Abstract
Bone tissue engineering is an area of regenerative medicine that attempts to repair bone defects. Seed cells such as dental pulp stem cells (DPSCs) and adipose tissue-derived stem cells (ADSCs) are two of the most well-characterized cells for bone regeneration because their use involves few ethical constraints and they have the ability to differentiate into multiple cell types, secreting growth factors and depositing mineral. However, bone regeneration ability of these cells remains unclear. This study aimed to compare the bone formation capacity of DPSCs and ADSCs in vitro and in vivo. Studies revealed that DPSCs had enhanced colony-forming ability, higher proliferative ability, stronger migration ability and higher expression of angiogenesis-related genes. They also secreted more vascular endothelial growth factor compared to ADSCs. In contrast, ADSCs grew more slowly compared to DPSCs but exhibited greater osteogenic differentiation potential, higher expression of osteoblast marker genes, and greater mineral deposition. Furthermore, after DPSCs and ADSCs were implanted into a mandibular defect of a rat for 6 weeks, ADSCs showed visible bone tissue as early as week 1 and promoted faster and greater bone regeneration compared to the DPSC group. These results suggest that ADSCs might be more useful than DPSCs for bone regeneration.
Introduction
Bone defects are common clinical problems resulting from trauma, necrosis, tumors and nonunion of fractures, which are difficult to remedy. Nowadays, stem cell-based bone regeneration and bone tissue engineering can be considered as an attractive technique in the field of bone repair and reconstruction [Citation1,Citation2]. Mesenchymal stem cells (MSCs) for tissue engineering should have multi-differentiation potential, be easily accessible and be obtainable by minimally-invasive or non-invasive collection procedures. Bone marrow-derived mesenchymal stem cells were the first MSCs obtained [Citation3], however BMSCs are not abundant, are easily contaminated and require an invasive technique to obtain [Citation4], while MSCs derived from human dental pulp tissues (hDPSCs) and human adipose tissues (hADSCs) have been found to be promising alternative source candidates for cell transplantation in bone tissue engineering [Citation5,Citation6]. Dental pulp stem cells are the first identified dental stem cell source with mesenchymal characteristics and osteogenic potential. In comparison with BMSCs, DPSCs showed higher colony forming unit (CFU) efficiency and proliferation rates along with a similar gene expression profile for genes related to mineralization [Citation7]. The osteogenic potential of ADSCs has been proven in various animal models [Citation8,Citation9], and the potential of ADSCs for bone regeneration has been investigated in several small-scale clinical trials [Citation10,Citation11]. Dental pulp stem cells and ADSCs are both easily accessible and can be obtained in a minimally-invasive, less painful procedure compared with that used to obtain BMSCs. Both DPSCs and ADSCs have the capacity of self-renewal, generating an adequate number of cells after in vitro expansion, and have the capacity to differentiate into multiple cell phenotypes under appropriate conditions [Citation3,Citation12]. Furthermore, DPSCs and ADSCs can secrete vascular endothelial growth factor (VEGF) [Citation13,Citation14] which has been proven to play an important role in angiogenesis and bone tissue repair [Citation15]. Although a previous study has demonstrated that DPSCs are more proliferative than ADSCs [Citation15], controversy remains regarding which cell type should be recommended for bone tissue regeneration, and it is also not clear whether the osteogenic ability of different mesenchymal stem cell types is determined by their angiogenic capacity. Therefore, in this study, we systematically evaluated the CFU efficiency, proliferative capacity, migration ability, angiogenesis-related ability, and osteogenic differentiation ability of DPSCs and ADSCs in vitro. Moreover, the present study evaluated the bone regeneration potential of DPSCs and ADSCs in a rat model of a mandibular defect.
Materials and methods
Cell isolation and culture
Two mesenchymal human stem cell types were used in this study: DPSCs derived from human dental pulp and ADSCs from adipose tissue. Written informed consent was obtained from all patients. Human dental pulp tissue was obtained from fresh, healthy human third molars extracted for orthodontic treatment purposes from 20- to 25-year-old individuals at the oral surgery clinic of the Ninth People’s Hospital affiliated to Shanghai Jiao Tong University School of Medicine. The isolation and culture of DPSCs was as described by Gronthos et al. [Citation16]. The ADSCs were obtained from 25- to 35-year-old healthy female patients during liposuction procedures at the plastic surgery department of the Ninth People’s Hospital affiliated to Shanghai Jiao Tong University School of Medicine. The ADSC primary cultures were obtained by enzymatic digestion, as reported by Zuk et al. [Citation17]. Cells were cultured in Dulbecco’s modified Eagle’s medium (DMEM; Gibco, Carlsbad, CA, USA) supplemented with 10% fetal bovine serum (FBS; Gibco) and 1% penicillin/streptomycin (all from Invitrogen, Carlsbad, CA, USA) and maintained in a humidified tissue culture incubator at 37 °C with 5% CO2. Cells were passaged using 0.25% trypsin (Nacalai Tesque, Kyoto, Japan). Cell cultures between the second and fifth passages were used for experiments.
Flow cytometry
Expression of mesenchymal markers on DPSCs and ADSCs was detected by flow cytometry [Citation18]. Briefly, DPSCs and ADSCs were detached using 0.25% trypsin/EDTA (Gibco) and resuspended in PBS at a density of 1 × 106 cells/mL. The selected cells were then incubated with the following fluorescence-conjugated antibodies to: CD29-phycoerythrin (PE), CD105-PE, CD34-fluorescein isothiocyanate(FITC), CD44-FITC, CD45-FITC, as well as isotype-matched antibodies (all from BD Biosciences, San Jose, CA, USA, and Biolegend, San Diego, CA, USA) for 30 min under light-shielded conditions. Non-labelled cells and isotypes were used as negative controls. The cells were then washed three times with PBS to remove unbound antibodies and resuspended in 300 μL PBS. Cells were analyzed using a flow cytometer (FACSCalibur; BD Biosciences, Franklin Lakes, NJ, USA) and data analysis was performed using FlowJo software (FlowJo, Ashland, OR, USA).
Colony forming unit (CFU) efficiency
Colony forming unit efficiency was determined as previously reported [Citation19]. A total of 200 cells were seeded into a 6-well plate with 2.5 mL DMEM, and cultured for a further 14 days. Cells were fixed in 4% paraformaldehyde for 30 min at room temperature and stained with 5% crystal violet (Merck, Darmstadt, Germany) for 20 min at room temperature. Colonies of more than 50 cells were counted, and the CFU was calculated by dividing the total number of colonies by 200.
Cell proliferation assay
In order to compare the proliferation capacity between DPSCs and ADSCs, 1000 cells were plated into a 96-well plate and incubated for 24 h with DMEM (10% FBS and 1% penicillin/streptomycin) at 37 °C in 5% CO2. The proliferation rate was measured using a Cell Counting kit-8 assay performed from day 0 to day 10, according to the manufacturer’s protocol (CCK-8; Dojindo Kagaku Co, Kumamoto, Japan).
Cell migration assay
The migration ability of DPSCs and ADSCs was assessed using Transwell inserts, which were 6.5 mm in diameter, with 8 μm pore size (Corning Inc., Corning, NY, USA) according to the manufacturer’s protocol. Dental pulp stem cells and ADSCs were seeded at a density of 2 × 104 cells/well into the upper chambers, using 200 μL serum-free DMEM while the lower chambers contained 800 μL DMEM supplemented with 10% FBS. Chambers were subsequently incubated for 24 h at 37 °C and 5% CO2. The non-migrated cells in the upper chamber were cleared, and the migrated cells on the lower surface of the membranes were fixed with 4% paraformaldehyde for 30 min, washed twice with PBS and stained with 5% crystal violet for 20 min at room temperature. After washing with PBS, the stained cells were observed under an upright fluorescent microscope (Nikon Corporation, Tokyo, Japan). The average number of migrated cells was counted in five independent, randomly-chosen fields of view.
Osteogenic differentiation assay
To compare the in vitro osteogenic differentiation ability of DPSCs and ADSCs incubated in osteogenic medium, we performed alkaline phosphate (ALP) staining, an ALP activity assay, and a calcium deposition assay (Alizarin Red S stainingARS/AL). For ALP staining, on days 3, 5, and 7 after the start of culture in osteoblast differentiation-inducing medium, cells were fixed with 4% paraformaldehyde for 30 min then an appropriate amount of BCIP/NBT (Sigma-Aldrich, St Louis, MO, USA) staining solution was added, and the cells were incubated at room temperature in the dark, followed by washing and scanning. For the ALP activity assay, cells were harvested on days 7 and 14 after initiation of culture in osteoblast-inducing medium, seeded at 5 × 104 cells/well in a 12-well plate. At each time-point cells were incubated with p-nitrophenyl phosphate (pNPP) (Sigma-Aldrich) and detected by measurement of optical density (OD) values at 405 nm; total protein levels were also determined using a BCA (bicinchoninic acid) protein assay kit (Pierce Biotechnology, Thermo Fisher Scientific, Waltham, MA, USA), and the ALP activity was expressed as absorbance values per milligram of total protein. For the calcium deposition assay, DPSCs and ADSCs were seeded at a density of 2.5 × 104 cells cultured in each well of a 24-well plate for differentiation. The cells were cultured in growth medium (DMEM) until they reached 70% confluence, then the growth medium was replaced with human osteoblast-inducing medium (Cyagen, Santa Clara, CA, USA) to induce osteogenesis. On days 7, 14, and 21 post-treatment, cells were fixed in 4% paraformaldehyde for 30 min and stained with Alizarin Red S (Sigma-Aldrich). Stained cells were observed under an inverted phase-contrast microscope to evaluate the formation of mineralized nodules. All experiments were performed in triplicate.
Adipogenic differentiation assay
To evaluate adipogenic cell differentiation, DPSCs and ADSCs were seeded at a density of 2.5 × 104 cells per well of a 24-well plate for differentiation. The cells were cultured in growth medium (DMEM) until they reached 100% confluence or post confluence, then the growth medium was replaced with human adipogenic differentiation medium (Cyagen) to induce adipogenesis. On days 7, 14, and 21 post-treatment, cells were fixed in 4% paraformaldehyde for 30 min and stained with Oil Red O (Sigma-Aldrich). Stained cells were observed under an inverted phase-contrast microscope (Nikon Corporation, Tokyo, Japan) to evaluate the formation of lipid droplets.
Quantitative Real-Time PCR (qRT-PCR)
Total cellular RNA was extracted with TRIzol reagent (Invitrogen, Carlsbad, CA, USA) on day 3, 5 and 7, and cDNA was synthesized from total RNA using a PrimeScript RT reagent kit (Takara Bio, Inc., Shiga, Japan) according to the manufacturer’s instructions. Expression of mineralization (BMP2, RUNX2, and ALP) and angiogenic (VEGFR & PECAM1) markers was assessed using an SYBR Premix ExTaq kit (Takara Bio, Inc.) on an ABI 7500 sequencing detection system (Applied Biosystems; Thermo Fisher Scientific). The primers are listed in Supplementary Table 1. The qRT-PCR program was as follows: 40 cycles each involving 5 s of denaturation at 95 °C and 34 s of amplification at 60 °C. Relative gene expression level was analyzed according to the comparative Cq method [Citation20] and normalized to GAPDH. All PCR amplifications were performed in triplicate.
Enzyme-linked immunosorbent assay (ELISA) of VEGF
Dental pulp stem cells and ADSCs were cultured in growth medium, and the culture medium was collected after 24 h at 2 and 4 days of culture. The concentrations of VEGF were measured using an ELISA kit (R & D Systems, Minneapolis, MN, USA). For each sample, 100 μL of the medium was assayed according to the manufacturer’s instructions. Optical density was measured at 450 nm (Bio-Tek, Winooski, VT, USA). The results were calculated using a calibration curve.
Comparative bone formation in vivo
A rat mandibular bone defect model was designed to study bone formation in vivo. Animals were obtained from the Ninth People’s Hospital Animal Center (Shanghai, People’s Republic of China). The animal test followed the guidelines of the Animal Experimental Ethical Inspection–Shanghai Ninth People’s Hospital affiliated to Shanghai Jiao Tong University, School of Medicine (HKDL[2018]489). For cell seeding, both DPSCs and ADSCs were resuspended in 0.2% Puramatrix™ (BD Biosciences) at a density of 1 × 106 cells/mL according to the manufacturer’s protocol. The surgical procedure has been described previously [Citation21]. Bone defects were surgically created (2 mm diameter, 1 mm thickness) using a 1-mm burr (Hager & Meisinger GmbH, Neuss, Germany) at low rational speed. Fifteen mandibular defects were created unilaterally in 15 rats and were randomly repaired with approximately 50 μL of 0.2% Puramatrix™ alone, a 0.2% Puramatrix/DPSC complex, or a 0.2% Puramatrix/ADSC complex (n = 5 per group). At 1, 3, and 5 weeks after the operation, the animals were intraperitoneally administered 30 mg/kg Alizarin Red S(AL), 25 mg/kg tetracycline hydrochloride (TE; Sigma-Aldrich), and 20 mg/kg calcein (CA; Sigma-Aldrich) respectively. All rats were sacrificed at 6 weeks, and the samples were extracted and tissue samples were removed and fixed in 4% paraformaldehyde for further studies. To evaluate the bone regeneration of the defects in vivo, the paraformaldehyde-fixed tissue samples were scanned with a small animal micro-computed tomography instrument (Micro-CT, SkyScan 1176; BrukerOptik GmbH, Belgium). The obtained data were reconstructed and analyzed using morphometric software (TRI/3D-BON; Ratoc System Engineering, Tokyo, Japan). The bone volume fraction (BV/TV), mean trabecular number (Tb.N), mean trabecular thickness (Tb.Th) and mean trabecular separation (Tb.Sp) were calculated within the region of interest (ROI). After the micro-CT scan, all the undecalcified specimens were embedded in polymethylmethacrylate (PMMA) [Citation22]. Finally, each specimen was sectioned into 50 μm thick slices using a Leica SP1600 saw microtome (Leica, Nussloch, Germany). Sequential fluorescence labelling of the sections was observed under a confocal laser scanning microscope (LSM 700; Carl Zeiss, Oberkochen, Germany), and the fluorescent labelled area was measured and evaluated. Afterwards, Van Gieson’s picro-fuchsin staining was performed for histological analysis, and the measurements on the specimens were performed with a personal computer-based image analysis system (image-Pro Plus™, Media Cybernetics, Silver Spring, MD, USA) [Citation23].
Statistical analysis
All data are expressed as the mean ± standard deviation of at least three independent experiments. Data were analyzed using Student’s t-test and one-way analysis of variance (ANOVA) followed by Tukey’s multiple comparisons test (Prism 6.0). The significance level of the statistical analysis was set at P < .05 or P < .01.
Results
Mesenchymal stem cell characterization
Both DPSCs and ADSCs exhibited a spindle shape, but DPSCs were slightly smaller than ADSCs (). Flow cytometry data indicated that DPSCs and ADSCs expressed MSC-associated surface markers, such as CD29, CD44 and CD105; neither cell type expressed CD34 or CD45 (). Colonies were counted in cultures of DPSCs and ADSCs. Colonies were identified as clusters of > 50 cells. Both DPSCs and ADSCs formed colonies. However, a significant increase in CFUs was seen in DPSCs compared to ADSCs, suggesting an increased colony forming efficiency in the DPSCs (P < .01) (). Moreover, when compared with ADSCs, DPSCs showed a higher proliferation rate (). In addition, according to the Transwell assay, the DPSCs showed significantly stronger migration ability ().
Figure 1. Comparison of cell characteristics of dental pulp stem cells (DPSCs) and adipose-derived stem cells (ADSCs). (A) Cell morphology of DPSCs and ADSCs. (B) The results of flow cytometric analysis of DPSCs and ADSCs. (C) Crystal violet staining revealed the colony forming unit of DPSCs and ADSCs. (D) Quantitative analysis of colony-forming units in each group. (E) Proliferation curves of DPSCs and ADSCs. (F) Crystal violet staining revealed the migratory cells in cultures of DPSCs and ADSCs, and (G) Quantitative analysis of migratory cells in each group. Data are presented as the mean ± standard deviation of the mean (n = 3), **P < .01. Scale bar: 100 µm.
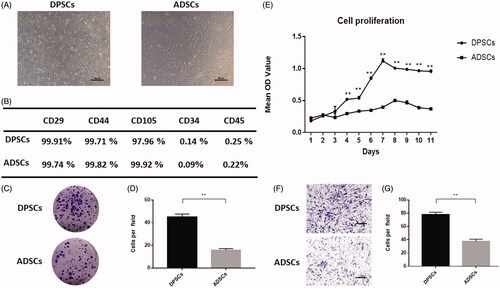
Comparison of in vitro multi-differentiation potential
The DPSCs and ADSCs exhibited multilineage differentiation potential. The ADSC group showed more intense staining on day 3 after osteogenic induction, while in the DPSC group staining could be observed on day 7 (). Moreover, the ADSCs displayed stronger ALP activity than DPSCs over the differentiation process, and there was a significant difference between groups (). In addition, mineralized calcium deposition was observed in ADSCs by ARS staining at day 7 after culture in osteogenic medium, which strongly increased by day 21 (). Dental pulp stem cells cultured in osteogenic medium showed some mineralized nodule formation on day 21. The staining results of ARS are also consistent with the trend of ALP staining. Oil Red O staining indicated differentiation of DPSCs and ADSCs into adipocyte-like cells. In addition, the presence of lipid vesicles was higher in ADSCs compared with DPSCs ().
Figure 2. Cell multi-differentiation potential. (A) Alkaline phosphate (ALP) staining at 3, 5, and 7 days after osteogenic induction. (B) ALP activity in the two groups at each time-point determined by ALP semi-quantitatively. (C) Cultures of DPSCs and ADSCs stained with ARS after 7, 14, and 21 days of osteogenic induction. (D) Cultures of DPSCs and ADSCs stained with Oil Red O after 7, 14, and 21 days of adipogenic induction Data are presented as the mean ± standard deviation of the mean (n = 3), **P < .01. Scale bar: 100 µm.
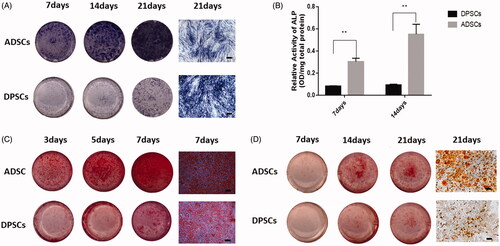
Gene expression profile of mineralization- and angiogenesis-related markers and VEGF release in DPSCs and ADSCs
There was a time-dependent change in the expression of osteogenic-related genes such as BMP2, RUNX2, and ALP (). Furthermore, the most striking feature was that the gene expression levels of each gene were obviously higher in the ADSC group than in the DPSC group beginning on day 3. Angiogenesis is known to play an essential role in bone regeneration and the bone repair of bone defects. In this study, at each time point, VEGF and PECAM-1 were more highly expressed in DPSCs than in ADSCs, and this reached statistical significance (). ELISA was used to quantify VEGF secreted by DPSCs and ADSCs. The results showed that both cells can secrete VEGF, but there was a difference between the two cell populations. The amount of VEGF secreted by the DPSC group was significantly higher than that of the ADSC group ((P < .01) ().
Figure 3. Expression of osteogenic and angiogenic markers in DPSCs and ADSCs. The mRNA levels of (A) BMP2, (B) RUNX2, and (C) ALP (osteogenesis-related genes) (D) VEGF, and (E) PECAM1 (angiogenesis-related gene) are shown. (F) VEGF secreted from DPSCs and ADSCs, detected by ELISA. Data are presented as the mean ± standard deviation of the mean (n = 3), *P < .05, **P < .01.
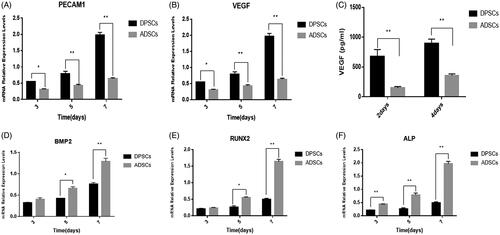
Comparison of bone formation in the mandibular defect model
According to micro-CT analysis, as shown in , the reconstructed micro-CT images were cut into circular regions with a diameter of 2 mm in accordance with the defect region, so that the presented circular area directly represented the volume of newly-formed bone. The micro-CT images () revealed that in the control group, the newly-formed bone was only present at the edges of the defects, and the shaded areas in the DPSC group were slightly larger than in the control group. However, robust new bone formation in the ADSC group was extracted after 6 weeks. Quantitative evaluation of the trabecular bone within each ROI is shown in . The BV/TV (), Tb.N (), Tb.Th (), and Tb.sp () were significantly higher in the ADSC group than in the DPSC or the control group. Next, new bone formation and mineralization were analyzed at 1, 3 and 5 weeks. According to sequential fluorescence labelling, visible new bone formation and mineralization occurred in the ADSC group as early as 1 week AL labelling (red)] after implantation. In contrast, only weak calcium deposition was observed in the DPSC group. During week 3, bone formation increased slightly in the DPSC group but was still obviously slower than in the ADSC group (). As shown in , the increases in the percentage of the labelled bone area were significantly higher in the ADSC group than in the DPSC group, both of which were significantly higher than those in the control group at different time points. Moreover, the undecalcified histological sections of ROI are presented in . The opposition of new bone along the ADSCs and DPSCs composite scaffold materials was greater than that of the hydrogel scaffolds alone, in which only a small amount of new bone was formed. New bone formation in the DPSC group was less than that observed in the ADSC group. Quantitative histomorphometric analysis within various ROIs showed that the new bone area was significantly greater in the ADSC group than in the DPSC group ().
Figure 4. The analysis of DPSC and ADSC promotion of bone regeneration by micro-CT. (A) Micro-CT images taken at 6 weeks after implantation of 0.2% Puramatrix™ alone, 0.2% Puramatrix™/DPSC complex, or 0.2% Puramatrix™/ADSC complex, respectively. (B) Bone volume/total volume (BV/TV), (C) trabecular number (Tb.N), (D) trabecular thickness (Tb.Th), and (E) trabecular separation (Tb.Sp) by micro-CT for each group at 6 weeks post operation. Data are presented as the mean ± standard deviation of the mean (n = 3), *P < .05, **P < .01. Scale bar: 200 µm.
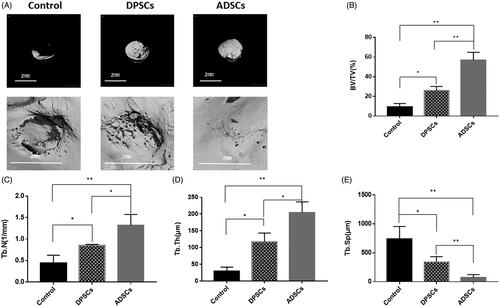
Figure 5. Analysis of DPSCs and ADSCs promotion of bone regeneration. (A) Sequential fluorescent labelling of AL, TE, and CA was administrated at 1, 3, 5 weeks post-operation. Images of column “Merge 1” represent the merged three fluorochromes. “Merge 2” represents merged images of the three fluorochromes together with the plain confocal laser microscope image. (B) The percentage of TE, AL, and CA staining for each group assessed at week 6 after implantation. (C) Histological analysis revealed that both DPSCs and ADSCs promoted bone regeneration, shown by van Gieson’s staining. The new bone area is shown in red. (D) The percentage of new bone area was assessed at 6 weeks after implantation by histomorphometric analysis. Data are presented as the mean ± standard deviation of the mean (n = 3), *P < .05, **P < .01. Scale bar: 500 µm.
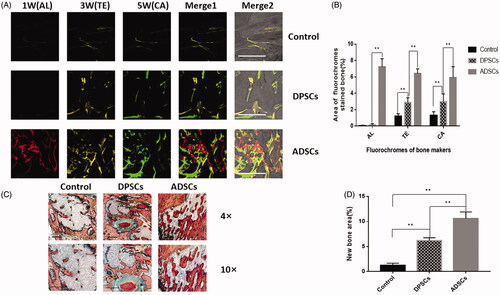
Discussion
Stem cell-based therapy has raised great interest for the treatment of bone defects and has been studied clinically [Citation24]. Both dental pulp and adipose tissue are unique sources of MSCs because of their outstanding characteristics [Citation3,Citation12]. In particular, extracted teeth constitute a very promising source since they harbor a variety of progenitor stem cells [Citation16]. Dental pulp contains a larger number of mesenchymal stem cells compared to equal volumes of bone marrow [Citation25]. Due to the great clinical potential, ease of accessibility, and less invasive harvesting process of DPSCs, they have been used in several clinical studies which indicated the extensive potential of the DPSCs in tissue repair and regeneration [Citation26,Citation27]. As well as dental pulp tissue, adipose tissue produces a population of cells that have similar features to stem cells [Citation28]. Adipose tissue-derived stem cells can be obtained repeatedly and in large quantities during plastic surgery which is a more convenient, less painful and safer procedure compared to the process required to harvest BMMCs. For this reason, ADSCs have been found to be a more promising source of stem cells in regenerative medicine compared to BMSCs [Citation3]. The results of a series of animal experiments and clinical trials have shown that ADSCs are effective seed cells for bone tissue engineering. This report is the first to systematically characterize the properties of cells harvested from dental pulp tissue in comparison with cells isolated from adipose tissue.
DPSCs have been reported to have higher proliferative ability than ADSCs [Citation15]. Data in the present study demonstrated that colonies of DPSCs developed at a higher frequency compared to ADSCs. Additionally, a much higher proliferative ability of DPSCs was observed which is in agreement with a previous report [Citation15]. These results suggest that DPSCs require a shorter culture period to reach the transplantation levels required for clinical application. Moreover, DPSCs exhibited a more accelerated migration profile, which may have important meaning for recruiting host cells and promoting damage repair. Moreover, it is well known that angiogenesis plays a critical role in bone repair and regeneration [Citation29,Citation30], and it is known that both DPSCs and ADSCs can secrete VEGF to promote angiogenesis [Citation31,Citation32]. In the present study, we demonstrated that DPSCs secrete a much higher concentration of VEGF than ADSCs. This finding was supported by the expression of corresponding genes which were considerably higher in DPSCs than ADSCs. In contrast, in vitro experiments showed higher ALP activity, more obvious mineral deposition, and stronger osteogenic gene expression in the ADSC group, indicating that the osteogenic differentiation potential of ADSCs is obviously superior to that of DPSCs. Furthermore, a rat mandibular defect model was used to evaluate the bone regeneration and bone repair capabilities of stem cells, which is more relevant to the clinical setting. In the rat mandibular model, apparent new bone formation and mineralization occurred in the ADSC group as early as 1 week after implantation, while a visible mineralization process only began 3 weeks after implantation in the DPSC group. Six weeks later, new bone had formed around the defect in the control group, but the amount of newly-formed bone was obviously lower than in the ADSC group. In conclusion, these radiological and histological results confirm the advantage of using ADSCs for bone regeneration. In the present study, although the angiogenic ability of DPSCs was higher, the corresponding osteogenic capacity was weaker in comparison to ADSCs. The results in the present study indicate that the bone repairability of mesenchymal stem cells is not only determined by the cells’ angiogenic ability, but that other factors may also play a role in bone regeneration such as the inflammatory microenvironment. Further experimental support is needed, along with a further investigation of the cross-talk between inflammatory, angiogenic, and mineralization pathways in the repair of bone defects.
In conclusion, we have demonstrated that compared with DPSCs, ADSCs show enhanced matrix mineralization capability, increased expression of osteogenic-related genes, and in vivo experiments also show that ADSCs have stronger bone repairability. These properties indicate that ADSCs are more suitable as seed cells in bone engineering than DPSCs. Considering the strong colony-forming ability, proliferative capacity, migration ability, and higher angiogenic potential of DPSCs, we speculate that DPSCs may have the potential for future use in angiogenesis, but that their efficacy and function have yet to be determined in further research.
Supplementary_Table.docx
Download ()Disclosure statement
No potential conflict of interest was reported by the authors.
Additional information
Funding
References
- Rao RR, Stegemann JP. Cell-based approaches to the engineering of vascularized bone tissue. Cytotherapy 2013;15:1309–1322.
- Amini AR, Laurencin CT, Nukavarapu SP. Bone tissue engineering: recent advances and challenges. Crit Rev Biomed Eng. 2012;40:363–408.
- Zare H, Jamshidi S, Dehghan MM, et al. Bone marrow or adipose tissue mesenchymal stem cells: comparison of the therapeutic potentials in mice model of acute liver failure. J Cell Biochem. 2018;119:5834–5842.
- Ren Y, Wu H, Zhou X, et al. Isolation, expansion, and differentiation of goat adipose-derived stem cells. Res Vet Sci. 2012;93:404–411.
- Sancilio S, Gallorini M, Di Nisio C, et al. Alginate/hydroxyapatite-based nanocomposite scaffolds for bone tissue engineering improve dental pulp biomineralization and differentiation. Stem Cells Int. 2018;2018:1.
- Dubey NK, Mishra VK, Dubey R, et al. Revisiting the advances in isolation, characterization and secretome of adipose-derived stromal/stem cells. Int J Mol Sci. 2018;19:2200–2223.
- Shi S, Robey PG, Gronthos S. Comparison of human dental pulp and bone marrow stromal stem cells by cDNA microarray analysis. Bone 2001;29:532–539.
- Cui L, Liu B, Liu G, et al. Repair of cranial bone defects with adipose derived stem cells and coral scaffold in a canine model. Biomaterials 2007;28:5477–5486.
- Abudusaimi A, Aihemaitijiang Y, Wang YH, et al. Adipose-derived stem cells enhance bone regeneration in vascular necrosis of the femoral head in the rabbit. J Int Med Res. 2011;39:1852–1860.
- Sandor GK, Numminen J, Wolff J, et al. Adipose stem cells used to reconstruct 13 cases with cranio-maxillofacial hard-tissue defects. Stem Cells Translat Med. 2014;3:530–540.
- Prins HJ, Schulten EA, Ten Bruggenkate CM, et al. bone regeneration using the freshly isolated autologous stromal vascular fraction of adipose tissue in combination with calcium phosphate ceramics. Stem Cells Translat Med. 2016;5:1362–1374.
- Galler KM, Cavender A, Yuwono V, et al. Self-assembling peptide amphiphile nanofibers as a scaffold for dental stem cells. Tissue Engineering Part A. 2008;14:2051–2058.
- Botero TM, Son JS, Vodopyanov D, et al. MAPK signaling is required for LPS-induced VEGF in pulp stem cells. J Dent Res. 2010;89:264–269.
- Li Q, Li PH, Hou DJ, et al. EGF enhances ADSCs secretion via ERK and JNK pathways. Cell Biochem Biophys. 2014;69:189–196.
- Stanko P, Kaiserova K, Altanerova V, et al. Comparison of human mesenchymal stem cells derived from dental pulp, bone marrow, adipose tissue, and umbilical cord tissue by gene expression. Biomed Pap Med Fac Univ Palacky Olomouc Czech Repub. 2014;158:373–377.
- Gronthos S, Mankani M, Brahim J, et al. Postnatal human dental pulp stem cells (DPSCs) in vitro and in vivo. Proc Nat Acad Sci USA. 2000;97:13625–13630.
- Zuk PA, Zhu M, Mizuno H, et al. Multilineage cells from human adipose tissue: implications for cell-based therapies. Tissue Eng. 2001;7:211–228.
- Teresa LR, Sanchez-Abarca LI, Muntion S, et al. MSC surface markers (CD44, CD73, and CD90) can identify human MSC-derived extracellular vesicles by conventional flow cytometry. Cell Communication and Signaling: CCS 2016;14:2.
- Angelopoulos I, Brizuela C, Khoury M. Gingival mesenchymal stem cells outperform haploidentical dental pulp-derived mesenchymal stem cells in proliferation rate, migration ability, and angiogenic potential. Cell Transplant. 2018;27:967–978.
- Livak KJ, Schmittgen TD. Analysis of relative gene expression data using real-time quantitative PCR and the 2(-Delta Delta C(T)) Method. Methods (San Diego, Calif). 2001;25:402–408.
- Kustro T, Kiss T, Chernohorskyi D, et al. Quantification of the mandibular defect healing by micro-CT morphometric analysis in rats. J Cranio-Maxillo-Facial Surgery: Official Publication of the Euro Assoc Cranio-Maxillo-Facial Surg. 2018;46:2203–2213.
- Alkharobi H, Beattie J, Meade J, et al. Dental pulp cells isolated from teeth with superficial caries retain an inflammatory phenotype and display an enhanced matrix mineralization potential. Front Physiol. 2017;8:244.
- Wang S, Zhang Z, Xia L, et al. Systematic evaluation of a tissue-engineered bone for maxillary sinus augmentation in large animal canine model. Bone 2010;46:91–100.
- Kaigler D, Pagni G, Park CH, et al. Stem cell therapy for craniofacial bone regeneration: a randomized, controlled feasibility trial. Cell Transplant. 2013;22:767–777.
- Radunovic M, De Colli M, De Marco P, et al. Graphene oxide enrichment of collagen membranes improves DPSCs differentiation and controls inflammation occurrence. J Biomed Mater Res. 2017;105:2312–2320.
- Li Y, Zhao S, Nan X, et al. Repair of human periodontal bone defects by autologous grafting stem cells derived from inflammatory dental pulp tissues. Stem Cell Res Ther. 2016;7:141.
- d’Aquino R, De Rosa A, Lanza V, et al. Human mandible bone defect repair by the grafting of dental pulp stem/progenitor cells and collagen sponge biocomplexes. ECM. 2009;18:75–83.
- Bora P, Majumdar AS. Adipose tissue-derived stromal vascular fraction in regenerative medicine: a brief review on biology and translation. Stem Cell Res Ther. 2017;8:145.
- Zhang X. Intravital imaging to understand spatiotemporal regulation of osteogenesis and angiogenesis in cranial defect repair and regeneration. Meth Mol Biol (Clifton, NJ) 2018;1842:229–239.
- Chim SM, Tickner J, Chow ST, et al. Angiogenic factors in bone local environment. Cytokine & Growth Factor Rev. 2013;24:297–310.
- Hilkens P, Fanton Y, Martens W, et al. Pro-angiogenic impact of dental stem cells in vitro and in vivo. Stem Cell Res. 2014;12:778–790.
- Preisner F, Leimer U, Sandmann S, et al. Impact of human adipose tissue-derived stem cells on malignant melanoma cells in an in vitro co-culture model. Stem Cell Rev and Rep. 2018;14:125–140.