting gene delivery by magnetic force in vitro and in vivo. Gene Ther. 2002;9:102–109.
- Zhao Y, Zhao X, Cheng Y, et al. Iron oxide nanoparticles-based vaccine delivery for cancer treatment. Mol Pharm. 2018;15:1791–1799.
- Hee Kim E, Lee H, Kook Kwak B, et al. Synthesis of ferroluid with magnetic nanoparticles by sonochemical method for MRI contrast agent. J Magn Magn Mater. 2005;289:328–330.
- Rosengart AJ, Kaminski MD, Chen H, et al. Magnetizable implants and functionalized magnetic carriers: a novel approach for noninvasive yet targeted drug delivery. J Magn Magn Mater. 2005;293:633–638.
- Yanase M, Shinkai M, Honda H, et al. Antitumor immunity induction by intracellular hyperthermia using magnetite cationic liposomes. Jpn J Cancer Res: Gann. 1998;89:775–782.
- Bohara RA, Pawar SH. Innovative developments in bacterial detection with magnetic nanoparticles. Appl Biochem Biotechnol. 2015;176:1044–1058.
- Zeng L, Luo K, Gong Y. Preparation and characterization of dendritic composite magnetic particles as a novel enzyme immobilization carrier. J Mol Catal B: Enzymatic. 2006;38:24–30.
- Tu J, Du G, Reza Nejadnik M, et al. Mesoporous silica nanoparticle-coated microneedle arrays for intradermal antigen delivery. Pharm Res. 2017;34:1693–1706.
- Andrade Â, Ferreira R, Fabris J, et al. Coating nanomagnetic particles for biomedical applications. Biomed Eng-Front Challenges: InTech. 2011;61:69–90.
- Bahrami K, Sheikh Arabi M. Copper immobilized ferromagnetic nanoparticle triazine dendrimer (FMNP@TD–Cu(ii))-catalyzed regioselective synthesis of 1,4-disubstituted 1,2,3-triazoles. New J Chem. 2016;40:3447–3455.
- Yang D, Hu J, Fu S. Controlled synthesis of magnetite − silica nanocomposites via a seeded sol − gel approach. J Phys Chem C. 2009;113:7646–7651.
- Khalafi-Nezhad A, Nourisefat M, Panahi F. l-Cysteine functionalized magnetic nanoparticles (LCMNP): a novel magnetically separable organocatalyst for one-pot synthesis of 2-amino-4H-chromene-3-carbonitriles in water. Org Biomol Chem. 2015;13:7772–7779.
- Ghotbi Z, Haddadi A, Hamdy S, et al. Active targeting of dendritic cells with mannan-decorated PLGA nanoparticles. J Drug Target. 2011;19:281–292.
- Bradford MM. A rapid and sensitive method for the quantitation of microgram quantities of protein utilizing the principle of protein-dye binding. Anal Biochem. 1976;72:248–254.
- Prashant CK, Bhat M, Srivastava SK, et al. Fabrication of nanoadjuvant with poly-epsilon-caprolactone (PCL) for developing a single-shot vaccine providing prolonged immunity. Int J Nanomedicine. 2014;9:937–950.
- Carrillo-Conde B, Song EH, Chavez-Santoscoy A, et al. Mannose-functionalized “pathogen-like” polyanhydride nanoparticles target C-type lectin receptors on dendritic cells. Mol Pharm. 2011;8:1877–1886.
- Masuko T, Minami A, Iwasaki N, et al. Carbohydrate analysis by a phenol-sulfuric acid method in microplate format. Anal Biochem. 2005;339:69–72.
- Shukla S, Jadaun A, Arora V, et al. In vitro toxicity assessment of chitosan oligosaccharide coated iron oxide nanoparticles. Toxicol Rep. 2015;2:27–39.
- Hardy E, Martínez E, Diago D, et al. Large-scale production of recombinant hepatitis B surface antigen from Pichia pastoris. J Biotechnol. 2000;77:157–167.
- Qadis AQ, Goya S, Yatsu M, et al. Effects of a bacteria-based probiotic on subpopulations of peripheral leukocytes and their cytokine mRNA expression in calves. J Vet Med Sci. 2014;76:189–195.
- Gonzalez DD, Rimondi A, Aguirreburualde MP, et al. Quantitation of cytokine gene expression by real time PCR in bovine milk and colostrum cells from cows immunized with a bovine rotavirus VP6 experimental vaccine. Res Vet Sci. 2013;95:703–708.
- Wickert L, Steinkrüger S, Abiaka M, et al. Quantitative monitoring of the mRNA expression pattern of the TGF-β-isoforms (β1, β2, β3) during transdifferentiation of hepatic stellate cells using a newly developed real-time SYBR Green PCR. Biochem Biophys Res Commun. 2002;295:330–335.
- Fard NN, Noorbazargan H, Mirzaie A, et al. Biogenic synthesis of AgNPs using Artemisia oliveriana extract and their biological activities for an effective treatment of lung cancer. Artif Cells Nanomed Biotechnol. 2018;27:1–12.
- Pfaffl MW. A new mathematical model for relative quantification in real-time RT-PCR. Nucleic Acids Res. 2001;29:e45–e4e.
- Solaro R, Chiellini F, Battisti A. Targeted delivery of protein drugs by nanocarriers. Materials. 2010;3:1928–1980.
- Peng XH, Qian X, Mao H, et al. Targeted magnetic iron oxide nanoparticles for tumor imaging and therapy. Int J Nanomed. 2008;3:311–321.
- Nahar M, Jain NK. Preparation, characterization and evaluation of targeting potential of amphotericin B-loaded engineered PLGA nanoparticles. Pharm Res. 2009;26:2588–2598.
- Bhattacharjee S. DLS and zeta potential – what they are and what they are not? J Control Release. 2016;235:337–351.
- Agarwal M, Kumar Agarwal M, Shrivastav N, et al. Preparation of chitosan nanoparticles and their in-vitro characterization. Int J Life Sci Sci Res. 2018;4:1713–1720.
- Cole MA, Thissen H, Losic D, et al. A new approach to the immobilisation of poly(ethylene oxide) for the reduction of non-specific protein adsorption on conductive substrates. Surf Sci. 2007;601:1716–1725.
- Cuiling R, Jinhua L, Xingguo C, et al. Preparation and properties of a new multifunctional material composed of superparamagnetic core and rhodamine B doped silica shell. Nanotechnology. 2007;18:345604.
- Chen L, Xu Z, Dai H, et al. Facile synthesis and magnetic properties of monodisperse Fe3O4/silica nanocomposite microspheres with embedded structures via a direct solution-based route. J Alloys Compounds. 2010;497:221–227.
- Ma D, Guan J, Dénommée S, et al. Multifunctional nano-architecture for biomedical applications. Chem Mater. 2006;18:1920–1927.
- Nigam S, Barick KC, Bahadur D. Development of citrate-stabilized Fe3O4 nanoparticles: conjugation and release of doxorubicin for therapeutic applications. J Magn Magn Mater. 2011;323:237–243.
- Häfeli UO, Pauer GJ. In vitro and in vivo toxicity of magnetic microspheres. J Magn Magn Mater. 1999;194:76–82.
- Zhao Y, Zhao X, Cheng Y, et al. Iron oxide nanoparticles-based vaccine delivery for cancer treatment. Mol Pharm. 2018;15(5):1791–1799.
- Prego C, Paolicelli P, Díaz B, et al. Chitosan-based nanoparticles for improving immunization against hepatitis B infection. Vaccine. 2010;28:2607–2614.
- Apostolopoulos V, McKenzie I. Role of the mannose receptor in the immune response. Curr Mol Med. 2001;1:469–474.
- Irache JM, Salman HH, Gamazo C, et al. Mannose-targeted systems for the delivery of therapeutics. Expert Opin Drug Deliv. 2008;5:703–724.
- Boscardin SB, Hafalla JC, Masilamani RF, et al. Antigen targeting to dendritic cells elicits long-lived T cell help for antibody responses. J Exp Med. 2006;203:599–606.
- Xiang SD, Wilson K, Day S, et al. Methods of effective conjugation of antigens to nanoparticles as non-inflammatory vaccine carriers. Methods. 2013;60:232–241.
- Lepenies B, Lee J, Sonkaria S. Targeting C-type lectin receptors with multivalent carbohydrate ligands. Adv Drug Deliv Rev. 2013;65:1271–1281.
- Agnes M, Tan A, Jordens R, et al. Strongly increased efficiency of altered peptide ligands by mannosylation. Int Immunol. 1998;10:1299–1304.
- Yeeprae W, Kawakami S, Yamashita F, et al. Effect of mannose density on mannose receptor-mediated cellular uptake of mannosylated O/W emulsions by macrophages. J Control Release. 2006;114:193–201.