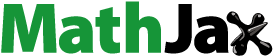
Abstract
Osteomyelitis is difficult to treat because infective bone is poorly accessible for intravenously administering antibiotics and biofilm formation increases bacterial resistance. In this study, microspheres prepared using poly(lactide-co-glycolide) (PLGA) and embedded with moxifloxacin (MOX–PLGA microspheres) and rifampicin/moxifloxacin (RIF/MOX–PLGA microspheres) using the water-in-oil-in-water double emulsion solvent evaporation technique were used for local delivery. Shape of MOX–PLGA microspheres and RIF/MOX–PLGA microspheres were spherical, mean particle size of them were 20.52 μm and 16.62 μm, respectively. Encapsulation efficiency of the MOX-PLGA microspheres was 17.35% ± 2.42%. However, the encapsulation efficiency for MOX and RIF in RIF/MOX–PLGA microspheres was 33.25% ± 7.51% and 49.0% ± 11.25%, respectively. Moxifloxacin and rifampicin were released slowly from microspheres. Both microspheres can efficiently release antibiotics in vitro. Antibacterial and bacterial biofilm-inhibition properties of the released solution were investigated from RIF/MOX–PLGA, MOX–PLGA, and blank PLGA microspheres at varying time points in vitro. RIF/MOX–PLGA microspheres demonstrated the strongest antibacterial activity and bacterial biofilm-inhibition property than the other two microspheres (p < .05). This study suggests that the novel RIF/MOX–PLGA microspheres can be used as a promising carrier for osteomyelitis treatment.
Osteomyelitis is caused by open fractures and following orthopaedic reconstruction operations is a serious complication [Citation1,Citation2]. Bacteria can invade the body via an invasive surgery or trauma and adhere to the surface of the implant or ischaemic bone. Bacteria rapidly replicates and results in severe inflammation. Osteomyelitis is more difficult to treat as it easily relapses than other infectious diseases in the body and mainly attributed to three aspects. First, intravenous antibiotics cannot easily reach the infected bone owing to the blood–bone barrier [Citation3]. Second, bacteria form a biofilm to protect them against the body’s defence mechanisms and against antibiotics, resulting in a continuous production of pathogens that worsens inflammation [Citation4–6]. Third, osteomyelitis is usually caused by Staphylococcus aureus, which accounts for approximately 80% of all pathogenic bacteria and tends to form biofilms [Citation7]. Reportedly, S. aureus that is engulfed by osteoblasts lives within them, which alters the function of osteoblasts, leading to bone destruction [Citation8]. Thus, the organism evades immune responses, body fluids and antibacterial agents. Therefore, several problems must be solved to treat osteomyelitis, such as improving bactericidal concentration at the infected site, inhibiting bacterial biofilm formation, killing bacteria within the biofilm and determining the antibacterial agents that can be used in bone infection.
Implanted local antibiotic delivery system is a good modality for treating osteomyelitis as the concentration of antibiotics can be continuously increased at the infected site and antibiotic systemic toxicity be reduced [Citation9,Citation10]. Polymethyl methacrylate (PMMA) was the first local drug delivery system developed for treating bone infections and is still widely used in clinical practice [Citation11]. However, its clinical effect is hindered by several shortcomings. A small quantity of antibiotics that can resist heat generated by PMMA may be loaded in the bone cement. Furthermore, most drugs loaded in the bone cement are not released, and as bone cement is not biodegradable, it has to be removed with a second surgery. Therefore, the use of biodegradable drug carriers with antibiotics targeting S. aureus and bacterial biofilm is a topic of interest for treating bone infections [Citation12,Citation13]. Poly(lactide-co-glycolide) (PLGA) is a matrix of microspheres and has the advantages of low immunity, good biocompatibility and regulatory degradability. Thus, PLGA has been widely used as a drug delivery system for administering molecules such as proteins, polypeptides and genes [Citation14,Citation15]. Rifampicin (RIF) is an effective broad-spectrum antibiotic with strong activity against bacterial replication and latent bacteria. Furthermore, RIF is more efficacious in inhibiting bacterial RNA polymerase both intracellularly and extracellularly than vancomycin and tobramycin [Citation16]. Moreover, RIF can effectively penetrate the polysaccharide matrix of the bacterial biofilm and kill S. aureus within it, including methicillin-resistant Staphylococcus aureus (MRSA). RIF has high bioavailability and shows good distribution in the bone [Citation17]. RIF in combination with other antibiotics significantly reduces bacterial resistance to RIF. Studies have shown that quinolones can effectively kill gram-negative bacteria in biofilms. Quinolones combined with RIF can significantly improve the success rate of osteomyelitis treatment and reduce the risk of bacterial resistance [Citation18–20]. Clinically, Staphylococcus species comprises the major pathogenic bacteria that cause implant- or post-trauma-related bone infections. The combined use of RIF and quinolone has the best efficacy, regardless of whether it is used for MRSA, and is superior to other combination treatment for osteomyelitis [Citation21]. Moxifloxacin (MOX) is a fourth-generation quinolone and has excellent broad-spectrum antibacterial properties and low systemic toxicity; thus, it has been used for treating chronic osteomyelitis. In addition, previous studies have confirmed that compared with other antibiotics such as glycopeptides, clindamycin and RIF, MOX exhibits higher bioavailability and bone tissue penetration to enter bone cells and kill latent S. aureus [Citation22].
To date, a few studies have investigated the efficacy of loaded PLGA as an antibiotic carrier for treating osteomyelitis [Citation14,Citation23]. However, no study has investigated the efficacy of PLGA microspheres loaded with both RIF and MOX (RIF/MOX–PLGA microspheres). In this study, we prepared novel RIF/MOX–PLGA microspheres using water-in-oil-in-water (w/o/w) double emulsion solvent evaporation technique. Thus, this study aimed to evaluate the antibacterial properties, morphology, particle size distribution, drug loading rate, encapsulation rate and in vitro release of these microspheres and to explore and compare the antibacterial and anti-biofilm activities of single and combined drug-loaded PLGA microspheres.
Materials and methods
Materials
PLGA (LA/GA = 50/50, MW = 9 × 104) was purchased from the Medical Equipment Institute in Shandong, China. RIF and MOX were procured from Sigma-Aldrich (St. Louis, MO, USA). Polyvinyl alcohol (PVA) and dichloromethane (DCM) were used without further purification. Fluorescence dye (DEAD/LIVE BacLight Kit, Invitrogen, Carlsbad, CA, USA) was used for bacterial staining.
RIF/MOX–PLGA and MOX–PLGA microsphere preparation
RIF/MOX–PLGA microspheres were prepared using the w/o/w double emulsion solvent evaporation method. Briefly, 30 mg/mL of MOX in deionised water solution was configured as the internal water phase (W1). Then, 240 mg of PLGA and 30 mg of RIF were dissolved in 2 ml of DCM, which comprised the oil phase (O). Thereafter, 1 ml of MOX deionised water solution was added to O and stirred in a homogeniser (Biospec Product Inc., Bartlesville, OK, USA) at 7000 rpm for 2 min to prepare the w/o emulsion. Subsequently, 3 ml of the w/o emulsion was slowly added to 40 ml of 1% PVA to prepare the w/o/w emulsion. Then, the w/o/w emulsion was added to 800 ml of 0.5% PVA and was stirred in the homogeniser at 6000 rpm for 3 h at room temperature. Finally, the microspheres that deposited at the bottom of the beaker were centrifuged and washed thrice with deionized water. The RIF/MOX–PLGA microspheres thus obtained were dried by freeze drying at −40 °C for 24 h (Scient 2–10 N, Ningbo Xinzhi biotechnology Co. Ltd., Ningbo, Zhejiang, China). MOX–PLGA microspheres were obtained by the same method and stored at 4 °C for further use.
Characterization of RIF/MOX–PLGA and MOX–PLGA microspheres
The dried microspheres were directly coated onto the conductive film and were sprayed with gold under vacuum conditions. The morphology of the microspheres was then observed using scanning electron microscopy (SEM; S-3400N, Hitachi Co. Ltd., Tokyo, Japan) at 10.0 KV. Particles were investigated after suspending dry microspheres in deionized water, and a 2 ml aliquot was placed in laser particle sizer (Microtrac X-100, Largo, FL, USA).
Determination of drug loading and entrapment efficiency
In this study, 10 mg of drug-loaded PLGA microspheres were completely dissolved in dimethyl sulfoxide under gentle ultrasonic treatment. This solution was then added to 2 ml of methanol, and the loading drug was completely dissolved for 30 min by ultrasonic treatment. Up to 5 ml of methanol was added to the solution and centrifuged at 14000 rpm for 10 min. The supernatant was collected and diluted to 10 ml with methanol water (1:1) and was analyzed to determine the amounts of drugs loaded in PLGA microspheres by high performance liquid chromatograph (HPLC, Agilent 1200, Santa Clara, CA, USA)
Entrapment Efficiency and drug loading were calculated using the following equations:
In vitro drug release
In this study, 10 mg of drug-loaded PLGA microspheres were immersed in centrifuge tubes containing 10 ml of phosphate buffered saline (PBS; pH = 7.4) and 1% of tween 20. The tubes were incubated in a shaking incubator (FLY-100B Shaking Incubator, Shanghai Fengling Laboratory Instrument Co. Ltd., Shanghai, China) at 100 rpm and 37 °C. The tubes were centrifuged at preset time (8 h, 24 h, 48 h, 72 h, 96 h, 120 h, 144 h and 168 h), after which supernatants were collected and replaced with fresh and prewarmed PBS (pH = 7.4, 37 °C) to maintain constant volume. The collected supernatants were examined in triplicate using HPLC under aforementioned conditions.
Bacterial culture
Two strains of S. aureus (S. aureus 211447779, 21872738 and 21561186) were isolated from patients with clinical bone infection (provided by the experimental centre of the General Hospital of Ningxia Medical University). The bacteria were inoculated in Columbia sheep blood agar plate (bioMérieux, France) at 37 °C for 24 h. After inoculation, bacterial suspension containing 1 × 106 CFUs/mL was prepared for further use.
Antibacterial testing by time-kill assays and Kirby–Bauer method
Time-kill assay modified from that reported in a previous study [Citation24] was performed as follows: 50 mg of RIF/MOX–PLGA and MOX–PLGA microspheres were added to 3 ml of the S. aureus suspension. Blank PLGA microspheres were used as controls. The bacterial suspension was incubated at 37 °C under constant agitation. Aliquots of 100 μL of bacterial broth was obtained from each sample at 0 h, 1 h, 2 h, 4 h, 8 h, 12 h, 24 h and 48 h. Subsequently, the bacterial broth was evenly inoculated on MH agar plate (Oxoid, Basingstoke, Hampshire, UK) using sterile glass rods and incubated for 18 h at 37 °C. Finally, the logarithm of the colony number at different time points was calculated to plot the time–sterilization curve.
The efficiency of the antibacterial agent was determined using the Kirby–Bauer method. In this case, 1 × 106 CFUs/ml of S. aureus was completely spread on the MH agar plate. Blank disks soaked with the liquid released from MOX–PLGA and RIF–PLGA microspheres were collected and naturally dried. These dried disks were plated on MH agar plate, which were then incubated 37 °C for 24 h, and the zone of inhibition was measured.
Quantitative tests for inhibition of bacterial biofilm formation
To test the inhibition of bacterial biofilm formation of the liquid released from microspheres, crystal violet assay was performed using 96-well culture plates. Bacterial biofilms were categorized as preliminary (cultured to 6 h) and mature (cultured to 24 h). The liquid released from microspheres was added to each well of the 96-well culture plate at these two-time points and incubated at 37 °C for 24 h. Then, the plates were washed with distilled water thrice to remove planctobacteria. Subsequently, methanol was added for fixing the biofilm, which was stained using crystal violet (5 min). Ice-cold acetic acid was then added in crystal violet, and quantitative biofilm formation was then assessed at OD590 using a multi-functional micropore detector (SYNERGY2, American BioTek Co., USA).
Qualitative tests for determining the inhibition of bacterial biofilm formation
Bacterial biofilms were prepared in 24-well culture plates with microslides and observed under the confocal laser scanning microscope (CLSM; Olympus FV1000, Tokyo, Japan). Then, 500 μL of diluted bacterial (S. aureus 21561186) broth was added into a 24-well culture plate, and 500 μL of collected liquid released from the microspheres was added into the bacterial broth and incubated for 24 h. Bacterial biofilms were fixed and stained using 500 μL of a combination dye (DEAD/LIVE BacLight Kit, Invitrogen, Carlsbad, CA) for 20 min. Dead and viable bacterial cells on the microslides were observed using CLSM.
Statistical analysis
All quantitative values are presented as mean ± standard deviation. The Tukey’s post-hoc test was used to compare differences among blank PLGA, MOX–PLGA and RIF/MOX–PLGA microspheres. Values of p < .05 were considered statistically significant. All statistical analyses were performed using SPSS 19.0 (IBM Corp., Armonk, NY).
Results
SEM results () showed that under amplification, RIF/MOX–PLGA microspheres had smoother surface in spherical shape, whereas MOX–PLGA microspheres had irregular surface with some holes (), which may be related to the hydrophilic property and low entrapment rate of MOX. The size distribution of RIF/MOX–PLGA and MOX–PLGA microspheres is shown in . As shown, both size distribution curves are entirely consistent with the Gaussian distribution. Drug-loaded PLGA microspheres were found to have uniform grain diameter and narrow grain distribution. The mean diameters of MOX–PLGA and RIF/MOX–PLGA microspheres were 20.52 and 16.62 μm, respectively.
Figure 1. Scanning electron microscopy images of RIF/MOX–PLGA (a1, a2) and MOX–PLGA microspheres (b1, b2). Scale for size of microspheres in a1 and b1 is 50 μm. Scale for size of microspheres in a1 and b1 is 10 μm.
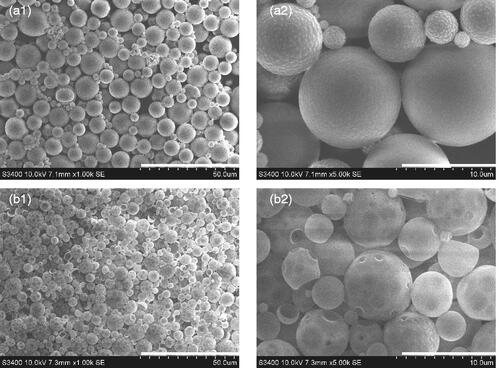
The drug loading rate for MOX–PLGA microspheres was 2.10% ± 0.12%, with an encapsulation efficiency of 17.35% ± 2.42%. However, the drug loading rates for MOX and RIF in RIF/MOX–PLGA microspheres were 3.42% ± 0.74% and 5.03% ± 0.85%, with encapsulation efficiency of 33.25% ± 7.51% and 49.0% ± 11.25%, respectively.
The release rates of the two drugs in 7 days are shown in . As shown, no burst release of MOX and RIF was observed within the first 24 h. However, 81.2% of MOX was released from MOX–PLGA microspheres after 168 h because of the low encapsulation efficiency. Compared with MOX–PLGA microspheres, 67.9% of MOX and 54.4% of RIF were released from RIF/MOX–PLGA microspheres within 168 h.
Figure 3. In vitro MOX release curves from MOX–PLGA microspheres (a) and in vitro MOX and RIF release curves from RIF/MOX–PLGA microspheres (b).
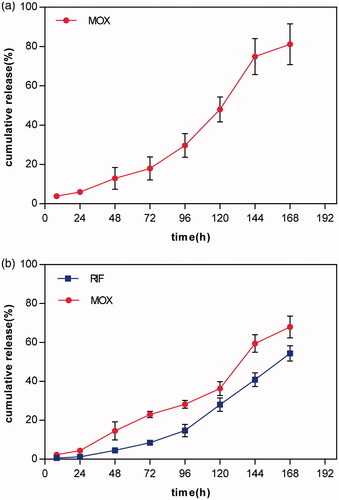
The results of the time-kill assay are shown in . The time-kill curve of RIF/MOX–PLGA microspheres lies below the two other curves, implying that RIF/MOX–PLGA microspheres required lesser time than MOX–PLGA and unloaded PLGA microspheres for sterilizing both S. aureus 211447779 and 21872738. The results of the agar disk diffusion test are shown in . Compared with the liquid released from MOX–PLGA and unloaded PLGA microspheres, that released from RIF/MOX–PLGA microspheres demonstrated a significantly larger inhibition zone for S. aureus 211447779 (p < .001). S. aureus 21872738 demonstrated resistance to the liquid released from MOX–PLGA microspheres; however, the liquid released from RIF/MOX–PLGA microspheres still had good inhibitory effect on S. aureus 21872738.
Figure 4. Results of the time-kill assay of the unloaded PLGA, MOX–PLGA and RIF/MOX–PLGA microspheres (a,b).
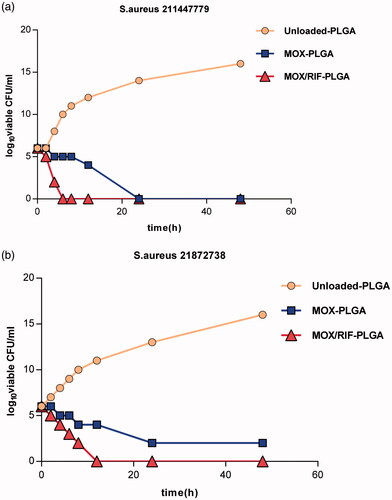
Figure 5. Inhibition zone under different time points exhibited by the emulsion medium from MOX–PLGA microspheres and RIF/MOX–PLGA microspheres against S. aureus 211447779 (a) and 21872738 (b).
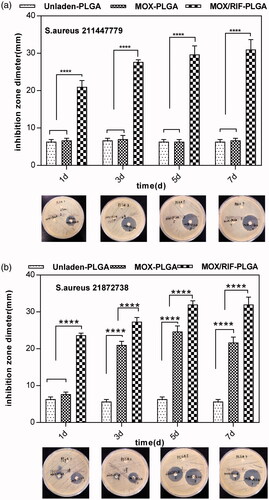
The crystal violet test confirmed that the drug-loaded PLGA microspheres inhibited both preliminary and mature bacterial biofilms in vitro (). A significantly lower absorbance value (p < .05) was obtained for the liquid released from RIF/MOX–PLGA microspheres than that released from both MOX–PLGA and unloaded PLGA microspheres. This demonstrated that RIF/MOX–PLGA microspheres have a good inhibitory effect for both preliminary and mature bacterial biofilms.
Figure 6. Results at different time points using the emulsion medium against both preliminary (a1, a2) and mature (b1, b2) stages of biofilm formation. The level of crystal violet absorption was used to estimate biofilm formation.*p < .05 was considered statistically significant.
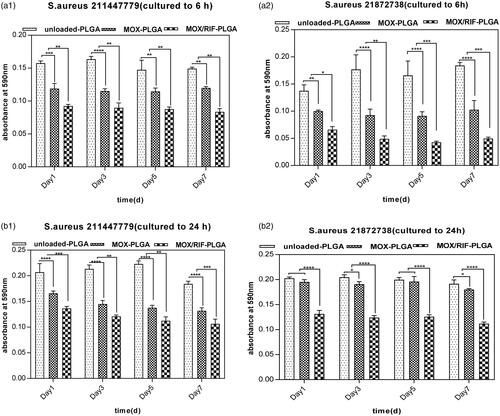
Biofilm formation on microslides was observed using CLSM (), where red and green fluorescence indicated dead and living bacteria, respectively. For the crystal violet test, RIF/MOX–PLGA microspheres had a strong sterilization effect on bacterial biofilm. Compared with the other two microslides, more red fluorescence was observed for the microslide treated with the liquid released from RIF/MOX–PLGA microspheres.
Discussion
Osteomyelitis is a challenge for orthopaedists because of blood–bone barrier that restricts the efficacy of intravenous antibiotics, self-protective mechanism of bacterial biofilms, the ability of S. aureus to invade and alter the function of osteoblasts [Citation25,Citation26]. The local delivery of antibiotics after the debridement of infected sites is a good method to treat osteomyelitis and has recently become a topic of interest. However, the drug carrier for local antibiotic delivery system should be biodegradable and the antibiotic loaded in this system should target both S. aureus and the bacterial biofilm [Citation27]. In this study, we developed a novel local antibiotic delivery system using PLGA microspheres loaded with RIF and MOX using the w/o/w double emulsion solvent evaporation technique. Being a hydrophilic drug, the loading rate of MOX was significantly higher in RIF/MOX–PLGA microspheres than in the single drug-loaded microspheres. Moreover, the stability of RIF/MOX–PLGA microspheres was good and showed stable, slow drug release. Moreover, the antibacterial effect and inhibition of antibacterial biofilm formation of RIF/MOX–PLGA microspheres were better than those of single drug-loaded microspheres.
PLGA has good biocompatibility and controllable degradation rate and is widely used in biomedical engineering. It is also widely studied as a sustained-release carrier for local antibiotics for treating osteomyelitis [Citation28,Citation29]. However, the co-loading of two antibiotics into PLGA microspheres which can increase the antimicrobial spectrum and improve its antimicrobial properties has rarely been studied. Loading of two antibiotics in PLGA microspheres with high loading rate and controllable release is the key to the investigation. Therefore, selecting an appropriate preparation method according to the nature of antibiotics is essential. The w/o/w double emulsion solvent evaporation technique is a common method for preparing drug-loaded PLGA microspheres [Citation30]. This technique involves the formation of three phases: W1, O and W2. Hydrophilic drugs are usually placed in W1, hydrophobic drugs and carrier polymers are placed in O and the homogenized oil phase and inner water phase form the colostrum, which is then transferred into the W2 to achieve homogenization (W1/O/W2). Then, the emulsion is then stirred to volatilize the organic solvent; subsequently, the carrier polymer gradually solidifies to form microspheres encapsulating the drug [Citation31]. Nevertheless, previous studies have not suggested that hydrophobic and hydrophilic drugs can improve the encapsulation rate of hydrophilic drugs when they are loaded together by the technique [Citation32]. In this study, RIF is a hydrophobic drug, whereas MOX is hydrophilic. Therefore, the saturated aqueous solution of MOX was used as the W1, and the organic solution of PLGA and RIF was used as O in order to reduce the infiltration of MOX into W2 during solvent volatilization process and increase the encapsulation rate of MOX.
Certainly, many factors in the preparation process can also affect the properties of the resultant microspheres; these include the proportion of acetic acid and hydroxyacetic acid in PLGA, the nature of water phase used in the preparation process and the speed and time of emulsification [Citation33–35]. In this study, the particle size, surface properties, drug loading and encapsulation rate of the prepared microspheres were used to evaluate the microspheres to optimize the preparation process to obtain the best performing drug-loaded PLGA microspheres. The release curves of the microspheres show that the drug released at a relatively stable rate during the entire release process without any obvious burst release. Good encapsulation efficiency is an important index to measure the success of the developed drug loading system [Citation36]. In this study, RIF achieved a high encapsulation efficiency in PLGA microspheres. However, the encapsulation rate of MOX in PLGA was relatively low because of its physical and chemical properties. However, as for RIF/MOX–PLGA microspheres it was found that the encapsulation rate of MOX increased double. Thus, the oil phase comprising RIF may significantly reduce the leakage of MOX to the outer water phase. We certainly need further studies focusing on improving drug encapsulation efficiency.
S. aureus is the main causative bacterium that causes osteomyelitis [Citation7,Citation37]. Therefore, most antibiotics used for treating osteomyelitis are active primarily against S. aureus. However, S. aureus can easily form a biofilm, which is a specific environment that is conducive to bacterial growth and reproduction [Citation5,Citation38,Citation39]. Bacterial biofilm formation is the main cause of drug-resistant osteomyelitis. Incorrect drug selection and ineffective bactericidal concentration of the intravenously administered drug may result in resistant strains of S. aureus [Citation37,Citation40]. Moreover, most bacterial biofilms contain various bacteria; thus, antibiotic treatment using a single drug is often ineffective [Citation41]. Reportedly, RIF is effective against bacterial biofilms and chronic osteomyelitis, especially against implant-related osteomyelitis [Citation14,Citation15]. RIF, when used alone, is susceptible to drug resistance; therefore, RIF in combination with other antibiotics to gradually treat osteomyelitis has become a trend for treating chronic osteomyelitis [Citation42]. MOX exhibits a wide antibacterial spectrum and high bioavailability within the bone [Citation22]. In our study, both quantitative and qualitative antibacterial tests demonstrated that the combined drug-loaded microspheres exhibited more effective bacteriostasis than single drug-loaded microspheres. Surgical debridement combined with drug treatment is the standard treatment for treating osteomyelitis. Although surgical debridement can clear most inflammatory necrotic tissues and mature biofilms at the infected site, it cannot completely clear the bacterial biofilm, and the residual biofilm quickly spreads and forms a new biofilm. Therefore, antibiotic treatment should inhibit both the formation of mature residual organisms and bacterial biofilms. Therefore, in this study, we used slowly releasing antibiotics to inhibit bacterial biofilm formation at different stages. These antibiotics had a good effect on both initial and mature bacterial biofilms. Additionally, the combined drug-loaded microspheres had a significantly stronger inhibition effect on bacterial biofilm formation than single drug-loaded microspheres
Conclusion
In this study, we developed novel biodegradable antibacterial microspheres, in which both RIF and MOX were loaded into PLGA by the w/o/w double emulsion solvent evaporation technique. Compared with MOX–PLGA microspheres, RIF/MOX–PLGA microspheres can improve the encapsulation efficiency of MOX. Furthermore, the presence of both RIF and MOX enhances the antibacterial activity inhibition of antibacterial biofilm formation of the combined drug-loaded microspheres. To conclude, RIF/MOX–PLGA microspheres have a huge potential advantage of treating osteomyelitis because of its biodegradability, local drug delivery and inhibition of bacterial biofilm formation.
Disclosure statement
No conflict of interest was reported by the authors.
Additional information
Funding
References
- Willem-Jan M, Inga P, Tanja S, et al. A doxycycline-loaded polymer-lipid encapsulation matrix coating for the prevention of implant related osteomyelitis due todoxy-cycline-resistant methicillin-resistant Staphylococcus aureus. J Control Release. 2015;209:47–56.
- Berebichez-Fridman R, Montero-Olvera P, Gómez-García R, et al. An intramedullary nail coated with antibiotic and growth factor nanoparticles: an individualized state-of-the-art treatment for chronic osteomyelitis with bone defects. Med Hypotheses. 2017;105:63–68.
- Birt MC, Anderson DW, Bruce Toby E, et al. Osteomyelitis: recent advances in pathophysiology and therapeutic strategies. J Orthop. 2017;14:45–42.
- Zimmerli W. Clinical presentation and treatment of orthopaedic implant associated infection. J Intern Med. 2014;276:111–119.
- Inzana JA, Schwarz EM, Kates SL, et al. Biomaterials app-roaches to treating implant-associated osteomyelitis. Biomaterials. 2016;81:58–71.
- Rahaman MN, Bal BS, Huang W. Review: emerging developments in the use of bioactive glasses for treating infected prosthetic joints. Mater Sci Eng C. 2014;41:224–231.
- Tong SY, Davis JS, Eichenberger E, et al. Staphylococcus aureus infections: epidemiology, pathophysiology, clinical manifestations, and management. Clin Microbiol Rev. 2015;28:603–661.
- Chen P, Yao Z, Deng G, et al. Differentially expressed genes in osteomyelitis induced by Staphylococcus aureus infection. Front Microbiol. 2018;9:1–10.
- Nandi SK, Bandyopadhyay S, Das P, et al. Understanding osteomyelitis and its treatment through local drug delivery system. Biotechnol Adv. 2016;34:1305–1317.
- Hassani Besheli N, Mottaghitalab F, Eslami M, et al. Sustainable release of vancomycin from silk fibroin nanoparticles for treating severe bone infection in rat tibia osteomyelitis model. ACS Appl Mater Interfaces. 2017;9:5128–5138.
- Oh EJ, Oh SH, Lee IS, et al. Antibiotic-eluting hydrophilized PMMA bone cement with prolonged bactericidal effect for the treatment of osteomyelitis. J Biomater Appl. 2016;30:1534–1544.
- García-Alvarez R, Izquierdo-Barba I, Vallet-Regí M. 3D scaffold with effective multidrug sequential release against bacteria biofilm. Acta Biomaterialia. 2017;49:113–126.
- Solmaz MD, Farzaneh L, Mohammad BJ, et al. Ciprofloxacin HCl-loaded calcium carbonate nanoparticles: preparation, solid state characterization, and evaluation of antimicrobial effect against Staphylococcus aureus. Artif Cells Nanomed Biotechnol. 2017;45:535–543.
- Mir M, Ahmed N, Rehman AU. Recent applications of PLGA based nanostructures in drug delivery. Colloids Surf B Biointerfaces. 2017;159:217–231.
- Bhuiyan DB1, Middleton JC, Tannenbaum R, et al. Bone regeneration from human mesenchymal stem cells on porous hydroxyapatite-PLGA-collagen bioactive polymer scaffolds. BME. 2017;28:671–685.
- Alt V, Kirchhof K, Seim F, et al. Rifampicin–fosfomycin coating for cementless endoprostheses: antimicrobial effects against methicillin-sensitive Staphylococcus aureus (MSSA) and methicillin-resistant Staphylococcus aureus (MRSA). Acta Biomaterialia. 2014;10:4518–4524.
- Shiels SM, Tennent DJ, Akers KS, et al. Determining potential of PMMA as a depot for rifampin to treat recalcitrant orthopaedic infections. Injury. 2017;48:2095–2100.
- Brinkman CL, Schmidt-Malan SM, Mandrekar JN, et al. Rifampin-based combination therapy is active in foreign-body osteomyelitis after prior rifampin monotherapy. Antimicrob Agents Chemother. 2017;61:e01822-16.
- Jørgensen NP, Skovdal SM, Meyer RL, et al. Rifampicin-containing combinations are superior to combinations of vancomycin, linezolid and daptomycin against Staphylococcus aureus biofilm infection in vivo and in vitro. Pathog Dis. 2016;74:ftw019.
- Rodriguez-Pardo D, Pigrau C, Corona PS, et al. An update on surgical and antimicrobial therapy for acute periprosthetic joint infection: new challenges for the present and the future. Expert Rev Anti Infect Ther. 2015;13:249–265.
- Uhto AP, Puhto T, Niinimaki T, et al. Predictors of treatment outcome in prosthetic joint infections treated with prosthesis retention. International Orthopaedics (Sicot). 2015;39:1785–1791.
- Soranoglou V, Galanopoulos I, Giamarellos-Bourboulis EJ, et al. Efficacy of intramuscular moxifloxacin in the treatment of experimentalosteomyelitis caused by methicillin-resistant Staphylococcus aureus. Int J Antimicrob Agents. 2017;50:186–190.
- Posadowska U, Brzychczy-Włoch M, Pamuła E, et al. Gentamicin loaded PLGA nanoparticles as local drug delivery system for the osteomyelitis treatment. Acta Bioeng Biomech. 2015;17:41–48.
- Mittal A, Kumar N. Drug-loaded polymeric composite skin graft for infection-free wound healing: fabrication, characterization, cell proliferation, migration, and antimicrobial activity. Pharm Res. 2012;29:3110–3021.
- Visani J, Staals EL, Donati D. Treatment of chronic osteomyelitis with antibiotic-loaded bone void filler systems: an experience with hydroxyapatites calcium-sulfate biomaterials. Acta Orthop Belg. 2018;84:25–29.
- Cibor U, Krok-Borkowicz M, Brzychczy-Włoch M, et al. Gentamicin-loaded polysaccharide membranes for prevention and treatment of post-operative wound infections in the skeletal system. Pharm Res. 2017;34:2075–2083.
- Min J, Choi KY, Dreaden EC, et al. Designer Dual Therapy Nanolayered Implant Coatings Eradicate Biofilms and Accelerate Bone Tissue Repair. ACS Nano. 2016;10:4441–4450.
- Yang H, Hao Y, Hu N, et al. Preparation and in vitro study of hydrochlor-ic norvancomycin encapsulated poly (D,L-lactide-co-glycolide, PLGA) micros-pheres for potential use in osteomyelitis. Artif Cells Nanomed Biotechnol. 2017;45:1326–1330.
- Reinbold J, Hierlemann T, Urich L, et al. Biodegradable rifampicin-releasing coating of surgical meshes for the prevention of bacterial infections. DDDT. 2017;11:2753–2762.
- Emanuel N, Rosenfeld Y, Cohen O, et al. A lipid-and-polymer-based novel local drug delivery system—BonyPid™: from physicochemical aspects to therapy of bacterially infected bones. J Control Release. 2012;160:353–361.
- Ansary RH, Rahman MM, Awang MB, et al. Preparation, characterization, and in vitro release studies of insulin-loaded double-walled poly(lactide-co-glycolide) microspheres. Drug Deliv and Transl Res. 2016;6:308–318.
- Ramazani F, Chen W, van Nostrum CF, et al. Strategies for encapsulation of small hydrophilic and amphiphilic drugs in PLGA microspheres: state-of-the-art and challenges. Int J Pharm. 2016;499:358–367.
- Andreas K, Zehbe R, Kazubek M, et al. Biodegradable insulin-loaded PLGA microspheres fabricated by three different emulsification techniques: investigation for cartilage tissue engineering. Acta Biomater. 2011;7:1485–1495.
- Kimishima K, Matsuno T, Makiishi J, et al. Effects of gatifloxaine content in gatifloxacine-loaded PLGA and β-tricalcium phosphate composites on efficacy in treating osteomyelitis. Odontology. 2016;104:105–113.
- Dorati R, DeTrizio A, Genta I, et al. An experimental design approach to the preparation of pegylated polylactide-co-glicolide gentamicin loaded microparticles for local antibiotic delivery. Mater Sci Eng C Mater Biol Appl. 2016;58:909–917.
- ter Boo GJ, Grijpma DW, Moriarty TF, et al. Antimicrobial delivery systems for local infection prophylaxis in orthopedic- and trauma surgery. Biomaterials. 2015;52:113–125.
- Tuchscherr L, Kreis CA, Hoerr V, et al. Staphylococcus aureus develops increased resistance to antibiotics by forming dynamic small colony variants during chronic osteomyelitis. J Antimicrob Chemother. 2016;71:438–448.
- Raic A, Riedel S, Kemmling E, et al. Biomimetic 3D in vitro model of biofilm triggered osteomyelitis for investigating hematopoiesis during bone marrow infections. Acta Biomater. 2018;73:250–262.
- Iannitelli A, Grande R, Stefano AD, et al. Potential antibacterial activity of carvacrol-loaded poly(DL-lactide-co-glycolide) (PLGA) nanoparticles against microbial biofilm. IJMS. 2011;12:5039–5051.
- Claessens J, Roriz M, Merckx R, et al. Inefficacy of vancomycin and teicoplanin in eradicating and killing Staphylococcus epidermidis biofilms in vitro. Int J Antimicrob Agents. 2015;45:368–375.
- Greimel F, Scheuerer C, Gessner A, et al. Efficacy of antibiotic treatment of implant-associated Staphylococcus aureus infections with moxifloxacin, flucloxacillin, rifampin, and combination therapy: an animal study. DDDT. 2017;11:1729–1736.
- Kluin OS, Busscher HJ, Neut D, et al. Poly(trimethylene carbonate) as a carrier for rifampicin and vancomycin to target therapy-recalcitrant staphylococcal biofilms. J Orthop Res. 2016;34:1828–1837.