Abstract
To evaluate the safety and efficacy of novel cobalt complex with sulindac (Co-SLD), the zebrafish and oral squamous cell carcinoma CAL27 were investigated in the present study. The developmental toxicity of Co-SLD ranging from 5 to 20 μM was determined by exposure to 3–144-h post-fertilization (hpf) zebrafish. Our data showed that Co-SLD did not cause to the appreciable toxicity at low concentration (5 and 10 μM). A remarkable toxicity was observed at high concentration (20 μM), including increased mortality and malformation, delayed hatchability, reduced heart rate as well as suppressed behaviour. With regard to the antitumor activity of Co-SLD, inhibited cell growth and migration capability were outstandingly observed in oral squamous cell carcinoma treated with 10 and 20 μM Co-SLD, which could be mainly attributed to the Co-SLD-elicited mitochondrial damage as marked by the depression of mitochondrial membrane potential, ROS accumulation and ATP depletion. Furthermore, administration of 10 μM Co-SLD was an optimal concentration not only to avoid the normal tissue toxicity, but also to enhance the killing of cancer cells via disrupting mitochondrial dysfunction. Taken together the above results demonstrated the desirable response of oral squamous cell carcinoma to Co-SLD.
Introduction
Head and neck cancer, the sixth most common type of cancer in the world, has recently manifested a dramatic increase in the West [Citation1]. More than 50% of patients have recurrence within 3 years [Citation2], and the median overall survival for recurrent or metastatic head and neck cancer is 6 to 12 months [Citation3]. There are, however, limited treatment options for these patients with the recurrent and/or metastatic situation, and chemotherapy is indicated [Citation4]. Of note, platinum-based therapy such as platinum in combination with 5-fluorouracil and cetuximab is currently given as first-line regimen for recurrent and/or metastatic head and neck cancer [Citation5,Citation6]. But platinum-based regimens have a dismal prognosis and increase toxicity [Citation7,Citation8]. Furthermore, tumours often relapse with drug resistance and eventually promoted metastatic potential [Citation9,Citation10]. Thus, it is in urgent need to seek new high-selective and low-toxic anticancer drugs to generate the therapeutic diversity.
Currently, the development of metal complexes with non-platinum central atoms including ruthenium [Citation11], gold [Citation12], silver [Citation13], copper [Citation14], zinc [Citation15], Manganese [Citation16], nickel [Citation17], iridium [Citation18] and cobalt [Citation19] has been one of the hottest emerging areas of anticancer drug research. Among these, carbonyl cobalt complex has strong properties of cancer prevention including tumour growth/metastasis suppression [Citation20], antiproliferative [Citation21], DNA cleavage [Citation22], apoptotic induction [Citation23] and autophagy [Citation8], which was more active than cisplatin on the human breast tumour cell lines (MCF-7 and MDA-MB-231) [Citation24,Citation25] and ovarian cancer cells (SKOV3/DDP) [Citation26]. With the concept of carbonyl metal CO-releasing molecules (CORMs) appearing, the anti-inflammatory effects were found through liberating CO to inhibit nitrite content [Citation27]. Moreover, the CO released from the cobalt carbonyl complexes was also discovered to block the tumour angiogenesis [Citation28], disrupt tumour cell energy metabolism [Citation29] and modulate the immune system [Citation30].
Anticancer activities of cobalt complexes were closely connected with the structure of the ligand, and a non-steroidal drug structure as one of them can enhance the anticancer efficacy of the compound [Citation31]. It is now generally accepted that the inflammatory response is one of the predisposing conditions for carcinogenesis process [Citation32]. Sulindac, a non-steroidal anti-inflammatory drug, resulted in inhibiting the proliferation of tumour cells, and its mechanism was mainly through inhibition of cyclooxygenase-2 (COX-2) [Citation33,Citation34]. COX-2, an inducible isoform of cyclooxygenase, up-regulated in head and neck cancer is involved in the development and progression of cancers including growth, metastasis and prognosis [Citation35,Citation36]. As previously mentioned, inhibition of COX-2 pathways may have an evident implications for the prevention of both premalignant lesions and metastatic potential of head and neck squamous cell carcinoma [Citation37]. Furthermore, COX-2 overexpression is also a consequence of promoted drug resistance [Citation38].
In this present study, we synthesized a hybrid CORM derived from sulindac (Co-SLD). The enhanced antitumour effect of Co-SLD on oral squamous cell carcinoma, a type of head and neck cancer located in the oral cavity, was investigated for the first time, and its underlying mechanism was preliminarily explored in vitro. At the same time, zebrafish (Danio rerio), a valuable and cost-effective vehicle to predict toxicity of human drugs [Citation39], was used to assess the security of Co-SLD.
Materials and methods
Synthesis of the intermediate
A method for the synthesis of the intermediate was performed as in our previous study [Citation40]. 0.15 mmol 4-dimethylaminopyridine (DMAP) and 1.2 mmol 1-ethyl-3- (3-dimethylaminopropyl) carbodiimide hydrochloride (EDCI) were added to the acetonitrile solution containing 1.0 mmol sulindac and reacted at room temperature for 0.5 h. And then, 1.0 mmol propargyl alcohol was added to the reaction solution and continued to stir at room temperature for 4 h. After the reaction was terminated, the filtrate was collected by filtration, and the solvent in the filtrate was removed under reduced pressure. The residue was dissolved in chloroform, washed with water and dried over anhydrous Na2SO4, followed by filtration, and the solvent was removed under reduced pressure. The crude product was purified by column chromatography (eluent: ethyl acetate/n-hexane = 1:20) to obtain a pale yellow solid (289 mg, 73.1%). 1 H NMR (400 MHz, Chloroform-d) δ 7.76–7.51 (m, 4H), 7.17 (s, 1H), 7.10 (s, 1H), 6.81 (dd, J = 8.9, 2.4 Hz, 1H), 6.50 (td, J = 8.8, 2.5 Hz, 1H), 4.64 (d, J = 2.5 Hz, 2H), 3.55 (s, 2H), 2.74 (s, 3H), 2.42 (t, J = 2.5 Hz, 1H), 2.14 (s, 3H). ESI-MS (m/z): calcd. for C22H20FO3S [M + H]+: 395.1117; found 395.1125, C22H20FO3SNa [M + Na]+: 417.0937; found 417.0945.
Synthesis of the complex Co-SLD
Co-SLD was prepared using our previous reported methods [Citation40]. 1.2 mmol dicobaltoctacarbonyl was added to the THF solution containing 1.0 mmol intermediate, and the reaction was carried out overnight at room temperature. After the reaction, the solvent was evaporated under reduced pressure and the crude product was separated by column chromatography (eluent: ethyl acetate/n-hexane = 1:5) to give the product as a red powder (397 mg, 58.4%). IR (KBr disk, cm−1): 2096vs, 2063vs, 2034vs, 2010vs (Co-CO), 1720 s (COO). 1 H NMR (400 MHz, Chloroform-d) δ 7.76– 7.48 (m, 4H), 7.21 (s, 1H), 7.14 (s, 1H), 6.82 (dd, J = 8.9, 2.4 Hz, 1H), 6.50 (td, J = 8.8, 2.5 Hz, 1H), 5.96 (s, 1H), 5.26(s, 2H) 3.56 (s, 2H), 2.78 (s, 3H), 2.16 (s, 3H). 13 C NMR (101 MHz, Chloroform-d) δ197.9, 170.8, 162.1, 147.2, 145.1, 141.9, 136.3, 130.1, 129.4, 127.7, 125.5, 123.5, 112.2, 71.7, 65.2, 54.7. ESI-MS (m/z): calcd. for C29H20FCo2O9S [M + H]+:680.9476; found 680.9558.
Measurements of CO release
The ability of CO release from CORM was determined by a myoglobin (Mb) assay for spectrophotometric assessment of the conversion of deoxy-myoglobin (deoxy-Mb) to carbonmonoxy-Mb (Mb-Co), accordingly [Citation40,Citation41]. The total amount of liberated CO was calculated by monitoring the changes in absorbance at 540 nm. A stock solution of myoglobin (Sigma) was freshly diluted with phosphate-buffered saline to a final concentration (60 μM). Sodium dithionite (0.1%) was added into the above solution to generate deoxy-Mb, which was assayed to obtain a deoxy-Mb spectrum and then bubbled with CO to get an Mb-Co spectrum. Co-SLD and deoxy-Mb solution were mixed and overlaid with 500 μL light mineral oil to prevent CO leaking or the myoglobin binding oxygen. The absorption peak of deoxy-Mb at 560 nm was replaced by the two peaks of Mb-Co at 540 and 578 nm. The concentration of myoglobin in the stock solution was calculated from the absorption maximum of the Mb-Co solution at 540 nm.
Zebrafish husbandry and embryo harvesting
Zebrafish (Danio rerio) were bred in a recirculating system according to standard operating procedures, as published previously [Citation42]. Wild-type AB strain zebrafish were maintained under the following conditions: a 14-h light/10-h dark cycle, a constant water temperature at 28 ± 1 °C, a pH around 7 and a conductivity range of 450–500 μS. Freshly hatched brine shrimp was fed to fish twice per day (Brine Shrimp Direct, Ogden, Utah, USA).
Adult fish with a sex ratio of 1/2 (female/male) mated at the start of the light-induced spawning in embryo collection tanks. Fertilized embryos were rinsed with E3 culture medium (5 mmol/L sodium chloride (NaCl), 0.33 mmol/L calcium chloride (CaCl2), 0.33 mmol/L magnesium sulphate heptahydrate (MgSO4) and 0.17 mmol/L potassium chloride (KCl)) and then incubated at 28.5 °C. At 3-h post-fertilization (hpf), healthy developing embryos were selected for the further experimental. All the experimental manipulations were conducted in accordance with the guidelines on the Humane Treatment of Laboratory Animals stipulated by the Ministry of Science and Technology (MOST) of the People's Republic of China.
Chemical exposure and experimental design
Embryos exposed to 5, 10 and 20 μM Co-SLD from 3 to 144 post-fertilization (hpf) of zebrafish development. Fresh Co-SLD medium was added again every 24 h up to 144 hpf. Three repeated experiments were separately carried out for each separate experiment. Embryos with healthy development were divided into five groups as follows: (a) the control group, which received only treated with E3; (b) DMSO group, which administrated with DMSO at 3 hpf; (c–e) three Co-SLD treated groups, which exposed to 5, 10 and 20 μM Co-SLD at 3 hpf.
Cell line and culture
The human tongue squamous cell carcinoma cell line (CAL27) was obtained from BeNa Culture Collection (BNCC, Beijing, China). Cells were cultured in DMEM containing 10% foetal bovine serum at 37 °C in a humidified atmosphere of 5% CO2. Adherent cells monolayer was grown to 80%–90% confluence and then passaged using 0.05% trypsin digestion solution.
COX-2 activity assay
CAL27 cells were treated with Co-SLD, at concentrations of 0, 5, 10 and 20 μM for 24 h. After that, the content of PTGS/COX2 was detected by enzyme-linked immunosorbent kits (MEIMIAN, Jiangsu, China), according to manufacturer instructions. Triplicates were included in each sample, and experiments were repeated three times.
Mortality, hatching rate and malformation
The toxicity of Co-SLD was monitored by mortality, hatching rate and malformation rate in the developing zebrafish embryos, following the same methodology used previously [Citation42]. At 120 hpf, mortality was evaluated as the percentage of dead embryos out of the total embryos. Owing to embryogenesis completes by 72 hpf [Citation43], the hatching rate was recorded at 72 hpf and calculated as the number of embryos hatched from survived embryos. Likewise, malformation was screened out at 72 hpf, and the percentage of malformed embryos out of living embryos were counted. The representative morphology with abnormal embryos after anaesthesia with 0.01% MS 222 (Sigma, USA) was photographed under a stereoscopic dissecting microscope at 30× magnification (Motic, SMZ-161, Motic China Group CO., Ltd., China). One hundred embryos were assigned for each group.
Spontaneous movement, heart beat and behavioural assay
Spontaneous movement and heart beats were recorded at 24 hpf and 48 hpf, respectively, using an stereoscopic dissecting microscope (Motic, SMZ-161, Motic China Group CO., Ltd., China) and Media Cruiser recording software (Canopus Corporation, Kobe, Japan), as previously described [Citation44]. At 144 hpf, 12 healthy larvae were selected randomly to examine the influence of Co-SLD to the nervous system by evaluating swimming ability according to the method of Si et al [Citation42]. The locomotor activity of larvae was evaluated using Noldus tracking device (Noldus Information Technology, Wageningen, Netherlands) at a temperature of 28 °C for 20 min to reduce any disturbance. And then the locomotor activities were recorded during 30-min light-to-dark (5 min for each period) transition stimulation. The data for spontaneous movements, heart beats and locomotor activities were analysed using EthoVision XT 10.0 software (Noldus Information Technology, Wageningen, Netherlands).
Growth inhibitory analysis
The contentious cellular proliferation was analysed with an iCELLigence RTCA system (ACEA Bioscience, San Diego, CA, USA) in the presence or absence of Co-SLD. CAL27 cells were harvested 24 h after different treatments and seeded into an E-8-well plate at a density of 5 × 103 cells/well. Changes in cell survival rate were monitored and quantified by detecting sensor electrical impedance. The sensor devices were placed into the 5% CO2 incubator, and the cell index value was determined every hour automatically for up to 96 h, as previously noted [Citation45]. Ki67 monoclonal antibody (Abcam, San Francisco, CA, USA) as a proliferation marker was detected by fluorescent staining. The images were captured by a confocal laser microscope (LSM, Carl Zeiss AG, Germany).
Colony-formation assay
To evaluate the long-term effect of Co-SLD on cell growth, colony-forming ability was performed in CAL27 cells with or without Co-SLD. The cells were seeded into 60 mm in triplicate dishes and were treated with different concentrations of Co-SLD for 24 h. After incubated at 37 °C for 14 days, colonies were fixed with chilled methanol and stained with 0.4% crystal violet. Colonies containing ≥50 cells were counted, and cloning-forming efficiency was determined.
Cell cycle assay
CAL27 cells were treated with different concentrations of Co-SLD to perform using cell cycle staining kit (MultiSciences, Hangzhou, China) according to the manufacturer’ s protocols. After 24 h, they were harvested and fixed with 70% ice-cold ethanol overnight at –20 °C. The fixed cells were centrifuged at 1000 rpm for 5 min and washed three times with PBS, and then stained with propidium iodide (PI) in the dark for 30 min at room temperature. The samples were detected with BD Accuri C6 (Becton Dickinson, San Jose, CA, USA) for cell cycle distribution.
Apoptosis analysis
Apoptosis in living embryos was detected with acridine orange (AO) staining at 48 hpf according to the method of Zhou et al. [Citation46]. Apoptosis was detected in the CAL27 cells by the commercial kit (BD Biosciences, San Jose, CA, USA) according to the manufacturer’s protocol. Unstained cells and cells stained with FITC-Annexin V or with PI alone were used to set up compensation and quadrants. The cell population of apoptotic/necrotic was assessed with a Flowsight imaging flow cytometer (Amnis/Merck Millipore, Darmstadt, Germany).
Wound healing assay
In vitro wound assays were performed using IBIDI culture-inserts (ibidi, Martinsired, Germany). The CAL27 were seeded at 1 × 104 cells and incubated in culture medium until full confluence was attained. Cells were incubated in serum-free medium for up to 48 h, and then the silicon reservoir insert was gently removed with sterile forceps. Cells migrated into the wounded area, and photographs were taken immediately (0 h), 6 h, 12 h, 24 h and 48 h by using an optical microscopy. The wound area was measured and analysed by the ImageJ software (National Institute of Health, USA).
Mitochondrial membrane potential assay
Changes in mitochondrial membrane potential (Δψm) after Co-SLD treatments were studied by staining with the JC-1 dye. In healthy cells with high Δψm, JC-1 accumulates in the mitochondria as red J-aggregates in the mitochondrial matrix. When the Δψm collapses, JC-1 exists as a green monomer in the cytoplasm. CAL27 cells were incubated with 10 mg/mL JC-1 (Sigma-Aldrich Biotechnology, St. Louis) for 20 min at room temperature. Representative images were captured by fluorescence microscopy (Olympus, Tokyo, Japan). The ratio of red-to-green fluorescence as the extent of a collapse in the Δψm was measured by a fluorescence microplate reader (Tecan Infinite M200, Austria).
ROS detection
According to our previous method [Citation47], ROS yield was measured fluorometrically using the Amplex Red, a highly sensitive and stable probe for H2O2. After exposure to different Co-SLD concentrations, CAL27 cells were replenished with serum-free DMEM medium without phenol red and supplemented with 100 μL of working solution containing 5 μM of Amplex Red reagent and 2 U/mL of horseradish peroxidase. After incubation for 30 min at 37 °C in the dark, fluorescence intensity was measured by a microplate reader (Tecan Infinite M200, Austria) with an excitation/emission at 560 nm/590 nm, respectively.
Adenylates determination
Cellular ATP, ADP and AMP after Co-SLD treatments were determined by HPLC analysis as previously described [Citation48]. The HPLC conditions were as follows: a reverse phase C18 column (Agilent Technologies, Santa Clara, CA, USA); a mobile phase containing 35 mM NaH2PO4, 6 mM tetrabutylammonium; mobile phase: acetonitrile gradient 95:5 to 75:25. Peak areas were detected at 210 nm for 0–10 min. The analytical concentrations of adenylates were identified by comparison of the area of the sample with that of standards (Beijing Solarbio Science & Technology Co., Ltd, China).
Statistical analysis
Three independent experiments were performed. All quantitative data were expressed as the mean ± SEM. Statistical analysis was done with SPSS 16.0 (SPSS Inc., Chicago, IL, USA). Comparisons between two groups were completed with one-way analysis of variance (ANOVA) followed by LSD post hoc test. A value of P < .05 was considered statistically significant. Most graphs were plotted using Origin 7.5 software (OriginLab, Northampton, MA, USA).
Results and discussion
Syntheses and properties of Co-SLD
As shown in , Co-SLD was prepared through the addition reaction of Co2 (CO) 8 and intermediate which was synthesized by the reaction of between organic acids with sulindac and propargyl alcohol. Co-SLD was characterized by NMR, ESI-MS and IR spectroscopy. Co-SLD easily dissolves in organic solvent. IR spectra data showed that several strong terminal carbonyl absorptions appeared in the range of 2096–2010 cm−1. Higher absorption bands were attributed to terminal carbonyls, which is in accordance with the Co atom. The corresponding ester carbonyl absorptions occurred at 1744 cm−1 for the Co-SLD. 1 H NMR spectra of the Co-SLD found that the chemical shift of the benzene ring protons was observed at 6.50 ppm, signal at 5.26 ppm as singlet assigned to hydrogen of OCH2 in Co-SLD.
Figure 1. Syntheses and properties of Co-SLD. (A) The structure and synthetic route for Co-SLD. (B) The typical changes in the electronic spectrum of myoglobin as CO releasing from compounds (myoglobin binds one CO to form carbonyl myoglobin). (C) The effect of compound concentration on COX-2 in CAL27. Each value is expressed as the mean ± SEM (n = 3). **p < .01 and ***p < .001 compared with the control group.
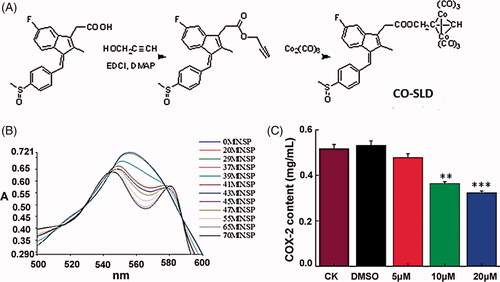
[Co2 (CO) 6] is a source of CO, and the ability of CO release was monitored in a myoglobin-based aqueous assay by UV–Vis spectroscopy. To evaluate both the total amount of CO released and the rate of CO release, CO-SLD was measured at 10 μM by following the alterations in absorption. The UV spectrum and the concentration of CO-Mb as the variations of time were observed in . The half-life (t1/2) of Co-SLD was 34.7 min, indicating a slow release of CO from Co-SLD. Compared with CORMs based on ruthenium [Citation49], the rate of CO release of Co-SLD made CO liberating slower. The rate of CO release was considered to depend on the structures of non-CO ligands [Citation50].
To explore the relationship between the modulation of COX-2 expression and the antitumour activity of Co-SLD, the level of COX-2 in CAL-27 cells was further investigated. As illustrated in , Co-SLD significantly displayed lower activity to inhibit COX-2 expression level at 10 μM (P < .01) and 20 μM (P < .001) with respect to the control group, but when the administration of Co-SLD with 5 μM, alteration in the COX-2 activity was very slight.
Embryo development toxicity of Co-SLD in vivo
Zebrafish are a vertebrate model that they have transparency of the embryos, large numbers of eggs per spawn and rapid development, which can therefore serve as an animal model for high-throughput toxicity tests [Citation51]. To assess the possible toxicity of Co-SLD on zebrafish embryos, here we detected the mortalities, hatchability and malformations after administration of Co-SLD in 3 hpf zebrafish embryos at concentrations of 5, 10 and 20 μM. The results showed that treatment with 5 and 10 μM of Co-SLD had no appreciable effect on mortality, hatching rate and morphological abnormality measured at 24 and 72 hpf, respectively ().
Figure 2. Embryo development toxicity caused by Co-SLD in vivo. (A) The mortality rate of zebrafish embryos with different treatments. (B) The hatchability of zebrafish embryos with different treatments. (C) Morphological abnormalities of zebrafish with the different treatments. PE: pericardial oedema; YSE: yolk sac oedema; SB: spine flexion; TD: tail deficiency. Each value is expressed as the mean ± SEM (n = 3). **p < .01 and ***p < .001 compared with the control group.
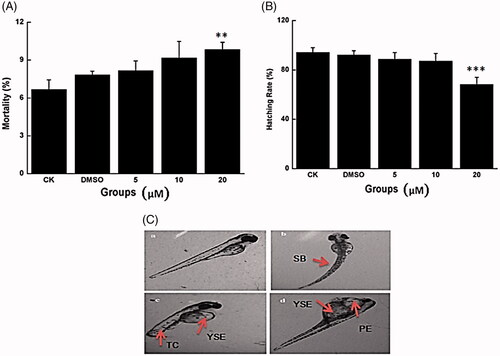
At the higher dose (20 μM), compared with the control group, approximately 9.8% of the embryos died at 24 hpf when treated with Co-SLD; the hatching rate decreased to 68.3% at 72 hpf when incubated with Co-SLD. Meanwhile, Co-SLD caused various malformations in zebrafish embryos at 72 hpf, mainly including pericardial oedema, yolk sac oedema, tail deficiency and spinal curvature. These typical malformations also apparently occurred in embryos cultured in cobalt [Citation52,Citation53]. Among these, pericardial/yolk sac oedema was the most common type in the Co-SLD-treated group. In summary, exposure to Co-SLD caused a dose-dependent embryonic developmental toxicity. Song et al. revealed that ruthenium-containing carbon monoxide-releasing molecules (CORM-3, >25 μM) exhibited a serious embryotoxicity of zebrafish [Citation54]. However, when the zebrafish were supplemented with lower concentration of 10 μM, CORM-3 exerted the protective impacts against lethal and sublethal developmental toxicity induced by irradiation, which contributed to a low concentration CO release from CORM-3 [Citation55].
Cardiotoxicity evaluation of Co-SLD in vivo
The heart is one of the first functional organs to develop in zebrafish embryos [Citation56]. Apoptosis plays a critical role in maintaining tissue homeostasis under physiological conditions and is an important component in developmental programs [Citation57]. The level of apoptosis in zebrafish embryos is commonly performed by staining with the vital dye AO which is a nucleic acid intercalating dye that permeates dying cells to bind chromatin and emits green fluorescence when bound to dsDNA [Citation58].
In the current study, we investigated the occurrence of apoptosis in whole embryos at 48 hpf using AO staining. As seen in , distinct apoptotic cells expressed as bright dots under AO staining. When embryos were exposed to Co-SLD at a dose of 20 μM, large numbers of cells underwent apoptosis, whereas there were few AO-positive cells in the control, DMSO, 5, and 10 μM treatment groups. Most importantly, our data showed apoptosis signal of embryos focusing on the developing zebrafish heart treated with Co-SLD, suggesting a remarkable cardio-toxicity caused by higher concentration of Co-SLD, which at least attributed to the myocardial toxicity of COX-2 inhibitors [Citation59]. Emerging evidence has also highlighted that very low level of myocyte apoptosis is sufficient to give rise to a lethal, dilated cardiomyopathy [Citation60]. Here, heart rate as an important indicator of cardiac function was measured in zebrafish providing a reliable metric for quantifying the developmental toxicity of Co-SLD. Concentrations of 5 and 10 μM of Co-SLD had little effects on the heart rate. However, the heart rate was significantly slower in the 20 μM Co-SLD group than that of the control group (, P < .01).
Figure 3. Changes in cardiotoxicity induced by Co-SLD at 48 hpf embryos. (A) Typical fluorescence microscope of live zebrafish embryos measured using AO staining. Apoptosis cells were identified as green punctate dots on a black background and indicated by white arrows. (B) The heart rate at 48 hpf zebrafish with the different treatments. Each value is expressed as the mean ± SEM (n = 3). *p < .05 compared with the control group.
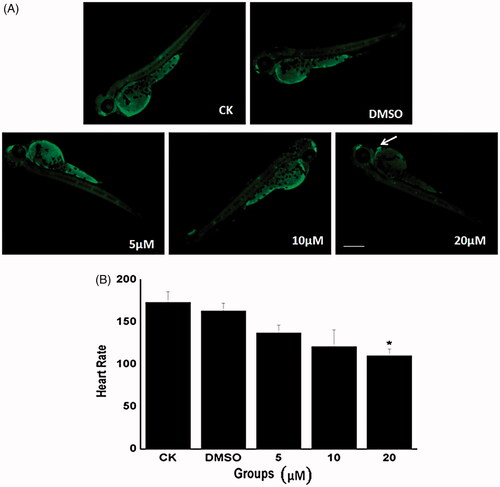
Alteration of embryos/larval locomotor activity f Co-SLD
We next verified the effects of Co-SLD as determined by behaviour assessment of zebrafish embryos treated with different concentrations of Co-SLD. Behavioural analysis often serves as a sensitive tool to detect the sub-lethal effects of chemicals [Citation61]. The spontaneous movements and swimming distances were measured at 24 and 144 hpf, respectively. During early zebrafish embryogenesis, spontaneous coiling contractions represent the first indications of locomotion, followed by the emergence of twitching responses to touch, and later by the ability to swim [Citation62]. As previous studies have concluded that spontaneous activity occurs between approximately 19 and 29 hpf, spontaneous movement of zebrafish embryos was recorded at 24 hpf [Citation63]. Spontaneous movement of zebrafish embryos was detected as mean duration. Compared with the control group, there was a slight decrease following 5 or 10 μM administration of Co-SLD and was a significant suppression following 20 μM treatment ((P < .001), ). A lower spontaneous movement may be associated with developmental delay in zebrafish embryos [Citation64].
Figure 4. Behavioural assessment with the increasing concentrations of Co-SLD treatments. (A) Spontaneous movement at 24 hpf zebrafish embryos (B) Swimming distances of larvae after a 30-min light-to-dark photostimulation at 144 hpf. Light and dark periods are denoted by white and dark bars at the bottom. Each value is expressed as the mean ± SEM (n = 3). *p < .05, **p < .01 and ***p < .001 compared with the control group.
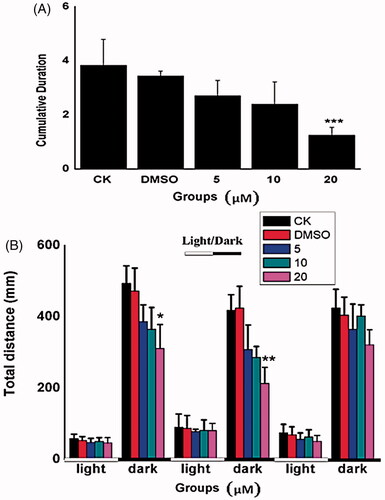
In response to light-to-dark transition stimulation, the larvae exhibited lower locomotive behaviour during the bright phase and a rapid increase during the dark period because of the dark-avoidance behaviour [Citation65]. Light stimulation from bright to dark can cause circumvention in the larvae, resulting in a rapid increase in locomotive behaviour in the dark period. In the present study, we found that there was no significant difference locomotive behaviour during visible light in the case of all treatment groups. However, embryos added with 20 μM Co-SLD showed significant reductions of swimming distances in the first (p < .05) or second (p < .01) dark phase at 144 hpf, respectively, when compared with the controls (). These data indicated that larvae response of developing nervous system particularly vulnerable to higher dose of Co-SLD. The behavioural decline may be partially due to the larval malformation from the nervous system and skeletal muscles, which are important factors involved in the overall locomotive behaviour [Citation66]. Similarly, Gong et al. have demonstrated that larval locomotor activity exposed to the CO-releasing molecules containing cobalt also was concentration-dependently decreased [Citation41].
Inhibition of cell growth induced by Co-SLD
It has been well reported that expression of Ki-67 antigen was higher (70%) in OSCC patient [Citation67]. A strong positive Ki67 signature visually occurred in the control group. Whereas, 24 h after supplement with Co-SLD at the doses of 10 and 20 μM, the florescence accumulation of Ki67 was dramatically attenuated in CAL 27 cells. Consistent with our findings, a previous study showed carbonyl cobalt CORMs with selectively inhibiting cyclooxygenase-2 also exhibited the better antiproliferative effects to HeLa cell and HT-29 cell lines [Citation40].
To validate the long-time effect of cell growth, cell index (CI) value was determined for up to 96 h in oral squamous cell carcinoma CAL27 cells treated with Co-SLD using real-time cell analysis (RTCA) system. As described in , there was a dose- or time-dependent suppression of survival of CAL27 cells induced by Co-SLD varying from 5 to 20 μM. KT50 (the amount of time it takes to kill 50% of the target tumour cells) was selected as a quantitative parameter for evaluating the efficient killing kinetic of Co-SLD [Citation68]. KT 50 values were roughly 63, 41 and 13 h, in CAL27 cells exposed to 5, 10, and 20 μM, respectively. After 14 d, the colony-forming assay displayed that the number of colonies formed was vastly inhibited by all concentrations of Co-SLD, with a dose-dependent manner (). At the highest concentration (20 μM), colony formation was reduced over 90% related to the control group. These indicated that Co-SLD at all concentrations displayed the superior killing effects in a sufficient time.
Figure 5. Inhibition of CAL27 cell growth of Co-SLD. (A) Capture images of Ki67 as a proliferation mark with a fluorescent confocal microscope. (B) Monitors of cell growth up to 96 h using the RTCA system. (C–D) Representative images of the colony formation and quantitative colony counts. Each value is expressed as the mean ± SEM (n = 3). ***p < .001 compared with the control group.
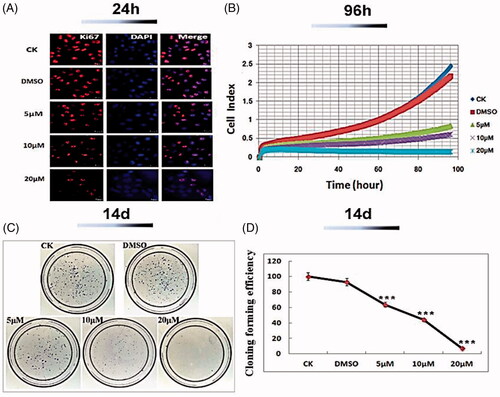
Changes of cell cycle arrest and apoptosis
To shed more light on the mechanism of the effect of Co-SLD of CAL27 cell growth, the cell-cycle distribution was examined using flow cytometry. Our data revealed that the percentage of cells in G1 phase was notably increased in CAL27 cells at 24 h after 5, 10 and 20 μM exposure of Co-SLD as compared with the control group, as can be seen in . Particularly, a peak fraction of cells in G1 phase was observed in the group of 10 μM Co-SLD (63.8%). These implied that Co-SLD could affect tumour growth and cellular proliferation through mediation of cell cycle.
Figure 6. Alterations of cell cycle and apoptosis induced by Co-SLD. (A) Analysis of cell cycle distribution of CAL-27 cells in G1, S and G2 cell cycle stage. (B) Percentage denotes the proportion of apoptotic cells in right lower quadrant and right upper quadrant. Each value is expressed as the mean ± SEM (n = 3). *p < .05 and ***p < .001 compared with the control group.
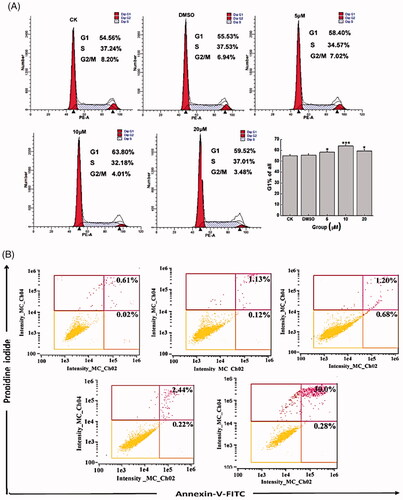
To further validate whether the decreased cell viability of CAL27 cells pretreated with Co-SLD was associated with apoptosis, CAL 27cells were treated with different concentrations of Co-SLD (5, 10 and 20 μM) followed by annexin V-FITC/PI staining to examine the proportion of apoptotic cells at 24-h post-administration. As seen in , the treatment of CAL27 cells with 5 μM Co-SLD for 24 h showed no significant increase in apoptotic cell fraction (1.88%) compared to the control group (0.63%). When Co-SLD was 10 or 20 μM, the proportion of apoptotic cells was up to 2.66% or 32.8%. Co-SLD at 20 μM not only obviously inhibited cell proliferation, but also elicited a significantly increasing amount of cell apoptosis in CAL27 cells. However, cells were treated for 24 h with 10 μM Co-SLD promptly inhibited the cell growth (39.8% reduction compared to the control group), but apoptosis was not very remarkable. This result demonstrated that the growth inhibition in 10 μM Co-SLD treatment was not fully apoptosis-dependent, which was closely involved in control of G1-phase cell arrest and S-phase inactivation at this time point, leading to a cessation of cell cycle progression [Citation69]. Based on the above exposition, Co-SLD could disrupt the balance of proliferation and cell death.
Co-SLD-induced cell migration
The increased migration and invasion of oral squamous cell carcinoma cells are crucial events in the development of metastasis to the lymph nodes and distant organs [Citation70]. To investigate the effects of Co-SLD on the metastatic potential in oral squamous cell carcinoma cells, the migration assay was performed at different time points using scratch wound healing. As exhibited in , there were no obvious alterations caused by Co-SLD treatments in the scratch area at time point 0, 6 and 12 h relative to the control group. After supplement with Co-SLD (5, 10 and 20 μM), to some extent the capability of migration was declined compared to the control group in all cases, but a distinct change was observed in the Co-SLD-treated group at 48 h. After administrations with 10 μM and 20 μM Co-SLD, cells were migrated relatively less, with wound area more than 85.7% and 86.7%, relative to the control group completely covered the cell-free area at 48 h. There is positive of relevance between overexpression COX-2 and tumorigenesis or poor prognosis in patients with squamous cell carcinoma [Citation71]. These results indicated that Co-SLD significantly blockaded the metastatic process of oral squamous cell carcinoma cells, which partially attributed to CO derived from CORMs containing cobalt [Citation72] and sulindac and its derivatives [Citation73].
Modulation of mitochondrial dysfunction of Co-SLD
To better understand the mechanisms of Co-SLD for improving the antitumour activity in oral squamous cell carcinoma cells, mitochondrial membrane potential (Δψm) was assessed by immunofluorescence. The fluorescence micrographs in clearly showed membrane potential of mitochondria in CAL27 cells. With increasing concentrations of Co-SLD at 5, 10 and 20 μM, the ΔΨm dependent red and green fluorescence intensities in cells were altered when compared with control cells. Particularly, 10 μM- or 20 μM-treated groups exhibited a weak red fluorescence and a distinct green fluorescence, suggesting a loss of Δψm. As displayed in , average red/green ratios of Co-SLD at 10 and 20 μM were roughly 26% and 40% reduction with respect to the control group, separately. Owing to its close association with the leakage of electron transport during the decline of Δψm, ROS generation seemed reasonable [Citation74,Citation75]. As we expected, the sharp increases in ROS were also found in Co-SLD-treated cells at 10 and 20 μM (). CO liberated from Co-SLD had a high affinity to cytochrome c and NAD (P) H oxidase to regulate the levels of ROS. Disproportional increment in intracellular ROS can damage DNA, proteins, lipids, and other macromolecules, and subsequently trigger the senescence and apoptosis [Citation76]. Collectively, Co-SLD induced a dissipation of the Δψm that is accompanied by the accumulation of ROS.
Figure 8. Modulation of mitochondrial function by Co-SLD. (A–B) Average ratios of fluorescence amplitudes measured in the red and the green fluorescence channel, as expressed by red/green ratios. (C) Statistical analysis for ROS generation by Amplex Red. (D) The levels of AMP, ADP, and ATP as a function of energy charge were determined by HPLC. Each value is expressed as the mean ± SEM (n = 3). *p < .05, **p < .01 and ***p < .001 compared with the control group.
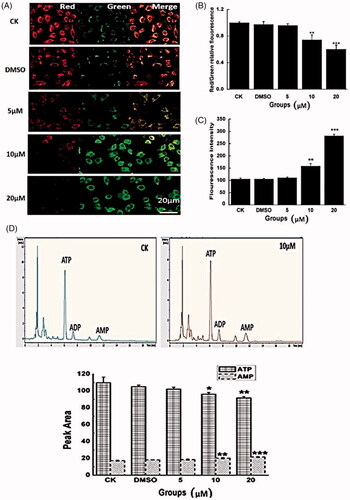
In order to further investigate mitochondrial function, mitochondrial energy metabolism was carried out in oral squamous cell carcinoma cells using the adenylates ATP, ADP and AMP profile test. The results illustrated in , treatment with Co-SLD at 10 and 20 μM significantly increased the AMP peak areas and markedly decreased the ATP peak areas compared with those of the control group. These indicate that Co-SLD impeded the energy supply. As we know, maintenance of the mitochondrial membrane potential is fundamental for a high energy requirement [Citation77]. As a result, diminished energy production in turn affected the maintaining of Δψm. Generally, mitochondrial dysfunction triggered by Co-SLD was characterized by the concurrent breakdown of the membrane potential and high ROS production as well as ATP depletion, and eventually formed a vicious cycle.
Conclusions
To estimate safety and efficacy of novel Co-SLD, the zebrafish as in vivo model and oral squamous cell carcinoma CAL27 as in vitro model were used in the present study. The developmental toxicity of Co-SLD in range of 5–20 μM was determined during 3–144 hpf of zebrafish. Our data showed that Co-SLD did not give rise to the appreciable toxicity at low concentration (5 and 10 μM), but remarkably led to the toxicity at 20 μM, as reflected by increased mortality and malformation, delayed hatchability, triggered cardiac cell apoptosis, reduced heart rate and spontaneous movement, as well as suppressed larval behaviour. As for the antitumor activity of Co-SLD, cell growth and migration were profoundly restrained in oral squamous cell carcinoma treated with 10 and 20 μM Co-SLD. Co-SLD-elicited mitochondrial dysfunction provided a vital interpretation to kill cancer cells, as marked by the depression of mitochondrial membrane potential, ROS accumulation and ATP depletion. Taken together, the above results demonstrated a considerable inhibition effect of Co-SLD on the oral squamous cell carcinoma. Furthermore, because of a very low degree of toxicity to normal animal, administration of 10 μM Co-SLD was a suitable strategy for application. In view of these aspects, the carbonyl cobalt CORMs with selectively inhibiting cyclooxygenase-2 has a potential property to be a medicine for therapy of oral squamous cell carcinoma.
Disclosure statement
The authors confirm that this article content has no conflict of interest.
Additional information
Funding
Reference
- Starmer HM, Abrams R, Webster K, et al. Feasibility of a mobile application to enhance swallowing therapy for patients undergoing radiation-based treatment for head and neck cancer. Dysphagia. 2018;33:227–233.
- Ferris RL, Blumenschein G, Jr., Fayette J, et al. Nivolumab for recurrent squamous-cell carcinoma of the head and neck. N Engl J Med. 2016;375:1856–1867.
- Price KA, Cohen EE. Current treatment options for metastatic head and neck cancer. Curr Treat Options Oncol. 2012;13:35–46.
- Marur S, Forastiere AA. Head and neck squamous cell carcinoma: update on epidemiology, diagnosis, and treatment. Mayo Clinic Procee. 2016;91:386–396.
- Petre A, Dalban C, Karabajakian A, et al. Carboplatin in combination with weekly Paclitaxel as first-line therapy in patients with recurrent/metastatic head and neck squamous cell carcinoma unfit to EXTREME schedule. Oncotarget. 2018;9:22038–22046.
- Dubot C, Bernard V, Sablin MP, et al. Comprehensive genomic profiling of head and neck squamous cell carcinoma reveals FGFR1 amplifications and tumour genomic alterations burden as prognostic biomarkers of survival. Eur. J. Cancer. 2018;91:47–55.
- Sacco AG, Cohen EE. Current treatment options for recurrent or metastatic head and neck squamous cell carcinoma. JCO. 2015;33:3305–3313.
- Law BYK, Qu YQ, Mok SWF, et al. New perspectives of cobalt tris(bipyridine) system: anti-cancer effect and its collateral sensitivity towards multidrug-resistant (MDR) cancers. Oncotarget. 2017;8:55003–55021.
- Kartal-Yandim M, Adan-Gokbulut A, Baran Y. Molecular mechanisms of drug resistance and its reversal in cancer. Crit. Rev. Biotechnol. 2016;36:716–726.
- Wu Q, Yang Z, Nie Y, et al. Multi-drug resistance in cancer chemotherapeutics: mechanisms and lab approaches. Cancer Lett. 2014;347:159–166.
- Paunescu E, McArthur S, Soudani M, et al. Nonsteroidal anti-inflammatory-organometallic anticancer compounds. Inorg. Chem. 2016;55:1788–1808.
- Ndagi U, Mhlongo N, Soliman M. E. Metal complexes in cancer therapy - an update from drug design perspective. Drug Des Devel Ther. 2017;11:599–616.
- Banti C. N, Giannoulis A. D, Kourkoumelis N, et al. Novel metallo-therapeutics of the NSAID naproxen. Interaction with intracellular components that leads the cells to apoptosis. Dalton Trans. 2014;43:6848–6863.
- Nagababu P, Barui AK, Thulasiram B, et al. Antiangiogenic activity of mononuclear copper(II) polypyridyl complexes for the treatment of cancers. J Med Chem. 2015;58:5226–5241.
- Dam J, Ismail Z, Kurebwa T, et al. Synthesis of copper and zinc 2-(pyridin-2-yl)imidazo[1,2-a]pyridine complexes and their potential anticancer activity. Eur J Medicinal Chem. 2017;126:353–368.
- Prathima B, Rao YS, Ramesh GN, et al. Synthesis, spectral characterization and biological activities of Mn(II) and Co(II) complexes with benzyloxybenzaldehyde-4-phenyl-3-thiosemicarbazone. Spectrochimica Acta A Mol Biomol Spectro. 2011;79:39–44.
- Tabrizi L, Talaie F, Chiniforoshan H. Copper(II), cobalt(II) and nickel(II) complexes of lapachol: synthesis, DNA interaction, and cytotoxicity. J Biomol Struct Dynamics. 2017;35:3330–3341.
- Kastl A, Wilbuer A, Merkel AL, et al. Dual anticancer activity in a single compound: visible-light-induced apoptosis by an antiangiogenic iridium complex. Chem. Communic (Cambridge, England). 2012;48:1863–1865.
- Munteanu CR, Suntharalingam K. Advances in cobalt complexes as anticancer agents. Dalton Trans. 2015;44:13796–13808.
- Tsukiji N, Osada M, Sasaki T, et al. Cobalt hematoporphyrin inhibits CLEC-2-podoplanin interaction, tumor metastasis, and arterial/venous thrombosis in mice. Blood Adv. 2018;2:2214–2225.
- King AP, Gellineau HA, Ahn JE, et al. Bis(thiosemicarbazone) complexes of cobalt(III). Inorg Chem. 2017;56:6609–6623.
- Thamilarasan V, Sengottuvelan N, Sudha A, et al. Cobalt(III) complexes as potential anticancer agents: physicochemical, structural, cytotoxic activity and DNA/protein interactions. J Photochemist Photobiol. 2016;162:558–569.
- Vignesh G, Pradeep I, Arunachalam S, et al. Biological and protein-binding studies of newly synthesized polymer-cobalt(III) complexes. Luminescence. 2016;31:533–543
- Schimler SD, Hall DJ, Debbert SL. Anticancer (hexacarbonyldicobalt)propargyl aryl ethers: synthesis, antiproliferative activity, apoptosis induction, and effect on cellular oxidative stress. J Inorganic Biochem. 2013;119:28–37.
- Schmidt K, Jung M, Keilitz R, et al. Acetylenehexacarbonyldicobalt complexes, a novel class of antitumor drugs. Inorganica Chimica Acta. 2000;306:6–16.
- Qin QP, Qin JL, Meng T, et al. High in vivo antitumor activity of cobalt oxoisoaporphine complexes by targeting G-quadruplex DNA, telomerase and disrupting mitochondrial functions. Eur J Med Chem. 2016;124:380–392.
- Sawle P, Foresti R, Mann BE, et al. Carbon monoxide-releasing molecules (CO-RMs) attenuate the inflammatory response elicited by lipopolysaccharide in RAW264.7 murine macrophages. Br J Pharmacol. 2005;145:800–810.
- Ahmad S, Hewett PW, Fujisawa T, et al. Carbon monoxide inhibits sprouting angiogenesis and vascular endothelial growth factor receptor-2 phosphorylation. Thromb Haemost. 2015;113:329–337.
- Wegiel B, Gallo D, Csizmadia E, et al. Carbon monoxide expedites metabolic exhaustion to inhibit tumor growth. Cancer Res. 2013;73:7009–7021.
- Simon T, Anegon I, Blancou P. Heme oxygenase and carbon monoxide as an immunotherapeutic approach in transplantation and cancer. Immunotherapy 2011;3:15–18.
- Gong YG, Zhang TF, Li M, et al. Toxicology and bioactivities of CO-releasing molecules based on cobalt complexes. Yao Xue Xue Bao = Acta Pharmaceutica Sinica 2016;51:425–433.
- Byatnal AA, Byatnal A, Sen S, et al. Cyclooxygenase-2–an imperative prognostic biomarker in oral squamous cell carcinoma–an immunohistochemical study. Pathol Oncol Res. 2015;21:1123–1131.
- Yin T, Wang G, Ye T, et al. Sulindac, a non-steroidal anti-inflammatory drug, mediates breast cancer inhibition as an immune modulator. Sci Rep. 2016;6:19534.
- Obermoser V, Baecker D, Schuster C, et al. Chlorinated cobalt alkyne complexes derived from acetylsalicylic acid as new specific antitumor agents. Dalton Trans. 2018;47:4341–4351.
- Yang B, Jia L, Guo Q, et al. Clinicopathological and prognostic significance of cyclooxygenase-2 expression in head and neck cancer: a meta-analysis. Oncotarget 2016;7:47265–47277.
- Sudbo J, Ristimaki A, Sondresen JE, et al. Cyclooxygenase-2 (COX-2) expression in high-risk premalignant oral lesions. Oral Oncol. 2003;39:497–505.
- St John MA. Inflammatory mediators drive metastasis and drug resistance in head and neck squamous cell carcinoma. Laryngoscope. 2015;125:S1–S11.
- Janakiraman H, House RP, Talwar S, et al. Repression of caspase-3 and RNA-binding protein HuR cleavage by cyclooxygenase-2 promotes drug resistance in oral squamous cell carcinoma. Oncogene 2017;36:3137–3148.
- Siebel AM, Vianna MR, Bonan CD. Pharmacological and toxicological effects of lithium in zebrafish. ACS Chem Neurosci. 2014;5:468–476.
- Li J, Zhang J, Zhang Q, et al. Synthesis and biological activities of carbonyl cobalt CORMs with selectively inhibiting cyclooxygenase-2. J Organomet Chem. 2018;874:49–62.
- Gong Y, Zhang T, Li M, et al. Toxicity, bio-distribution and metabolism of CO-releasing molecules based on cobalt. Free Radical Biol Med. 2016;97:362–374.
- Si J, Zhou R, Zhao B, et al. Effects of ionizing radiation and HLY78 on the zebrafish embryonic developmental toxicity. Toxicology 2019;411:143–153.
- McGrath P, Li CQ. Zebrafish: a predictive model for assessing drug-induced toxicity. Drug Discov Today. 2008;13:394–401.
- Zhao L, Si J, Wei Y, et al. Toxicity of porcelain-fused-to-metal substrate to zebrafish (Danio rerio) embryos and larvae. Life Sci. 2018;203:66–71.
- Liu Y, Yan J, Sun C, et al. Ameliorating mitochondrial dysfunction restores carbon ion-induced cognitive deficits via co-activation of NRF2 and PINK1 signaling pathway. Redox Biol. 2018;17:143–157.
- Zhou R, Zhang H, Wang Z, et al. The developmental toxicity and apoptosis in zebrafish eyes induced by carbon-ion irradiation. Life Sci. 2015;139:114–122.
- Sun C, Wang Z, Liu Y, et al. Carbon ion beams induce hepatoma cell death by NADPH oxidase-mediated mitochondrial damage. J Cellular Physiol. 2014;229:100–107.
- Sun C, Liu X, Di C, et al. MitoQ regulates autophagy by inducing a pseudo-mitochondrial membrane potential. Autophagy 2017;13:730–738.
- Wang P, Liu H, Zhao Q, et al. Syntheses and evaluation of drug-like properties of CO-releasing molecules containing ruthenium and group 6 metal. Eur J Med Chem. 2014;74:199–215.
- Li J, Zhang J, Zhang Q, et al. Syntheses and anti-cancer activity of CO-releasing molecules with targeting galactose receptors. Org Biomol Chem. 2018;16:8115–8129.
- Sipes NS, Padilla S, Knudsen TB. Zebrafish: as an integrative model for twenty-first century toxicity testing. Birth Defects Res C Embryo Today Rev. 2011;93:256–267.
- Cai G, Zhu J, Shen C, et al. The effects of cobalt on the development, oxidative stress, and apoptosis in zebrafish embryos. Biol Trace Elem Res. 2012;150:200–207.
- Reinardy HC, Syrett JR, Jeffree RA, et al. Cobalt-induced genotoxicity in male zebrafish (Danio rerio), with implications for reproduction and expression of DNA repair genes. Aquat Toxicol. 2013;126:224–230.
- Song JE, Si J, Zhou R, et al. Effects of exogenous carbon monoxide releasing molecules on the development of zebrafish embryos and larvae. Biomed Environ Sci. 2016;29:453–456.
- Zhou R, Song J, Si J, et al. Effects of Ru(CO)3Cl-glycinate on the developmental toxicities induced by X-ray and carbon-ion irradiation in zebrafish embryos. Mutat Res. 2016;793-794:41–50.
- Glickman NS, Yelon D. Cardiac development in zebrafish: coordination of form and function. Sem Cell Dev Biol. 2002;13:507–513.
- Elmore S. Apoptosis: a review of programmed cell death. Toxicol Pathol. 2007;35:495–516.
- Tucker B, Lardelli M. A rapid apoptosis assay measuring relative acridine orange fluorescence in zebrafish embryos. Zebrafish 2007;4:113–116.
- Mathieu C, Meier P, Meyer zu Starten A, et al. [Do selective COX-2 inhibitors have adverse renal and cardiovascular effects?]. Praxis (Bern 1994). 2005;94:1851–1858.
- Zeng C, Sun H, Xie P, et al. The role of apoptosis in MCLR-induced developmental toxicity in zebrafish embryos. Aquat Toxicol (Amsterdam, Netherlands). 2014;149:25–32.
- Kane AS, Salierno JD, Gipson GT, et al. A video-based movement analysis system to quantify behavioral stress responses of fish. Water Res. 2004;38:3993–4001.
- Si J, Zhou R, Song J, et al. Toxic effects of (56)Fe ion radiation on the zebrafish (Danio rerio) embryonic development. Aquat Toxicol (Amsterdam, Netherlands). 2017;186:87–95.
- Raftery TD, Volz DC. Abamectin induces rapid and reversible hypoactivity within early zebrafish embryos. Neurotoxicol Teratol. 2015;49:10–18.
- Wang ZG, Zhou R, Jiang D, et al. Toxicity of graphene quantum dots in zebrafish embryo. Biomed Environ Sci. 2015;28:341–351.
- Steenbergen PJ, Richardson MK, Champagne DL. The use of the zebrafish model in stress research. Prog Neuro-Psychopharmacol Biol Psychiatry. 2011;35:1432–1451.
- Zhang T, Xu L, Wu JJ, et al. Transcriptional responses and mechanisms of copper-induced dysfunctional locomotor behavior in zebrafish embryos. Toxicol Sci. 2015;148:299–310.
- Verma R, Singh A, Jaiswal R, et al. Association of Ki-67 antigen and p53 protein at invasive tumor front of oral squamous cell carcinoma. Indian J Pathol Microbiol. 2014;57:553–557.
- Cerignoli F, Abassi YA, Lamarche BJ, et al. In vitro immunotherapy potency assays using real-time cell analysis. PloS one. 2018;13:e0193498.
- Gong H, Zolzer F, von Recklinghausen G, et al. Arginine deiminase inhibits proliferation of human leukemia cells more potently than asparaginase by inducing cell cycle arrest and apoptosis. Leukemia. 2000;14:826–829.
- Yu T, Wu Y, Helman JI, et al. CXCR4 promotes oral squamous cell carcinoma migration and invasion through inducing expression of MMP-9 and MMP-13 via the ERK signaling pathway. Mol Cancer Res MCR. 2011;9:161–172.
- Gallo O, Masini E, Bianchi B, et al. Prognostic significance of cyclooxygenase-2 pathway and angiogenesis in head and neck squamous cell carcinoma. Human Pathol. 2002;33:708–714.
- Zou C, Zhang H, Li Q, et al. Heme oxygenase-1: a molecular brake on hepatocellular carcinoma cell migration. Carcinogenesis 2011;32:1840–1848.
- Stein U, Arlt F, Smith J, et al. Intervening in beta-catenin signaling by sulindac inhibits S100A4-dependent colon cancer metastasis. Neoplasia (New York, N.Y.). 2011;13:131–144.
- Pyatrikas DV, Fedoseeva IV, Varakina NN, et al. Relation between cell death progression, reactive oxygen species production and mitochondrial membrane potential in fermenting Saccharomyces cerevisiae cells under heat-shock conditions. FEMS Microbiol Lett. 2015;362:fnv082.
- Wang JP, Hsieh CH, Liu CY, et al. Reactive oxygen species-driven mitochondrial injury induces apoptosis by teroxirone in human non-small cell lung cancer cells. Oncol Lett. 2017;14:3503–3509.
- Bazhin AV, Philippov PP, Karakhanova S. Reactive oxygen species in cancer biology and anticancer therapy. Oxid Med Cell Longevity. 2016;2016:1.
- Mathur A, Hong Y, Kemp BK, et al. Evaluation of fluorescent dyes for the detection of mitochondrial membrane potential changes in cultured cardiomyocytes. Cardiovasc Res. 2000;46:126–138.