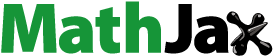
Abstract
The mucosal immune system serves as the first line of defense against Bordetella pertussis. Intranasal vaccination, due to its potential to induce systemic and mucosal immune responses, appears to prevent the initial adherence and colonization of the bacteria at the first point of contact. In the present study, two B. pertussis antigens, pertussis Toxoid (PTd) and Filamentous hemagglutinin (FHA), which play a very significant role in virulence and protection against pertussis, were encapsulate into N-trimethyl chitosan (TMC) nanoparticulate systems. After preparation of TMC nanoparticles (NPs), the NPs were characterized and their ability to induce efficient immune responses against B. pertussis was studied in a mouse model. Our findings showed that PTd + FHA-loaded TMC NPs have strong ability to induce IL-4, IL-17, IFN-γ, IgG, and IgA in the mouse model. Results from this study suggest that nasal administration of the PTd + FHA-loaded TMC NPs induced not only a systemic immune response but also a local mucosal response, which may improve the efficacy of pertussis prevention through respiratory tract transmission.
Introduction
Pertussis or whooping cough, mainly caused by the Gram-negative bacteria Bordetella pertussis (B. pertussis), is a highly contagious acute respiratory infection of the upper respiratory tract [Citation1]. Pertussis is initiated by adherence of bacteria to ciliated respiratory epithelium in the nasopharynx and trachea [Citation2]. The mucosal tissues of the upper respiratory tract are the main portal entry of B. pertussis [Citation3]. Of note, the mucosal immune system provides the first line of defense against the pathogen. Therefore, the local mucosal immune responses in the respiratory tract may be important in order to overcome this disease [Citation4].
There are currently two vaccine generations, including whole-cell vaccines and acellular vaccines for parenteral administration, for the prevention of the disease [Citation5]. The first generation, whole-cell vaccines, is composed of killed and detoxified bacteria while the second generation, acellular vaccines, contains purified or recombinant B. pertussis antigens [Citation6]. Despite a significant decline in the incidence of the disease, whole-cell vaccines, due to side effects such as fever, seizure and encephalopathy, have been replaced with acellular vaccines in a wide variety of countries [Citation7]. The acellular pertussis vaccines are produced by purification and inactivation of appropriate antigens of B. pertussis, among which virulence factors including pertussis toxin (PT), Filamentous hemagglutinin (FHA), Fimbriae (Fim2 and Fim3), and pertactin (PRN) [Citation8] have fewer side effects and prevent acute forms of pertussis [Citation9]; however, they were shown to be ineffective in preventing initial adherence, nasal colonization, bacterial transmission, complete clearance, and long-term protection [Citation10,Citation11]. The inefficiency of the current acellular pertussis vaccines can be one of the most important reasons for the increased incidence of pertussis in many countries with high vaccine coverage [Citation12,Citation13]. Of note, intranasal vaccination, due to the potential for inducing systemic and mucosal immune responses, seems to be able to prevent the organism from colonization at the first point of contact [Citation4].
PT and FHA are the main virulence factors of B. pertussis, which not only mediate the binding of bacteria to ciliated cells and phagocytes but also play important roles in the establishment and pathogenesis of the bacteria in the respiratory tract [Citation14]. Antibodies against PT and FHA were shown to prevent bacterial colonization, resulting in significant protection against infection [Citation15]. The access of antigens administered through the intranasal route to sub-epithelial lymphoid tissues is an essential factor for the development of mucosal and systemic immune responses [Citation16]. However, the access to soluble antigens is impeded through several factors such as epithelial barrier, enzymatic degradation, and mucociliary clearance [Citation17]. More importantly, due to the poor ability of subunit vaccines to induce a potent immune response, there is a need for the use of an effective adjuvant in the design of such vaccines [Citation18]. For this purpose, the encapsulation of antigens in a nanoparticle (NP) system can be a suitable option for nasal administration of vaccines [Citation19,Citation20]. Such a system can serve not only as an antigen delivery system but also as an appropriate adjuvant.
The purpose of the present study was to encapsulate pertussis toxoid (PTd) and FHA from B. pertussis into N-trimethyl chitosan (TMC) through an ionotropic gelation method for the development of pertussis intranasal vaccination. After synthesis, the potential protection of PTd + FHA-loaded TMC NPs, as a carrier and adjuvant system, was immunologically investigated in an in vitro model.
Materials and methods
Preparation of PTd + FHA-loaded TMC NPs
NPs were synthesized based on ionic gelation of TMC, as described previously [Citation21]. In brief, various concentrations (0.5, 1, 1.5, and 2 mg/mL) of TMC were prepared in distilled water and then the solution was filtered through a 0.45 mm membrane filter. PTd and FHA (each 10 mg/mL) were added to sodium tripolyphosphate (TPP) solution (1 mg/mL), and 200 µl of PTd + FHA + TPP solution (1 mg/mL) was added dropwise to TMC solution when gently mixed for 45 min with a magnetic stirrer at room temperature. The resultant NPs were collected by centrifuging at 10,000 g for 20 min on a glycerol bed (10 µl). Subsequently, the supernatants were discarded and the pellet was collected and suspended in phosphate buffer saline (PBS) to prepare antigen-loaded NPs for physicochemical analysis and immunization.
Characterization of PTd + FHA-loaded TMC NPs
The size and zeta potential of the synthesized NPs were characterized by dynamic light scattering (DLS) using the zeta sizer SZ3000 (Malvern instrument, Worcestershire, UK) [Citation22]. Transmission electron microscopy (TEM) was used to examine the morphology of the NPs. To determine the amount of the entrapped antigen in the NPs, we calculated the differences between the total amount added to the loading solution and the amount of non-entrapped antigen remaining in the supernatant. The concentration of PTd and FHA in the supernatants was calculated by using the BCA protein assay (Sigma-Aldrich, USA) [Citation23]. The encapsulation efficacy (EE) of PTd + FHA-loaded TMC NPs was measured using the following equation:
In vitro release
PTd + FHA-loaded TMC NPs were isolated by centrifugation at 10,000 g at 4 °C on 10 µl of a glycerol bed for 20 min. The supernatant was removed, the NPs were re-suspended in 6 ml of 0.1 M PBS (pH 7.4), and resultant solution was kept at 37 °C under magnetic stirring (100 rpm). To assess the amount of the released antigen from NPs, 0.5 ml of the suspension was collected at different time intervals and centrifuged at 18,000 g for 15 min; afterwards, the BCA protein assay was applied to determine the amount of antigen in the supernatant. Lastly, the same volume of freshly-prepared PBS buffer was added to the release medium to reach the original volume. A sample with non-loaded TMC NPs was applied as a blank [Citation24].
Immunization studies
Four- to 6-week-old female BALB/c mice were purchased from the Animal Rearing Department of Pasteur Institute of Iran, Tehran, Iran. The mice were fed by standard grower diet ad labium according to the National Research Council Requirements (National Research Council. Subcommittee on laboratory animal, 1995). As shown in , the mice were randomly divided into five groups and immunized intranasally (i.n.) or intraperitoneally (i.p.) two times at 4-week intervals. Before vaccination, the mice were lightly anesthetized using ketamine (100 mg/kg) and Xylazine (10 mg/kg) through i.p. injection. Each mouse was immunized and boosted i.n. with 20 µl of a solution consisting of 6 µg of PTd and 6 µg of FHA; the mice were administered by an equal amount of 10 µl in each nostril with micropipette tip and allow for the mice to inhale the vaccine. Blood collection and nasal washing were carried out on day 42. Blood samples were collected by the retro-orbital-puncture method, and the sera were isolated by centrifugation at 5000 rpm for 10 min. Nasal washing was performed by injection of 200 μl of cold solution retrograde through the trachea into the nasopharynx, and the lavage fluid was collected at the nostrils. The samples were stored at −20 °C until ELISA (Enzyme-linked Immunosorbent Assay) analysis.
Table 1. Immunization study design.
Antibody determinations
ELISA, as described previously with some modifications, was used to determine the anti-FHA or anti-PTd serum IgG and secretory IgA (sIgA) antibodies [Citation25]. Each antigen was diluted to 2 μg/mL, added separately to microtitration plates (Nunc, Denmark), and incubated at 4 °C for overnight. The plates were washed with PBS containing 0.05% Tween-20 (PBS-T) and blocked with 5% non-fat dry milk in PBS for 1 h in 37 °C. Following three washes, the samples (1:100 diluted in PBS) were loaded on antigen-coated plates, and the plates were incubated for 60 min at 37 °C and then washed with PBS-T. Afterwards, 0.1 mL of HRP-conjugated goat anti-mouse IgG or IgA (Abcam, 1:6000 diluted) was added to each well, and the plates were incubated for 60 min at 37 °C. Following further washing steps, 100 mL of 3,3′,5,5′-tetramethylbenzidine (TMB) substrate was added to each well. After 20-min incubation at room temperature, the stop solution (0.1 N sulfuric acid) was added, and optical density was calculated at 450 nm using an automatic microplate reader (Bio-Rad, USA).
Cytokine assay
All the experimental mice were sacrificed by cervical dislocation 2 weeks after the last immunization, and the spleens were dissected aseptically. The resultant splenocytes were prepared as a single cell suspension, cultured (2 × cells/well) in RPMI-1640 medium (Sigma, Germany) supplemented with 10% fetal calf serum (FCS; Sigma, Germany) in the presence of 1 µg PT and 1 µg FHA, and incubated for 72 h at 37 °C in an atmosphere of 5% CO2. Positive and negative controls were stimulated with phytohemagglutinin-A (PHA) (2 µg/mL) and left unstimulated, respectively. Cell culture supernatants were harvested for cytokine assay by using mouse IL-17, IL-4, and IFN-γ ELISA kits (R&D Systems Inc., Minneapolis, MN, USA) according to manufacturers’ protocols. indicates all the experimental procedure used in this study.
Statistical analysis
The results are expressed as the mean ± standard error of the mean (SEM). Differences between groups were performed using unpaired two-tailed Student’s t-test. p-Values less than 0.05 (p < .05) were considered to be statistically significant.
Results
Characterization of PTd + FHA-loaded TMC NPs
The size and zeta potential of PTd + FHA-loaded TMC NPs were analyzed by the DLS technique. Morphological features of NPs were measured using TEM (). The mean size of the particle was 252.8 nm with a zeta potential of +30.8 mV which were acquired in a TMC concentration of 1 mg/mL (). Their polydispersity indexes (PI) were found to be in the range of 0.1–0.2, demonstrating that the samples prepared with the 1 mg/mL TMC concentration had the narrow size distribution than those prepared with higher TMC concentrations. The encapsulation efficacy of NPs was measured by bicinchoninic acid protein assay, found to be above 94% for PTd + FHA-loaded TMC NPs (). After 96 h, the in vitro antigen release from NPs was detected to be 93.12% ().
Figure 2. Transmission electron microscopy micrograph of PTd + FHA-loaded TMC NPs. PTd: pertussis toxoid; FHA: Filamentous hemagglutinin; TMC: N-trimethyl chitosan; NP: nanoparticle.
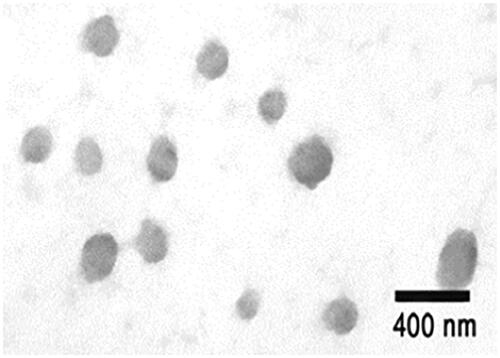
Figure 3. In vitro release profile of antigen from TMC NPs. TMC: N-trimethyl chitosan; NP: nanoparticle.
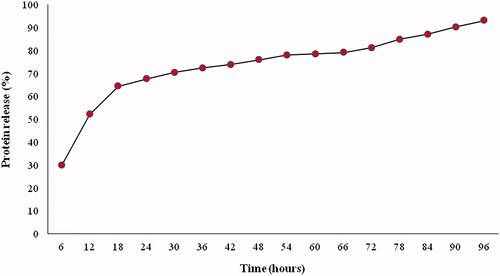
Table 2. Characterization of antigen-loaded TMC NPs.
Antigen-specific antibody levels following immunization
Results from the Humoral immunity assessment showed that IgG levels produced against PTd and FHA in the mice receiving PTd + FHA-loaded TMC NPs in the nasal administration, and those receiving the injectable antigen in combination with the alum adjuvant were the highest rate, demonstrating to have a significant difference as compared with other groups (p > .05) (). The highest levels of sIgA concentration were found in mice receiving antigens in the TMC NPs and i.n. when compared with other mouse groups. No detectable sIgA response was obtained after i.p. immunization using antigen-alum formulation ().
Cytokine measurements
Different groups of mice were investigated separately in this study. Based on the method described above, the spleen was isolated, homogenized and the spleen cells were cultured in the vicinity of the antigen for 72 h and the supernatant of spleen cells from the mice were assessed for cytokines production. Based on the results depicted in , the highest levels of IFN-γ and IL-17 production of antigen-activated spleen cells were found in the group of mice immunized with PTd + FHA-loaded TMC NPs. Furthermore, the production of IFN-γ in mouse groups receiving PTd and FHA antigens, both soluble and nasal or injectable with the alum adjuvant, was not significantly higher than the negative control group (those receiving the PBS and TMC alone) (p > .05). The highest levels of IL-4 production were found in the group receiving PTd and FHA antigens plus alum adjuvant. In addition, mice immunized with PTd + FHA-loaded TMC NPs exhibited a high level of IL-4 production.
Discussion
The resurgence of pertussis has occurred prominently in countries where the children were vaccinated with acellular pertussis vaccines [Citation10]. Commercially-licensed acellular pertussis vaccines, although inducing strong antibody and Th2 responses, fail to drive local mucosal and cell-mediated immune responses [Citation26–28]. The latter is required for optimum protection against nasal colonization and transmission of B. pertussis [Citation4]. Due to unique advantages of nasal routes and biocompatible/biodegradable polymers, in this study we developed an appropriate pertussis vaccine candidate using PTd + FHA-loaded TMC NPs for the nasal administration in a mouse model.
For this purpose, the ionotropic gelation method was applied to develop PTd + FHA-loaded TMC NPs. The method mediates the interaction between non-toxic negatively-charged crosslinker reagent (TPP) and positively-charged amino groups of TMC. Chitosan, a natural-based polymer obtained by alkaline deacetylation of chitin, is biodegradable, non-toxic, and biocompatible whose administration to humans does not pose any danger [Citation29]. Chitosan and its derivatives have the properties making them suitable, as a carrier and adjuvant, for mucosal vaccines [Citation30]. However, despite its successful application, chitosan is insoluble in physiologic PH [Citation31]. In contrast, TMC, a derivative of chitosan, has a suitable solubility in a wide range of PH. Hence, TMC has bio-adhesive properties and absorption enhancing effects in normal pH [Citation32].
Results from the physicochemical characterization of PTd + FHA-loaded TMC NPs demonstrated that the apparent characteristics and the size of the resultant NPs were suitable in 1 mg/mL of TMC. The loading efficacy of antigens into the nanoparticulate systems (approximately 95%) showed that the application of the ionic gelation method was able to incorporate a good amount of PTd and FHA onto the TMC NPs. The mean size of PTd + FHA-loaded TMC NPs was found to be nearly 252 nm, representing to be suitable for intranasal administration. Previously-published studies revealed that particles smaller than 1 µm have the ability to easily uptaken by M cells, epithelial cells, as well as dendritic cells [Citation33]. What is more, it was demonstrated that there seems no difference in immunogenicity between particles with the size of ∼200 to 500 nm [Citation34].
Our results from the positive zeta potential showed that the synthesized NPs obtained in this study can be effectively attached to the nasal mucosa. The electrostatic interaction between positively-charged TMC NPs and anionic glycoproteins present in the mucus layer appears to increase the residence time of the antigen. This results in increased antigen uptake by M cells from the nasal cavity to the sub-epithelial immune cells [Citation35–37].
Our findings from in vitro antigen release indicated that nearly 70% of the antigens were associated with the TMC NPs for 5 h under physiological conditions. It is valuable to note that the antigen is not released to a large extent before the antigen-loaded NPs pass the nasal mucosal barrier during their residence in the nasal cavity [Citation38].
Intranasal vaccination using PTd + FHA-loaded TMC NPs led to potent local and systemic immune responses when compared with free PTd and FHA administration. This showed that TMC NPs not only have an intrinsic immunostimulating effect but also can serve as a suitable vaccine adjuvant delivery system for nasally administered vaccines, which is in agreement with previous results [Citation39–41].
Prolonged exposure of the antigen to nasal mucosa, improved uptake by M-cells and antigen-presenting cells (APCs) at the nasal mucosa, more efficient delivery to mucosal lymph nodes, and/or more efficient stimulation of APCs after uptake are factors more likely contributing to the immunostimulating effect of TMC NPs on i.n. administration. Furthermore, TMC appears to open the intercellular tight junctions, facilitating the paracellular transport of antigens and increasing antigen absorption [Citation42].
The induction of sIgA antibodies at the mucosal epithelium is the leading advantage of i.n. vaccination [Citation43]. sIgA, as the first defensive line, plays a key role against B. pertussis at the portal of pathogen entry in the respiratory tract [Citation26,Citation44]. Our findings clearly demonstrated that the PTd + FHA-loaded TMC NPs administered i.n. were the only formulation capable of inducing high sIgA titers after two immunizations. In contrast, antigen formulations administered i.p. failed to show sIgA responses. This finding is consistent with previouslypublished documents that parenteral administrations of antigen formulations lack the ability to induce sIgA responses [Citation45,Citation46].
Conclusion
The present study demonstrated that TMC NPs are a suitable adjuvant/delivery system for effective induction of mucosal and systemic immune responses against pertussis in mice. The nasal administration of the PTd + FHA-loaded TMC NPs had the ability to induce both a systemic immune response and a local mucosal response, presumably leading to improved efficacy of pertussis prevention through respiratory tract transmission.
Disclosure statement
The authors stated that there are no conflicts of interest. The authors alone are responsible for the content and writing of this article.
Correction Statement
This article has been republished with minor changes. These changes do not impact the academic content of the article.
Additional information
Funding
References
- Kilgore PE, Salim AM, Zervos MJ, et al. Pertussis: microbiology, disease, treatment, and prevention. Clin Microbiol Rev. 2016;29:449–486.
- Melvin JA, Scheller EV, Miller JF, et al. Bordetella pertussis pathogenesis: current and future challenges. Nat Rev Microbiol. 2014;12:274–288.
- Siegel SJ, Weiser JN. Mechanisms of bacterial colonization of the respiratory tract. Annu Rev Microbiol. 2015;69:425–444.
- Solans L, Locht C. The role of mucosal immunity in pertussis. Front Immunol. 2018;9:3068.
- Berbers GA, de Greeff SC, Mooi F. Improving pertussis vaccination. Hum Vaccin. 2009;5:497–503.
- Storsaeter J, Wolter J, Locht C. Pertussis vaccines. In Locht C, editor. Bordetella molecular microbiology. Norfolk, UK: Horizon Press; 2007. p. 245–288.
- Kuchar E, Karlikowska-Skwarnik M, Han S, et al. Pertussis: history of the disease and current prevention failure. In: Pokorski M, editor. Pulmonary dysfunction and disease. Cham, Switzerland: Springer International Publishing; 2016. p. 77–82.
- Poolman JT, Hallander H. Acellular pertussis vaccines and the role of pertactin and fimbriae. Expert Rev Vacc. 2007;6:47–56.
- Locht C, Mielcarek N, Microbiology M. New pertussis vaccination approaches: en route to protect newborns? FEMS Immunol Med Microbiol. 2012;66:121–133.
- Klein NP, Bartlett J, Rowhani-Rahbar A, et al. Waning protection after fifth dose of acellular pertussis vaccine in children. N Engl J Med. 2012;367:1012–1019.
- Warfel JM, Zimmerman LI, Merkel T. Acellular pertussis vaccines protect against disease but fail to prevent infection and transmission in a nonhuman primate model. PNAS. 2014;111:787–792.
- Burdin N, Handy LK, Plotkin S. What is wrong with pertussis vaccine immunity? Cold Spring Harb Perspect Biol. 2017;9:a029454.
- Locht C. Pertussis: where did we go wrong and what can we do about it? J Infect. 2016;72:S34–S40.
- van den Berg BM, Beekhuizen H, Willems RJ, et al. Role of Bordetella pertussis virulence factors in adherence to epithelial cell lines derived from the human respiratory tract. Infect. Immun 1999;67:1056–1062.
- van den Berg BM, Beekhuizen H, Mooi FR, et al. Role of antibodies against Bordetella pertussis virulence factors in adherence of Bordetella pertussis and Bordetella parapertussis to human bronchial epithelial cells. Infect Immun. 1999;67:1050–1055.
- Ugwoke MI, Agu RU, Verbeke N, et al. Nasal mucoadhesive drug delivery: background, applications, trends and future perspectives. Adv Drug Deliv Rev. 2005;57:1640–1665.
- Illum L. Nasal drug delivery—possibilities, problems and solutions. J Contr Rel. 2003;87:187–198.
- Perrie Y, Mohammed AR, Kirby DJ, et al. Vaccine adjuvant systems: enhancing the efficacy of sub-unit protein antigens. Int J Pharm. 2008;364:272–280.
- Akagi T, Baba M, Akashi M. Biodegradable nanoparticles as vaccine adjuvants and delivery systems: regulation of immune responses by nanoparticle-based vaccine. In Polymers in nanomedicine. Berlin, Heidelberg: Springer; 2011. p. 31–64.
- Marasini N, Skwarczynski M, Toth I. Intranasal delivery of nanoparticle-based vaccines. Ther Deliv. 2017;8:151–167.
- Calvo P, Remunan‐Lopez C, Vila‐Jato JL, et al. Novel hydrophilic chitosan‐polyethylene oxide nanoparticles as protein carriers. J Appl Polym Sci. 1997;63:125–132.
- Slütter B, Bal S, Keijzer C, et al. Nasal vaccination with N-trimethyl chitosan and PLGA based nanoparticles: nanoparticle characteristics determine quality and strength of the antibody response in mice against the encapsulated antigen. Vaccine. 2010;28:6282–6291.
- Smith PK, Krohn RI, Hermanson G, et al. Measurement of protein using bicinchoninic acid. Anal Biochem. 1985;150:76–85.
- Chen F, Zhang Z-R, Huang Y. Evaluation and modification of N-trimethyl chitosan chloride nanoparticles as protein carriers. Int J Pharm. 2007;336:166–173.
- Shi W, Kou Y, Jiang H, et al. Novel intranasal pertussis vaccine based on bacterium-like particles as a mucosal adjuvant. Immunol Lett. 2018;198:26–32.
- Higgs R, Higgins S, Ross P, et al. Immunity to the respiratory pathogen Bordetella pertussis. Mucos Immunol. 2012;5:485.
- Ross PJ, Sutton CE, Higgins S, et al. Relative contribution of Th1 and Th17 cells in adaptive immunity to Bordetella pertussis: towards the rational design of an improved acellular pertussis vaccine. PLoS One. 2013;9:e1003264.
- Allen AC, Wilk MM, Misiak A, et al. Sustained protective immunity against Bordetella pertussis nasal colonization by intranasal immunization with a vaccine-adjuvant combination that induces IL-17-secreting T RM cells. Mucos Immunol. 2018;11:1763.
- Mehrabi M, Montazeri H, Mohamadpour Dounighi N, et al. Chitosan-based nanoparticles in mucosal vaccine delivery. Arch Razi Inst. 2018;73:165–176.
- Jabbal-Gill I, Watts P, Smith A. Chitosan-based delivery systems for mucosal vaccines. Expert Opin Drug Deliv. 2012;9:1051–1067.
- Choi C, Nam JP, Nah JW, et al. Application of chitosan and chitosan derivatives as biomaterials. J Ind Eng Chem. 2016;33:1–10.
- Verheul RJ, Amidi M, van der Wal S, et al. Synthesis, characterization and in vitro biological properties of O-methyl free N, N, N-trimethylated chitosan. Biomaterials. 2008;29:3642–3649.
- Vila A, Sanchez A, Evora C, et al. PLA-PEG particles as nasal protein carriers: the influence of the particle size. Int J Pharm. 2005;292:43–52.
- Gutierro I, Hernández RM, Igartua M, et al. Size dependent immune response after subcutaneous, oral and intranasal administration of BSA loaded nanospheres. Vaccine. 2002;21:67–77.
- Neutra MR, Kozlowski P. Mucosal vaccines: the promise and the challenge. Nat Rev Immunol. 2006;6:148.
- Pardeshi CV, Belgamwar VS. Controlled synthesis of N,N,N-trimethyl chitosan for modulated bioadhesion and nasal membrane permeability. Int J Biol Macromol. 2016;82:933–944.
- Slütter B, Plapied L, Fievez V, et al. Mechanistic study of the adjuvant effect of biodegradable nanoparticles in mucosal vaccination. J Contr Rel. 2009;138:113–121.
- Amidi M, Romeijn SG, Verhoef JC, et al. N-trimethyl chitosan (TMC) nanoparticles loaded with influenza subunit antigen for intranasal vaccination: biological properties and immunogenicity in a mouse model. Vaccine. 2007;25:144–153.
- Dabaghian M, Latifi AM, Tebianian M, et al. Nasal vaccination with r4M2e. HSP70c antigen encapsulated into N-trimethyl chitosan (TMC) nanoparticulate systems: Preparation and immunogenicity in a mouse model. Vaccine. 2018;36:2886–2895.
- Amini Y, Tebianian M, Mosavari N, et al. Development of an effective delivery system for intranasal immunization against Mycobacterium tuberculosis ESAT-6 antigen. Artific Cells, Nanomed, Biotech. 2017;45:291–296.
- Subbiah R, Ramalingam P, Ramasundaram S, et al. N,N,N-Trimethyl chitosan nanoparticles for controlled intranasal delivery of HBV surface antigen . Carbohydr Polym. 2012;89:1289–1297.
- Vllasaliu D, Exposito-Harris R, Heras A, et al. Tight junction modulation by chitosan nanoparticles: comparison with chitosan solution. Int J Pharmac. 2010;400:183–193.
- Ramvikas M, Arumugam M, Chakrabarti SR, et al. Nasal Vaccine Delivery. In Skwarczynski M, Toth I, editors. Micro and nanotechnology in vaccine development. Norwich, NY: William Andrew Publishing; 2017. p. 279–301.
- Hellwig SM, van Spriel AB, Schellekens JF, et al. Immunoglobulin A-mediated protection against Bordetella pertussis infection. Infect Immun. 2001;69:4846–4850.
- Jabbal-Gill I, Fisher AN, Rappuoli R, et al. Stimulation of mucosal and systemic antibody responses against Bordetella pertussis filamentous haemagglutinin and recombinant pertussis toxin after nasal administration with chitosan in mice. Vaccine. 1998;16:2039–2046.
- Cropley I, Douce G, Roberts M, et al. Mucosal and systemic immunogenicity of a recombinant, non-ADP-ribosylating pertussis toxin: effects of formaldehyde treatment. Vaccine. 1995;13:1643–1648.