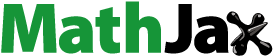
Abstract
Poor cellular uptake and slow intracellular drug release remain the main barriers for the efficient application of micellar delivery system. Taking advantage of the overexpressed CD44 receptor and mild acidic microenvironment of tumour cells, CD44-targeted pH-responsive micelles based on the self-assembly of histidine–hyaluronic acid–dodecylamine (His–HA–DA) were prepared for the delivery of doxorubicin (DOX). These micelles exhibited pH-responsive behaviour with increased particle size, decreased encapsulation efficiency (EE%) of DOX and rapid release of DOX triggered by low pH. Compared with free DOX, DOX/HHD exhibited relatively high cellular uptake mainly via the CD44-mediated endocytosis. The on-demand intracellular release of DOX from DOX/HHD led to improved cytotoxicity. DOX/HHD also showed great penetration efficiency in 3D tumour spheres in vitro. Moreover, these micelles with suitable particle size gained excellent tumour-targeting effects, as well as improved anti-tumour effects and reduced side effects in vivo. In conclusion, these micelles with CD44 targeted and pH-responsive behaviours provide a promising strategy for the efficient delivery of anti-tumour drugs in vivo.
Graphical Abstract
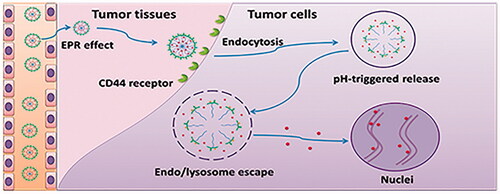
Introduction
Micellar delivery system, one of the most promising nano-delivery systems, has been deeply developed for the delivery of anti-tumour agents during the past decades. Typical polymer micelles are based on the self-assembly of the amphiphilic polymers with hydrophilic segments to ensure stability during the blood circulation and hydrophobic segments to load the insoluble drugs [Citation1,Citation2]. Compared with traditional anti-tumour drugs, drug-loaded polymer micelles exhibit various advantages, such as improving the solubility of hydrophobic agents, enhancing the serum stability before reaching the targeted areas, and significantly enhancing the accumulation in the tumour tissues via the enhanced permeability and retention (EPR) effect [Citation3], leading to the improvement of the therapeutic effects and reduction of the side effects [Citation4]. Despite the extensive research on micellar delivery systems, low intracellular drug concentration and poor therapeutic effect caused by the low cellular uptake and uncontrolled intracellular drug release impeded the application of these delivery systems [Citation5,Citation6].
As for the delivery of anti-tumour drugs in vivo, polymer micelles accumulate in tumour tissues and then are internalized. The size-dependent EPR effect only leads to the accumulation in tumour tissues while the subsequent internalization is usually hampered owing to the weak interactions between the hydrophilic micellar shell and the amphiphilic cellular membrane. As reported previously, modification with targeted ligands in polymer micelles that could specifically bind to the receptors on tumour cells could significantly enhance the cellular uptake via the ligand–receptor-mediated endocytosis [Citation7,Citation8]. Over the past years, various targeted ligands, like RGD peptide, folic acid [Citation9,Citation10] and transferrin [Citation11] have been used for the modification of polymer micelles. It is reported that CD44 receptors are overexpressed in many tumour cells such as 4T1 cell lines. Hyaluronic acid (HA), possessing high-specific interaction with CD44 receptor, has been widely used as a target ligand to achieve active targeting therapeutic effect. Besides, the excellent biocompatibility and the inherent structure promoted HA to be utilized as a hydrophilic segment of vector to improve the serum stability of the whole delivery system [Citation12]. For example, Wang et al. [Citation13] designed the redox-responsive micellar delivery system based on the self-assembly of HA–Lys–LA conjugates for the effective delivery of chemotherapeutic agent doxorubicin (DOX), which exhibited enhanced cellular uptake and improved cytotoxicity in vitro and revealed good bioavailability and excellent targeted efficiency in vivo.
Another obstacle for the application of polymer micelles is the slow release of payloads from micelles after the internalization, which leads to the limitation of therapeutic effects and initiation of multidrug resistance (MDR) [Citation14]. As for an idol micellar delivery system, the payloads are expected to release slowly during the blood circulation, but rapidly within tumour cells. To address this contradiction, smart polymer micelles that can respond to the tumour microenvironment have attracted much attention [Citation15,Citation16]. These smart delivery systems exhibit sustained release of payloads before arriving at targeted sites while rapid release of payloads is triggered by special signals within tumour cells, such as low pH [Citation7,Citation17], high concentration of glutathione (GSH) [Citation18], and reactive oxygen species (ROS) [Citation19]. Among these intracellular stimuli, pH in endo/lysosome which is significantly lower than that in normal tissues in blood circulation is the most frequently used one to design an intracellular-controlled drug delivery system. The pH responsiveness of the polymer micelles can be triggered by the breakage of the chemical bond in the polymer or via the protonated/deprotonated strategy under the acidic conditions, which led to the disassembly of the micelles and resulted in sharp and complete release of the cargo [Citation20]. Histidine has been widely used as the pH-responsive segment in smart micelles, wherein the imidazole ring with pKa of around 6.5 in the structure would be pronated at endo/lysosomal pH resulting in the loss of hydrophilic/hydrophobic balance and final disintegration of smart micelles [Citation21]. Furthermore, the protonation of imidazole group may lead to the endosome escape of drug-loaded micelles via the proton sponge effect, which is in favour of reducing the drug degradation by the enzymes in lysosomes [Citation22,Citation23].
In this study, a novel CD44-targeted micellar delivery system based on the tumour environment-responsive polymer histidine–hyaluronic acid–dodecyl amine (His–HA–DA) was developed for the effective delivery of widely used chemotherapeutic agent DOX. Briefly, HA, as the hydrophilic backbone and the targeted ligand, was conjugated with the hydrophobic segment DA wherein DOX was loaded and finally modified with the pH-responsive segment His to obtain the vector His–HA–DA. It is assumed that the HA modification would help DOX-loaded micelles DOX/His–HA–DA stay stable and exhibit good biocompatibility in blood circulation, significantly accumulating in tumour sites and then be abundantly internalized by tumour cells via the ligand–receptor-mediated endocytosis [Citation24]. After internalization, His modification enables sharp and complete intracellular DOX release to achieve enhanced tumour therapy efficacy (shown in Scheme 1).
Materials and methods
Materials, cell lines and animals
HA with molecular weight (MW) of 8–10 kDa was obtained from Fruida Biochemistry Co., Ltd (Shandong, China). 3-(3-Dimethylamin-opropyl)-1-ethylcarbodiimide hydrochloride (EDC•HCl), N-Hydroxysuccinimide (NHS) and Dodecylamine (DA) were purchased from Adamas-beta-Reagent Co., Ltd. Histidine (His) was obtained from Sinopharm Chemical Reagent Co., Ltd (Shanghai, China). Doxorubicin hydrochloride (DOX•HCl) over 98% purity was from Melone Pharmaceutical Co., Ltd (Dalian, China). Methyl thiazolyl tetrazolium bromide (MTT) was achieved from Sigma-Aldrich (St. Louis, MO). Hoechst 33258 and LysoTraker Green were from Invitrogen (Eugene, OR, USA). RPMI-1640 culture medium was bought from Hyclone Thermo-Fisher Biochemical Products Co., Ltd (Beijing, China). Foetal bovine serum (FBS) was purchased from Zhejiang Tianhang Biotechnology Co., Ltd (Hangzhou, China). All other chemicals were of analytical grade and utilized without further purification.
Murine breast cancer cell lines 4T1 from Jiangsu Province Key Laboratory of Biotechnology and Immunology (Suzhou, China) were cultured in RPMI-1640 with 10% (v/v) foetal bovine serum and 1% (v/v) penicillin–streptomycin solution under 37 °C and 5% CO2 condition.
Female nude mice (BALB/C) (16 ± 2 g) were bought from the Shanghai SLAC Laboratory Animals Co. Ltd (Shanghai, China) and raised in SPF condition. All animal protocols were approved by the Institutional Animal Care and Committee of Suzhou Polytechnic Institute of Agriculture and were in compliance with the Guidelines for the Care and Use of Laboratory Animals and ‘ARRIVE’ guidelines.
Tumour bearing mouse models used in the targeted effect and antitumor effect sections were constructed by subcutaneous injection of 4T1 in abdomen of Balb/c mice at a density of 106 cells/mouse (0.1 ml in FBS-free medium). Tumour sizes were measured every 2 days and tumour volumes were calculated with the following equation: V = L × W2/2, where V refers to the volume of the tumour; L and W represent the tumour size at the longest dimension as well as widest point, respectively.
Synthesis of amphipathic polymers
The amphiphilic polymer HA-DA was synthesized via the amidation reaction between HA and DA [Citation25]. Briefly, 388 mg (0.5 mmol) HA was dissolved in 30 ml distilled water and EDC·HCl (192 mg, 1 mmol)/NHS (115 mg, 1 mmol) was added to activate the carboxyl groups of HA for 1 h at 45 °C. 92 mg (0.5 mmol) DA in 20 ml ethanol was then added into the HA solution, and the reaction proceed at 45 °C for 24 h. The products were dialyzed against distilled water for 2 days to remove byproducts, followed by lyophilizing to get HA-DA.
Then, His was conjugated to HA-DA under the catalysis of EDC and NHS [Citation26]. In brief, 194 mg (0.5 mmol) HA-DA was dissolved in 10 ml distilled water and the carboxyl groups were activated by EDC·HCl (96 mg, 0.5 mmol)/NHS (57.5 mg, 0.5 mmol). 155 mg His was then added and the mixture was stirred for 24 h at 45 °C. Pure His-HA-DA polymer was obtained by dialyzing and lyophilizing.
The polymers were dissolved in D2O and the chemical structures were explored using 1H NMR spectra. The degree of substitution (DS) which is defined as the number of DA or His molecules per 100 sugar residues of HA was examined via 1H NMR spectroscopy.
Preparation and pH-response study of DOX/HHD
CMC values of HHD under different pH values
CMC values of HHD under different pH values were determined via the pyrene probe method [Citation27]. In brief, pyrene solution in methanol (10−6 mol/l) was added into centrifuge tubes, and the organic solvent was removed under rotary evaporator. HHD in PBS (10 ml, pH 7.4 or 5.3) with concentration ranging from 0.39 to 100 μg/mL was then added into the tubes and shaken at 37 °C. 12 h later, the fluorescence intensity was measured by a fluorescence spectrophotometer (λex = 336 nm, λem = 373 or 384 nm).
Preparation and characterization of DOX-loaded micelles
DOX-loaded HA-DA or His-HA-DA micelles (DOX/HD or DOX/HHD) were prepared with the dialysis method. 5 ml polymer solution (2 mg/mL) was mixed with 1 ml DOX·HCl (5 mg/mL), and 100 μL TEA was added. The solution was dialysed against distilled water so as to remove TEA and free DOX, followed by filtering through 0.22 μm membrane, and the DOX-loaded micelles were obtained () [Citation28] (Wavelengths used for DOX quantification were: λex = 480nm, λem = 590nm).
Table 1. Characteristic of DOX-loaded micelles.
The particle size and zeta potential of DOX-loaded micelles under different pH values were determined via a dynamic laser scanning (DLS) method. The EE% and DLC% of DOX-loaded micelles at pH 7.4 were explored via the ultrafiltration method. Besides, the concentration of DOX was determined with a fluorescence spectrophotometer [Citation29]. The EE% and DLC% of DOX-loaded micelles were measured with the equations as follows:
(1)
(1)
(2)
(2)
where w0 and w1 refer to the amounts of loaded DOX and the total weight of DOX in solution, respectively, and w refers to the total weight of DOX-loaded NPs.
Dox release from DOX micelles
The DOX release behaviour from DOX/HD or DOX/HHD was investigated via the dialysis method. Briefly, 5 ml DOX in different formulations (200 μg/mL) was placed in dialysis bags, which were dialyzed against 100 ml PBS (pH 7.4 or 5.3) at 37 °C, 100 r/min. At designed intervals, 1 ml release medium was taken out to determine the fluorescence intensity. Soon afterwards, the equal fresh medium was added.
Cellular uptake of DOX in different formulations
Confocal laser scanning microscopy (CLSM) was used to study the cellular uptake of DOX-loaded micelles. 4T1 cells were seeded in six-well plates at a density of 105 cells/well and cultured for 24 h, followed by incubating with DOX/HHD or DOX/HD (concentration of DOX was 10 μg/mL) for 2 h. The cultured cells were then fixed with 4% paraformaldehyde and nuclei were stained with Hoechst 33258. The intracellular distribution of DOX was then observed via CLSM (40×).
The quantitative uptake of DOX-loaded micelles was explored using flow cytometry. 4T1 cells were seeded in six-well plates at a density of 105 cells/well and cultured for 24 h (). Later, these cells were incubated with DOX in different formulations (concentration of DOX was 10 μg/mL) for 2 h, followed by washing with PBS for three times and determining the intracellular DOX via flow cytometry.
Table 2. IC50 of DOX in different formulations against 4T1 cells.
Intracellular distribution and tracking of DOX-loaded micelles
Intracellular distribution and tracking of DOX-loaded micelles were studied by CLSM. In brief, DOX/HHD or DOX/HD was added to 4T1 cells in six-well plates and incubated for 2, 4, or 8 h. Then the cells were stained with LysoTrack-Green® and Hoechst 33258, all of which were observed under CLSM after fixing with paraformaldehyde (63×).
Acridine orange (AO) staining was utilised to evaluate the integrity of the lysosomes after the treatment of micelles. Cultured 4T1 cells in 12-well plate were incubated with blank micelles (HHD-Mic or HD-Mic) for 0, 2, or 4 h, followed by washing with PBS for three times. These cells were then fixed with 4% paraformaldehyde for 10 min and stained with AO (6 μM) for 15 min, followed by observing under fluorescence microscopy (20×).
Cytotoxicity of DOX-loaded micelles
The cytotoxicity of DOX in different formulations against the tumour cells or normal cells was investigated via an MTT assay [Citation30]. In brief, 4T1 cells or normal cells were seeded in 96-well plates and incubated for 24 h, followed by removing the culture medium and adding fresh medium containing DOX·HCl, DOX/HD or DOX/HHD in various concentrations. After incubating for 24 or 48 h, 20 μL MTT (5 μg/mL) was then added to every well and incubated for another 4 h. The medium was removed later and 150 μL dimethyl sulfoxide (DMSO) was added, followed by measuring the absorbance at 570 nm with a microplate reader. The cytotoxicity of different formulations against 4T1 cells was measured according to the following equation:
(3)
(3)
where A570(treated) refers to the absorbance of cells treated with DOX formulations at 570 nm; A570(non-treated) stands for the absorbance of cells in non-treated group, and A0 is the absorbance of cells in non-cell group.
Penetration of DOX-loaded micelles in 3D tumour spheroids
As reported previously, 3 D tumour spheroids were prepared successfully [Citation26]. In brief, 100 μL per well of low melting point agarose was added to cover the 96-well plates to form a groove. 4T1 cells were then seeded in the groove at a density of 500 cells per well, followed by obtaining the 3 D tumour spheroids after cultured for about 6 days. 3D tumour spheres were then transferred to glass-bottom dishes and incubated with DOX/HHD or DOX/HD (concentration of DOX was 5 μg/mL) for 2 or 12 h. The penetration of DOX-loaded micelles was observed using CLSM (10×) with a ‘Z-stack’ function in 3 D tumour spheroids.
Tumour-targeting efficiency in vivo
Near infra-red fluorescence (NIRF) imaging to study the tumour-targeting efficiency
Tumour-targeting efficiency of micelles was explored by a NIRF imaging system with DiR as a fluorescent probe [Citation31]. DiR-loaded micelles (DiR/HD or DiR/HHD) were prepared via the emulsification-solvent evaporation method and tumour-bearing mice were prepared as mentioned above. As the volume of tumour reached 100–150 mm3, mice were intravenously injected with DiR/HD or DiR/HHD and observed under a NIRF imaging system at designed time points. After 48 h, the mice were sacrificed and main organs and tumours were excised for NIR imaging.
Biodistribution of DOX in vivo
Tumour-bearing mice were injected with DOX in various formulations at a dose of 7.5 mg/kg. After 24 h, the mice were sacrificed and the tumours and main organs namely heart, liver, spleen, lung and kidneys were excised for halogenation. The DOX in tumours and major organs were extracted with the mixed organic solvents (chloroform: methanol = 3:1, v/v) and the concentration of DOX was measured with a multi-functional microplate reader [Citation30].
Anti-tumour effect of DOX-loaded micelles
Mice bearing subcutaneous 4T1 tumour model were prepared as mentioned above. The mice were randomly divided into four groups with five mice for each group, namely saline, DOX•HCl, DOX/HD and DOX/HHD group. The first dose of four formulations was injected intravenously via the tail vein when reaching a volume of 100 mm3. Other three doses of DOX formulations were injected every 4 days (Days 5, 9, 13). The volume of tumours and body weight were measured every other day. On Day 21, the mice were sacrificed to harvest the tumour and major organs for the HE analysis.
Statistical analysis
All the experiments were conducted at least three times and all data were presented as mean ± standard deviation (SD). Obtained results were analyzed by ANOVA and the Student’s t-test was utilized for pair-wise comparisons. Statistical significance was showed as ***p < .001, **p < .01 and *p < .5.
Results and discussion
Synthesis and structural characterization
Firstly, the polymer His-HA-DA was synthesized with HA used as a hydrophilic segment and DA selected as a hydrophobic segment, while His was connected to the polymer as a pH-responsive segment. The structure of HA, HA-DA and His-HA-DA was, respectively, characterized via the 1H NMR spectra, as depicted in . As for His-HA-DA, δ3.0–4.0 ppm referred to the hydrogen of HA; δ 2.7 ppm referred to the hydrogen of DA; δ 7.4 ppm referred to the imidazole group of His. It suggested that the polymer was synthesized successfully and the DS values of DA and His conjugated to HA were 32.5 and 23.1%, respectively, according to the integral areas of the characteristic peaks.
Preparation and pH-responsive study of the DOX-loaded micelles
To evaluate whether the polymer could form into micelles, the CMC values of His–HA–DA under different pH values were determined with the pyrene as a fluorescent probe. As shown in , under physiological conditions (pH 7.4), His–HA–DA exhibited a low CMC value of 6.37 × 10−4 mg/mL, indicating that His-HA-DA could self-assemble into polymer micelles which were resistant to the dilution during blood circulation. However, as pH decreased to 5.3, the CMC value of His-HA-DA increased to 4.57 × 10−3 mg/mL, which demonstrated that the polymer was easy to be disassembled in acidic conditions [Citation32,Citation33].
Figure 2. pH-responsive study of DOX/HHD. (A). CMC of HHD at pH 7.4 or 5.3; (B). Particle size of DOX/HD and DOX/HHD; (C). Particle size of DOX/HHD at pH 7.4 or 5.3; (D). Zeta potential of DOX/HHD at pH 7.4 or 5.3; (E). EE% of DOX/HHD at pH 7.4 or 5.3; (F). Release of DOX from DOX.HCl, DOX/HD or DOX/HHD at pH 7.4 or 5.3.
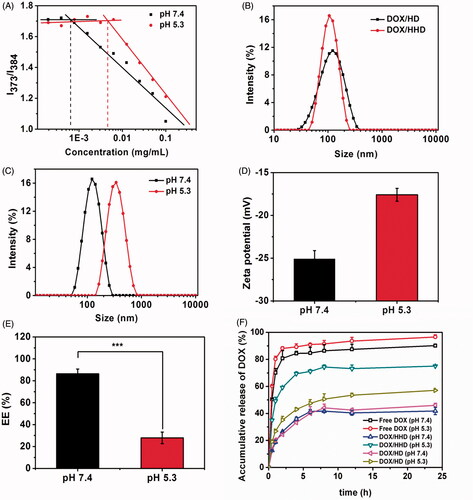
Then DOX-loaded micelles DOX/HD and DOX/HHD were prepared via the dialysis method in which DOX·HCl was desalted with TEA and loaded into the hydrophobic core of the micelles. As shown in , DOX/HD and DOX/HHD exhibited similar particle size, PDI and zeta potential, as well as EE% and DL%. Therefore, the non-pH-responsive DOX/HD was used as a control group in the following tests.
To explore the pH-responsive behaviour of DOX/HHD, the particle size, zeta potential, DOX intensity and DOX release in vitro under pH 7.4 or 5.3 conditions were investigated. As shown in , compared with that at pH 7.4, DOX/HHD exhibited a much larger particle size but similar PDI at pH 5.3. Besides, as pH decreased from 7.4 to 5.3, the zeta potential of DOX/HHD increased from −26.5 ± 0.5 to −7.4 ± 1.3 mV. It suggested that under the acidic conditions, the His segment of the polymer micelles was protonated, which led to the expanding of micelles and increasing of surface charge [Citation34]. The EE% of DOX/HHD in different pH conditions was shown in where DOX/HHD exhibited a significant decrease in EE% with pH increased from 7.4 to 5.3, demonstrating the disclosure of DOX from micelles under acidic conditions. The DOX release study also suggested that DOX/HHD exhibited a sustained release of payloads at pH 7.4, but a rapid release of DOX at pH 5.3. This on-demand drug release behaviour was favourable to reduce the drug release before reaching the targeted sites and release the payloads rapidly within tumour cells. On the contrary, DOX·HCl displayed rapid release of DOX at both pH 7.4 and pH 5.3, and DOX/HD revealed slow drug release at both pH 7.4 and pH 5.3.
Cellular uptake and intracellular distribution of DOX
To achieve satisfactory cytotoxicity, DOX-loaded micelles should be internalized into cells to ensure the intracellular DOX concentration. As shown in , after 2 h incubation, DOX/HHD or DOX/HD-treated cells exhibited relatively high DOX intensity in the cytoplasm. However, the uptake was significantly inhibited by pre-incubation with free HA. Later, the quantitatively cellular uptake of DOX in different formulations was investigated via flow cytometry. After 2 h of incubation, DOX/HD and DOX/HHD exhibited a higher cellular uptake than DOX•HCl, suggesting that loading into polymer micelles could help to enhance the intracellular drug concentration. In addition, the cellular uptake of the pH-responsive DOX/HHD was similar to that of non-sensitive DOX/HD, which indicated that the modification with His did not influence the cellular uptake. Consistent with the CLSM study, 4T1 cells exhibited an inhibited cellular uptake of DOX/HHD after pre-incubation with free HA, demonstrating that the uptake of DOX/HHD was obtained via the HA-CD44 receptor-mediated endocytosis [Citation35].
Intracellular tracking of DOX-loaded micelles
To achieve effective delivery, DOX was expected to release from micelles and diffuse to nuclei to induce cell apoptosis after internalization. The DOX-loaded micelles were incubated with cells for designed time and the intracellular distribution of DOX was investigated using CLSM. As shown in , incubated for 2 h, DOX/HHD co-located with endo/lysosomes, which provided the polymer micelles with an acidic microenvironment where the pH-triggered DOX release occurred. However, after incubated for 4 h, the pH-responsive DOX/HHD partly separated from endo/lysosomes and diffused to nuclei. At 8 h, DOX/HHD exhibited red fluorescence within the whole tumour cell and partially co-located with nuclei, which suggested that DOX released from polymer micelles in acidic conditions. On the contrary, DOX/HD was mainly located in cytoplasm within 4 h, and few DOX diffused to nuclei as time prolonged to 8 h. Then, the membrane integrity of the endo/lysosomes was further investigated by AO staining after incubation with HHD-Mic or HD-Mic. As shown in , cells treated with HHD-Mic exhibited green fluorescence after 2 or 4 h incubation, indicating that the pH-responsive micelles were able to disrupt endo/lysosome membranes, possibly resulting from the proton sponge effect of His groups. However, cells treated with HD-Mic displayed overlapped yellow fluorescence between red and green fluorescence, suggesting that HD-Mic had no influence on the integrity of the lysosomes. Overall, it was assumed that DOX firstly released from DOX/HHD in response to the endo/lysosomal low pH and then escaped from endosomes via the proton sponge effect, which protected DOX from enzyme degradation and was beneficial to the therapeutic effect.
Cytotoxicity of DOX-loaded micelles against 4T1 cells
The cytotoxicity of DOX/HD and DOX/HHD against 4T1 cell lines in vitro after incubated for 24 h or 48 h with or without free HA was investigated via the MTT assay and the obtained results were shown in . The cytotoxicity of different DOX formulations exhibited concentration-dependent and time-dependent features. Importantly, much higher cytotoxicity was observed in cells treated with DOX/HHD than DOX/HD, revealing that pH-responsive segment modification might induce the endo/lysosomal pH-triggered DOX release along with the endosomal escape behaviours and finally led to stronger cell killing ability. In particular, the cytotoxicity of DOX/HHD was inhibited by free HA, which was consistent with the uptake study. The uptake study indicated that the internalization of DOX/HHD was achieved via the CD44 receptor-mediated endocytosis which could be saturated by pre-incubation with HA. Interestingly, although the cellular uptake was not that high, DOX·HCl exhibited higher cytotoxicity than DOX-loaded micelles owing to the rapid co-location with nuclei, which was also reported previously [Citation36].
Penetration of DOX-loaded micelles in 3 D tumour spheres
Nanoparticles, especially those with a negative surface charge, usually fail to kill the tumour cells in the deep regions due to the lacking of good penetration effect [Citation37]. To investigate whether the pH-sensitive behaviour of micelles could help DOX to get into the deep areas of the tumour, 3 D tumour spheres were constructed to explore the penetration of DOX-loaded micelles. At 2 h, DOX/HD and DOX/HHD exhibited strong fluorescence intensity on the edge areas but relatively weak fluorescence intensity on the deep regions of the 3D tumour spheres, indicating that DOX-loaded micelles had relatively low penetration efficiency after 2 h incubation. Several region of interest (ROIs) in the centre of each figure were selected and the DOX intensity was measured [Citation38], as depicted in . Incubated for 2 h, the DOX intensity of DOX/HHD treated tumour spheres was similar to that of DOX/HD treated tumour spheres at every depth, which can be explained by the fact that DOX/HHD and DOX/HD exhibited similar cellular uptake. Nevertheless, as incubation time prolonged to 12 h, DOX/HHD exhibited significantly higher intensity at deep areas than DOX/HD, suggesting that the pH-responsive DOX/HHD was favourable to penetrate the tumour tissues. Additionally, it indicated that with the rapid DOX release triggered by intracellular low pH, DOX/HHD which diffused to not only the nuclei but also the deep regions of tumour tissues, could improve the penetration efficiency and anti-tumour effect of DOX.
Tumour–target effect of polymer micelles in vivo
Tumour–target effect is one of the most important factors for the application of polymer micelles in vivo since polymer micelles accumulate in tumour tissues selectively so that they would be internalized. Therefore, the tumour-target effect and DOX biodistribution in vivo were investigated via a NIR imaging system. DiR, a NIR fluorescence probe, was used to determine the real-time distribution of polymer micelles in tumour bearing mice. As shown in , both DiR/HD and DiR/HHD exhibited huge accumulation in tumour tissues, indicating that these polymer micelles with suitable particle size and the active targeted HA surface obtained great tumour-targeted efficiency via the EPR effect, as well as the HA-CD44 receptor-mediated active targeting effect. However, the tumour-targeted efficiency of DiR/HHD was significantly inhibited when pre-injected with free HA, suggesting that the specific combination between HA and tumour CD44 receptor played an essential role in the tumour target of these polymer micelles. The DiR fluorescence intensity at tumour tissues was measured 48 h post-injection, and the DiR/HHD group exhibited higher fluorescence intensity in tumour than DiR/HD group, indicating that the pH-responsive HHD micelles exhibited a better tumour-targeted efficiency because of the better penetration into tumour tissues. In particular, DiR fluorescence was also found in liver and spleen, as a result of the capture of micelles by reticular endothelial system (RES) [Citation39].
Figure 7. tumour-Target effect of polymeric micelles in vivo. (A) Real time NIR imaging of DiR-loaded polymer micelles in vivo; (B) DiR fluorescence intensity in tumour tissues over time; (C) Biodistribution of DOX in tumour-bearing mice 24 h post-injection.
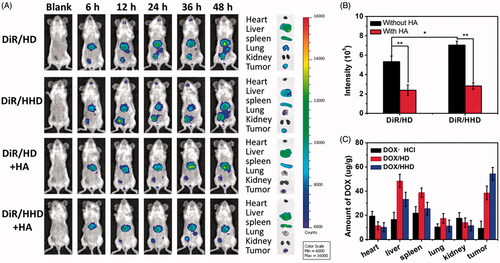
The quantitative biodistribution of DOX was determined by measuring the DOX concentration in tumour tissues and main organs 24 h after the injection of DOX in different formulations. As shown in , DOX-loaded micelles exhibited significant accumulation in tumour tissues and DOX/HHD delivered more DOX to tumour than DOX/HD, which was consistent with the target effect studied by NIR real-time imaging. However, DOX·HCl exhibited less accumulation in tumour tissues while more accumulation in heart tissues owing to the lacking of tumour-targeted efficiency. These achieved results indicated that encapsulated polymer micelles, especially pH-responsive HHD micelles, may improve the anti-tumour effect of DOX and reduce the cardiac toxicity in vivo as well.
Anti-tumour effect of DOX-loaded polymeric micelles in vivo
DOX in different formulations was injected into tumour-bearing mice and the anti-tumour effect was monitored by measuring the volume of tumour in each group. Meanwhile, the systemic toxicity was determined by measuring the body weight of mice. Compared with the saline group, DOX in various formulations exhibited significant inhibition of tumour growth. Moreover, DOX-loaded micelles exhibited a better anti-tumour effect than DOX·HCl due to the tumour-targeted efficiency and satisfactory cellular uptake in vivo. Of the two kinds of DOX-loaded micelles, the pH-responsive DOX/HHD exhibited a higher tumour growth inhibition rate than non-responsive DOX/HD. As shown in the HE staining figures (), DOX/HHD with the best inhibition on tumour growth also led to the most significant apoptosis in tumour tissue. DOX·HCl, without selected accumulation in tumour tissues, exhibited a poor anti-tumour effect but a great loss in body weight. In addition, DOX-loaded micelles exhibited reduced systemic toxicity than free DOX, since weight loss was not found in micelles groups [Citation40]. During the treatment period, the injection of DOX in different formulations did not cause apoptosis in the mouse main organs namely heart, liver, spleen, lung and kidney. In summary, the pH-responsive polymer micelles exhibited excellent tumour-targeted efficiency in vivo, good tumour tissue penetration into deep regions, satisfactory tumour cellular uptake and on-demand intracellular DOX release, leading to the improved anti-tumour effect and reduced side effects.
Conclusion
In this study, novel CD44-targeted pH-responsive polymer micelles formed from His-HA-DA were prepared for the delivery of DOX to breast cancer. These micelles with nano-size accumulated in tumour tissues via the EPR effect, followed by cellular uptake via the CD44-mediated endocytosis. DOX released rapidly from the micelles triggered by the acidic intracellular environment, escaped from the end/lysosomes and diffused to the nuclei, leading to the enhanced anti-tumour effect. In conclusion, these micelles with CD44-mediated active targeted efficiency and intracellular acid triggered drug release behaviour, could provide a promising strategy for the targeted delivery of anti-tumour agents in vivo.
Ethical approval
This article does not contain any studies with human participants or animals performed by any of the authors.
Informed consent
Informed consent was obtained from all individual participants included in the study.
Disclosure statement
No potential conflict of interest was reported by the author(s).
Additional information
Funding
References
- Jin X, Zhou J, Zhang Z, et al. Doxorubicin combined with betulinic acid or lonidamine in RGD ligand-targeted pH-sensitive micellar system for ovarian cancer treatment. Int J Pharm. 2019;571:118751.
- Setayesh A, Bagheri F, Boddohi S. Self-assembled formation of chondroitin sulfate-based micellar nanogel for curcumin delivery to breast cancer cells. Int J Biol Macromol. 2020;161:771–778.
- Sun X, Wang G, Zhang H, et al. The blood clearance kinetics and pathway of polymeric micelles in cancer drug delivery. ACS Nano. 2018;12(6):6179–6192.
- Talelli M, Barz M, Rijcken CJ, et al. Core-crosslinked polymeric micelles: principles, preparation, biomedical applications and clinical translation. Nano Today. 2015;10(1):93–117.
- Eetezadi S, Ekdawi SN, Allen C. The challenges facing block copolymer micelles for cancer therapy: In vivo barriers and clinical translation. Adv Drug Deliv Rev. 2015;91:7–22.
- Wicki A, Witzigmann D, Balasubramanian V, et al. Nanomedicine in cancer therapy: challenges, opportunities, and clinical applications. J Control Release. 2015;200:138–157.
- Pan J, Lei S, Chang L, et al. Smart pH-responsive nanoparticles in a model tumor microenvironment for enhanced cellular uptake. J Mater Sci. 2019;54(2):1692–1702.
- Zhou L, Xi Y, Chen M, et al. A highly antibacterial polymeric hybrid micelle with efficiently targeted anticancer siRNA delivery and anti-infection in vitro/in vivo. Nanoscale. 2018;10(36):17304–17317.
- Emtiazi G, Zohrabi T, Lee LY, et al. Covalent diphenylalanine peptide nanotube conjugated to folic acid/magnetic nanoparticles for anti-cancer drug delivery. J Drug Delivery Sci Technol. 2017;41:90–98.
- Hijaz M, Das S, Mert I, et al. Folic acid tagged nanoceria as a novel therapeutic agent in ovarian cancer. BMC Cancer. 2016;16:220.
- Li Y, Chen M, Yao B, et al. Transferrin receptor-targeted redox/pH-sensitive podophyllotoxin prodrug micelles for multidrug-resistant breast cancer therapy. J Mater Chem B. 2019;7(38):5814–5824.
- Debele TA, Yu LY, Yang CS, et al. pH- and GSH-sensitive hyaluronic acid-MP conjugate micelles for intracellular delivery of doxorubicin to colon cancer cells and cancer stem cells. Biomacromolecules. 2018;19(9):3725–3737.
- Wang Q, Zhong Y, Liu W, et al. Enhanced chemotherapeutic efficacy of the low-dose doxorubicin in breast cancer via nanoparticle delivery system crosslinked hyaluronic acid. Drug Deliv. 2019;26(1):12–22.
- Qiu L, Hu Q, Cheng L, et al. cRGDyK modified pH responsive nanoparticles for specific intracellular delivery of doxorubicin. Acta Biomater. 2016;30:285–298.
- Harrison EB, Azam SH, Pecot CV. Targeting accessories to the crime: nanoparticle nucleic acid delivery to the tumor microenvironment. Front Pharmacol. 2018;9:307.
- Zhang B, Hu Y, Pang Z. Modulating the tumor microenvironment to enhance tumor nanomedicine delivery. Front Pharmacol. 2017;8:952
- Kuchuk O, Tuccitto A, Citterio D, et al. pH regulators to target the tumor immune microenvironment in human hepatocellular carcinoma. Oncoimmunology. 2018;7(7):e1445452.
- Tang S, Meng Q, Sun H, et al. Dual pH-sensitive micelles with charge-switch for controlling cellular uptake and drug release to treat metastatic breast cancer. Biomaterials. 2017;114:44–53.
- Li X, Gao M, Xin K, et al. Singlet oxygen-responsive micelles for enhanced photodynamic therapy. J Control Release. 2017;260:12–21.
- Kanamala M, Wilson WR, Yang M, et al. Mechanisms and biomaterials in pH-responsive tumour targeted drug delivery: a review. Biomaterials. 2016;85:152–167.
- Mu Y, Wu G, Su C, et al. pH-sensitive amphiphilic chitosan-quercetin conjugate for intracellular delivery of doxorubicin enhancement. Carbohydr Polym. 2019;223:115072.
- Miao J, Yang XQ, Gao Z, et al. Redox-responsive chitosan oligosaccharide-SS-Octadecylamine polymeric carrier for efficient anti-Hepatitis B Virus gene therapy. Carbohydr Polym. 2019;212:215–221.
- Qu J, Peng S, Wang R, et al. Stepwise pH-sensitive and biodegradable polypeptide hybrid micelles for enhanced cellular internalization and efficient nuclear drug delivery. Colloids Surf B Biointerfaces. 2019;181:315–324.
- Ravar F, Saadat E, Gholami M, et al. Hyaluronic acid-coated liposomes for targeted delivery of paclitaxel, in-vitro characterization and in-vivo evaluation. J Control Release. 2016;229:10–22.
- Chen Y, Peng F, Song X, et al. Conjugation of paclitaxel to C-6 hexanediamine-modified hyaluronic acid for targeted drug delivery to enhance antitumor efficacy. Carbohydr Polym. 2018;181:150–158.
- Li F, Chen WL, You BG, et al. Enhanced cellular internalization and on-demand intracellular release of doxorubicin by stepwise pH-/reduction-responsive nanoparticles. ACS Appl Mater Interfaces. 2016;8(47):32146–32158.
- Muley P, Kumar S, El Kourati F, et al. Hydrophobically modified inulin as an amphiphilic carbohydrate polymer for micellar delivery of paclitaxel for intravenous route. Int J Pharm. 2016;500(1–2):32–41.
- Pan Y, Wang X, Yin Z. Synthesis and evaluation of cationic polymeric micelles as carriers of lumbrokinase for targeted thrombolysis. Asian J Pharm Sci. 2019;14(2):144–153.
- Wu Z, Chen B, Gan Z, et al. Exogenous vitamin C-triggered surface charge conversion of pH/reduction-responsive micelles for the enhanced tumor-specific activity of loaded doxorubicin. Mol Pharm. 2020;17(3):954–964.
- Chen WL, Yang SD, Li F, et al. Tumor microenvironment-responsive micelles for pinpointed intracellular release of doxorubicin and enhanced anti-cancer efficiency. Int J Pharm. 2016;511(2):728–740.
- Hsu JC, Naha PC, Lau KC, et al. An all-in-one nanoparticle (AION) contrast agent for breast cancer screening with DEM-CT-MRI-NIRF imaging. Nanoscale. 2018;10(36):17236–17248.
- Jafarzadeh-Holagh S, Hashemi-Najafabadi S, Shaki H, et al. Self-assembled and pH-sensitive mixed micelles as an intracellular doxorubicin delivery system. J Colloid Interface Sci. 2018;523:179–190.
- Li J, Liu Y, Li H, et al. pH-Sensitive micelles with mitochondria-targeted and aggregation-induced emission characterization: synthesis, cytotoxicity and biological applications. Biomater Sci. 2018;6(11):2998–3008.
- Liu Y, Zhou C, Wei S, et al. Paclitaxel delivered by CD44 receptor-targeting and endosomal pH sensitive dual functionalized hyaluronic acid micelles for multidrug resistance reversion. Colloids Surf B Biointerfaces. 2018;170:330–340.
- Zhong Y, Goltsche K, Cheng L, et al. Hyaluronic acid-shelled acid-activatable paclitaxel prodrug micelles effectively target and treat CD44-overexpressing human breast tumor xenografts in vivo. Biomaterials. 2016;84:250–261.
- Liu C, Guan Y, Su Y, et al. Surface charge switchable and core cross-linked polyurethane micelles as a reduction-triggered drug delivery system for cancer therapy. RSC Adv. 2017;7(18):11021–11029.
- Sun Q, Sun X, Ma X, et al. Integration of nanoassembly functions for an effective delivery cascade for cancer drugs. Adv Mater. 2014;26(45):7615–7621.
- Wang L, Lv Y, Li C, et al. Transformable dual-inhibition system effectively suppresses renal cancer metastasis through blocking endothelial cells and cancer stem cells. Small. 2020;16(40):e2004548.
- Zhang L, Zhou H, Belzile O, et al. Phosphatidylserine-targeted bimodal liposomal nanoparticles for in vivo imaging of breast cancer in mice. J Control Release. 2014;183:114–123.
- Sui J, Cui Y, Cai H, et al. Synergistic chemotherapeutic effect of sorafenib-loaded pullulan-Dox conjugate nanoparticles against murine breast carcinoma. Nanoscale. 2017;9(8):2755–2767.