ABSTRACT
Despite extensive research on Fe-based materials for soil cadmium(Cd) passivation, the combined effects with Ca agents on rice Cd migration remain unclear. This study examined the effects of NZVI, FeSO4 and their mixtures with CaCO3 on iron plaque formation and Cd translocation in rice plants grown in Cd-contaminated paddy soils. Results showed both NZVI and FeSO4 significantly increased rice biomass. FeSO4, NZVI, FeSO4+CaCO3, and NZVI+CaCO3 enhanced iron plaque by 65%, 72%, 77%, and 20%, respectively. Cd adsorption by iron plaque increased by 88% with FeSO4, 39% with NZVI, 49% with FeSO4+CaCO3, and decreased by 44% with NZVI+CaCO3. All treatments reduced Cd content in rice tissues, with brown rice Cd concentrations reduced by 66% with FeSO4, 58% with FeSO4+CaCO3, 45% with NZVI, and 39% with NZVI+CaCO3, respectively. This study highlights Fe-based amendments’ potential in safely utilizing Cd-contaminated farmlands, showing standalone Fe treatments outperform Fe-Ca combination for reducing Cd in brown rice.
KEYWORDS:
Introduction
Human activities lead to the entry of large amounts of heavy metals into soil [Citation1,Citation2]. The heavy metals entering the farmland often have a strong tendency to conceal themselves and are not easily detected. Heavy metal pollution in farmland soil not only has a negative impact on crop growth and yield [Citation3,Citation4], but also poses a threat to human health [Citation5]. Cadmium (Cd) pollution in farmland has been reported frequently [Citation6], and long-term consumption of foods with excess Cd can cause imperceptible damage to the liver, kidney, lung, bone, and cardiovascular system, leading to related diseases [Citation7–11]. Therefore, reducing Cd accumulation in crops is crucial. The ‘Cultivated Land Red-Line Restriction’ and the demographic pressures on China necessitate a cautious management approach for Cd-contaminated agricultural land. For farmland soils with light to moderate Cd pollution, implementing safe utilization measures can restrict the availability of Cd in the soil and reduce the concentration of Cd in crops [Citation12]. Through these methods, it is possible to maintain the original farming purpose of the cultivated land while also achieving the goal of potentially reducing the Cd content in the edible parts of crops to below the specified limit.
Rice is a major food crop, accounting for a considerable proportion of the world’s total food production and consumption [Citation13]. The practice of cultivating rice in soils with slight to moderate Cd contamination has proven to be an effective strategy for reducing the translocation of Cd to the aboveground portions of the rice plants. This method leverages the flooding conditions inherent to rice paddies, which promote the precipitation of CdS, thereby reducing the bioavailability of Cd in the soil [Citation14]. However, chronic exposure to Cd, primarily through daily consumption of rice, remains a predominant route of Cd ingestion for the human population [Citation15]. Consequently, strategies and interventions aimed at minimizing Cd uptake in rice have garnered significant attention within the research community.
In a flooded anaerobic environment, a reddish-brown iron oxide plaque (iron plaque) was formed on the surface of rice roots, which can adsorb heavy metals from the soil environment and decelerate the translocation and enrichment of heavy metals in rice plants to a certain extent [Citation16]. Research has shown that iron plaque-immobilised metal(loid) ions (Cd, As, Hg, and Cr) can reduce their accumulation in the aboveground rice parts [Citation16–18]. In contrast, iron plaque was also found to promote the migration and accumulation of Pb in rice grains [Citation19]. The reasons for this discrepancy may include factors such as rice variety, soil pH, heavy metal concentration, and Fe concentration supplied. However, previous studies have mostly focused on the rice seedling stage, whereas research on the effect of iron plaques on Cd accumulation in mature rice grains is relatively limited. Although it is necessary and meaningful to predict accumulation at mature-stage based on seedling experiments, the predicted results may not be always accurate. The iron plaque on the root surface of rice during the seedling stage is relatively newly formed and mostly remains as amorphous iron oxides, whereas those in aged plants have certain proportion of crystalline iron oxides, which can affect the adsorption and migration of heavy metals, and thereby affecting their distribution in plants [Citation20,Citation21]. Therefore, it is necessary to compare and explore the effects of iron plaque on the absorption and accumulation of Cd in rice during the seedling and mature stages. Rice can form iron plaques under natural soil conditions, but the amount of iron plaque formed is affected by the radial oxygen loss of the root and the Fe2+ present in the rhizosphere microenvironment [Citation22]. Applying an appropriate amount of Fe2+ to the soil can promote the formation of iron plaques on the root surfaces of rice plants [Citation23,Citation24]. The enhanced iron plaque will, to some extent, further inhibit the migration of heavy metals to the aboveground parts of plants [Citation16,Citation25]. The application of FeSO4 can supply plants with the essential microelement of Fe, promoting plant growth [Citation26], and can also reduce nitrogen loss during composting, enhancing the humification process [Citation27]. Meanwhile, numerous studies have indicated that the application of FeSO4 can increase the Fe content in the soil, thereby facilitating the formation of iron plaque on the surface of rice roots [Citation22]. Nano zerovalent iron (NZVI) has been used in wastewater treatment and polluted soil remediation owing to its large specific surface area, adsorption characteristics, and reducibility [Citation28]. Studies have shown that the application of NZVI can significantly reduce the availability of Cr(VI), Cd, and As in the soil, thereby decreasing their toxicity to plants [Citation29–33]. In addition to the adsorption effect for fixing heavy metals in soil, NZVI can undergo oxidation in the soil environment, releasing an appropriate amount of Fe2+, which has the potential to promote the formation of iron plaque on the root surface [Citation34]. It is clear that the use of these two Fe-based materials (FeSO4 and NZVI) has demonstrated promising results in the remediation of soil contaminated by heavy metals. The application of 100 μM Fe(III)-ethydiaminedhephen acetic (EDDHA) and Fe(II)-ethylenediaminetetra-acetic acid disodium salt (EDTANa2) also can effectively diminish the accumulation of Cd within the stems and leaves of rice plants grown in nutrient solution [Citation35]. However, the application of chelating agents such as EDDHA and EDTANa2 to soil has the potential to mobilize Cd, consequently, increasing the translocation of Cd within rice plants. Therefore, it is of greater necessity to investigate the application of FeSO4 and NZVI to understand their effects on the formation of iron plaque and the subsequent migration and accumulation of Cd in rice. This research can provide critical insights for the implementation of safe utilization strategies for Cd-contaminated agricultural land. In the safe utilization of farmland contaminated by Cd, the application of Ca-based passivation materials is relatively extensive, which can adjust soil pH, limit the availability of Cd, and reduce the absorption of Cd by plants [Citation36]. Do Ca-based and Fe-based materials, when combined, promote iron plaque formation, raise soil pH, and maximize Cd restriction, reducing Cd uptake by rice? To validate this conjecture, we conducted a pot experiment by adding NZVI, FeSO4, with or without CaCO3 to examine their effects on Fe plaque formation and the translocation of Cd during the seedling and mature stages of rice plants grown in a Cd-contaminated paddy soil.
Materials and methods
Soil collection and treatments
The soil was collected from a paddy field (longitude: 118.305168, latitude: 27.061412) in Jian’ou City, Fujian Province. Surface soil of 20 cm depth was collected and lumps were broken with a wooden hammer after air drying, and then passed through a 1 cm sieve. The soil pH and organic matter content were 6.4 and 11.2 g kg−1, respectively. The soil Cd content was 0.41 mg kg−1. The percentages of sand, loam, and clay soils were 45.2%, 48.2%, and 6.3%, respectively. We put 3.5 kg of soil in a plastic bucket after passing through 1 cm sieve to keep the 3 cm liquid level submerged. In total, there are 15 buckets of treatment. The solution containing 3.5 mg of Cd (as Cd(NO3) 2·4 H2O) was added to the plastic bucket, resulting in a final Cd content of 1.41 mg kg−1 in the soil. According to the Soil Environmental Quality Risk Control Standard for Soil Contamination of Agricultural Land (Trial) (GB15618–2018), the Cd concentration of 1.41 mg kg−1 in the soil falls between the risk screening value (Cd threshold (mg kg−1): 0.4; 5.5(soil pH ≤ 6.5) and the risk intervention value (Cd threshold (mg kg−1): 2.0; 5.5(soil pH ≤ 6.5), and this concentration was selected based on the practical issues of safe utilization of Cd-contaminated farmland. After one month, watering (tap water) was stopped and soil was left to dry naturally. Subsequently, all the soil was taken out, crushed with a wooden hammer, and passed through a 1 cm sieve before being placed back into the bucket. The objective of this step is to ensure that the Cd is evenly distributed throughout the soil. Among the buckets, NZVI powder was added to 3 buckets, a mixture of CaCO3 and NZVI powder was added to another 3, and CaCO3 powder was added to the remaining 3 buckets of soil. In detail, 3.5 kg of soil and 3.5 g of NZVI powder were measured and placed into a large polyethylene self-sealing bag, ensuring that the final concentration of NZVI in the soil reached the desired level of 1 g kg−1. Similarly, a CaCO3 addition of 2 g kg−1 was applied to the soil, whereas a combined treatment utilizing 2 g kg−1 of CaCO3 in conjunction with 1 g kg−1 of NZVI (NZVI+CaCO3) was also administered. Mixed soil was placed into a bucket and kept submerged. Meanwhile, exogenous base fertilizers N (0.2 g kg−1), P2O5 (0.15 g kg−1), and K2O (0.2 g kg−1) were added to the bucket. After 1 month, the rice seedlings (two seedlings per bucket) were transferred into buckets for growth. After 28 d of growth, 1 g kg−1 of Fe2+ solution (as FeSO4·7 H2O) was added to the bucket. The dosage of FeSO4·7 H2O was referred to the studies by Yu et al. [Citation24] and Liu et al. [Citation37], who found that a concentration of 1 g Fe kg−1 (as FeSO4·7 H2O) had no detrimental effect on rice growth. Li et al. [Citation38] demonstrated that the application of 0.5 g kg−1 of nano-iron oxide (Fe2O3) can promote the growth of rice plants. Therefore, for the purposes of this study, an exogenous Fe supplementation rate of 1 g Fe kg−1 was chosen for both FeSO4 and NZVI. The NZVI powder exhibits a uniform texture, enabling it to blend seamlessly with soil. Conversely, FeSO4·7 H2O, due to its heterogeneous nature and crystalline water content, fails to achieve a uniform mix with soil. Consequently, the application of this material necessitates the adoption of a solution addition method. To enhance the effectiveness of FeSO4 in promoting iron plaque formation and preventing Cd translocation into rice, we opted to incorporate FeSO4 as a solution into 6 buckets of soil (3 for FeSO4 treatment group and 3 for FeSO4+CaCO3 treatment group) two weeks prior to the rice tillering stage. There were five treatment groups: (1) control group, (2) FeSO4 treatment, (3) FeSO4+CaCO3 treatment, (4) NZVI treatment and (5) NZVI+CaCO3 treatment. Each treatment was repeated three times. The fertiliser was applied once on 70th d (panicle initiation stage) after transplanting at the same amount as the base fertilizer according to Ghaley [Citation39]. Soil pore water samples were collected using a Rhizon sampler (Rhizosphere Research Products, Wageningen, Netherlands) to determine the concentrations of Cd, Fe, Mn, Cu, and Zn on the 0, 30, 60, 90, and 120th d after transplanting.
Cultivation and transplantation of rice plants
The rice seeds (Yongyou1540) were soaked in 30% H2O2 for 30 min thoroughly rinsed with deionised (DI) water and placed in quartz sand for germination. The quartz sand was soaked in 5% dilute hydrochloric acid for 12 h and then rinsed with DI water until it was neutral. After 10 d, uniformly growing rice seedlings were transferred into a 15 L box with 1/5 strength Hoagland solution at a pH of 5.5. The culture solution was changed every 3 d. After 14 d, uniform seedlings were transplanted into buckets filled with paddy soil. Rice samples were collected at the tillering and mature stages at 42 and 154 d, respectively. The rice cultivation experiment was conducted in a greenhouse at 25–35°C with light exposure of 12–14 h d−1 in the Xia’an area of Fujian Agriculture and Forestry University.
Plant harvest and treatments
The rice roots and aboveground parts were cleaned with DI water after harvesting, and the roots, stems, leaves, and grains were separated using clean stainless steel scissors. The roots were placed in a clean glass beaker and iron plaque was extracted from the root surface according to Yu et al. [Citation24]. After extraction, the roots were washed with DI water and placed in a clean paper kraft envelope. Stem, leaf, and root samples were placed in an envelope in an oven at 105°C for 1 h. Subsequently, plant samples were baked at 70°C for 72 hrs, and dry weights were measured. The plant samples were then crushed using a stainless-steel grinder. After hulling, the rice was divided into husks and brown rice, which were powdered using a grinder. All plant samples were digested according to Yu et al. [Citation24], and the digested solutions were filtered through a 0.45 μm filter membrane for elemental analysis. The concentrations of Cd, Fe, Mn, Cu, and Zn in plant tissues, iron plaque extracts, and pore water were determined using inductively coupled plasma mass spectrometry (ICP-MS, NexION 300 ×; Perkin Elmer, NY). To ensure the accuracy and reliability of the digestion process, a reagent blank and a standard reference material (GBW07603, Chinese National Certified Reference Material) were processed alongside the samples, with the recovery rate consistently maintained between 95% and 105%. Soil samples were collected after harvesting, air-dried, ground naturally, passed through a 2 mm sieve, and pH was measured at a water-to-soil ratio of 2.5:1 according to Jin [Citation40] using a pH meter (Mettler Toledo, Switzerland).
Data processing and analysis
The data is presented in the form of mean ± SD (n = 3) and were analysed using the least significant difference (LSD) at the 5% level. A one-way analysis of variance (ANOVA) was performed using SPSS (19.0, SPSS, Inc., Chicago, IL, U.S.A.) to detect significant (p < 0.05) effects of FeSO4, FeSO4+CaCO3, NZVI, and NZVI+CaCO3 treatments on rice plant biomass, soil pH, and Cd, Fe, Mn, Cu, and Zn concentrations in brown rice, husks, leaves, stems, roots, iron plaque, and pore water samples.
The translocation factor (TF) for Cd within the rice plant, quantifying its movement from one tissue to another [Citation41], was determined using the following equation:
TFgrain/husk = grain Cd concentration/husk Cd concentration, TFhusk/leaf = husk Cd concentration/leaf Cd concentration, TFleaf/stem = leaf Cd concentration/stem Cd concentration, TFstem/root = stem Cd concentration/root Cd concentration, TFroot/iron plaque = root Cd concentration/iron plaque Cd concentration.
Results and discussion
Changes of dry weight in rice plant and soil pH
Compared to the control, the application of FeSO4 and NZVI increased the dry weight of the rice plants (). The addition of FeSO4 increased the dry weights of the roots, stems, leaves, and brown rice by 78% (p < 0.01), 49% (p < 0.001), 67% (p < 0.01), and 39% (p < 0.01), respectively. The dry weights of the roots, stems, leaves, and brown rice increased by 49% (p < 0.05), 18% (p < 0.05), 67% (p < 0.01), and 44% (p < 0.01), respectively by NZVI treatment. In comparison to the control group, the application of FeSO4+CaCO3 and NZVI+CaCO3 increased the dry weight of the leaves by 77% (p < 0.001) and 98% (p < 0.001), the stems by 9% and 14% (p < 0.05), and the roots by 20% and 8%, respectively ().
Figure 1. Effects of FeSO4 (1 g Fe kg−1), FeSO4+CaCO3 (1 g Fe kg−1 and 2 g kg−1 CaCO3), NZVI (1 g kg−1 NZVI), and NZVI+CaCO3 (1 g kg−1 NZVI and 2 g kg−1 CaCO3) on the dry weight of brown rice, husk, stem, leaf and root of rice plant grown in Cd-contaminated soil. Error bars represent the standard error (n = 3). Different letters indicate the mean difference is significant among treatments at the 0.05 level.
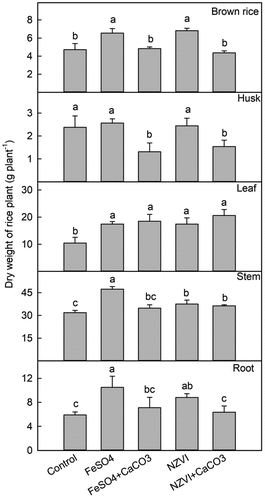
The soil pH decreased by 0.92 post-harvest as compared to the original soil (). The application of FeSO4, FeSO4+CaCO3, NZVI and NZVI+CaCO3 significantly increased soil pH by 0.61 (p < 0.01), 1.38 (p < 0.001), 1.12 (p < 0.001) and 1.87 (p < 0.001), respectively, compared with the control group.
Figure 2. Effects of FeSO4 (1 g Fe kg−1), FeSO4+CaCO3 (1 g Fe kg−1 and 2 g kg−1 CaCO3), NZVI (1 g kg−1 NZVI), and NZVI+CaCO3 (1 g kg−1 NZVI and 2 g kg−1 CaCO3) on soil pH after rice harvest. Error bars represent the standard error (n = 3). Different letters indicate the mean difference is significant among treatments at the 0.05 level.
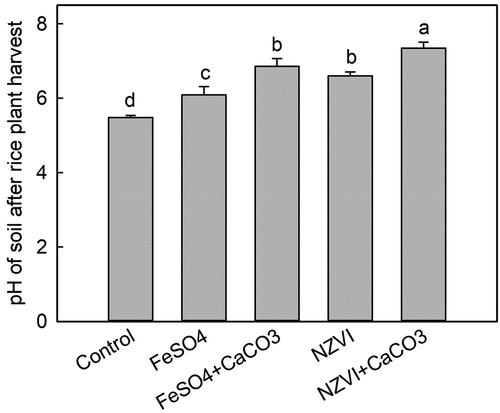
Cd, a heavy metal readily taken up by plants via their root systems, has the capacity to disturb the redox equilibrium within plant cells, which in turn impacts their growth and developmental processes [Citation42]. Upon entering the plant, Cd engages in a competitive interaction with essential ions, such as Ca, Fe, and Mn, for binding sites on transporter proteins [Citation43–45]. Cd is not required for plant growth, and it might modestly stimulate plant growth at trace levels [Citation46]. Nevertheless, when the concentration of Cd in soil exceeds a certain threshold, it can negatively impact plant development and result in decreased crop yields [Citation24,Citation47]. Siddique at al. [Citation48] indicated that both varieties of rice experienced a significant decline in the yield of straw and grains when subjected to Cd stress at a level of 1 mg kg−1. Emerging evidence has shown that appropriate amounts of Fe can promote plant growth and yield [Citation49–51]. Fe is an essential microelement for plant growth. Fe affects not only chlorophyll synthesis but also all organs that can capture light energy, including chloroplasts, chlorophyll protein complexes, and carotenoids [Citation52–54]. Fe exists in the electron transfer bonds of haemoglobin [Citation55]. In oxidation-reduction reactions, Fe can become Fe3+ or Fe2+, which bind to proteins in various forms within the plant body [Citation56]. It serves as an important electron transporter and catalyst and participates in physiological activities in plants. Therefore, the application of an appropriate amount of Fe is beneficial for plant growth, especially for crops growing in acidic soils. Yan et al. [Citation57] showed that an increase in soil pH promoted rice yield. When soil pH increased from 4.5 to 6.8, maximum rice yield was achieved [Citation57]. This is because rice plants have the highest absorption of N, P, and K at that pH [Citation57]. However, the combined application of FeSO4+CaCO3, as well as the use of NZVI+CaCO3, both elevated the soil pH to 6.86 and 7.35, respectively (), potentially impeding the translocation of nutrient ions from the soil solution to rice plants and thereby reducing the dry weight of husk and brown rice. In addition, the increase in Fe content in rice plants was crucial for increasing rice biomass (). Briat et al. [Citation58] showed that the application of an appropriate concentration of Fe was beneficial for the migration and enrichment of Fe in plant tissues, thereby promoting plant growth. Siddique et al. [Citation48] showed that the addition of 1 or 2 g kg−1 Fe (as FeSO4) increased the rice straw and grains yield, and this effect was more significant when rice plants were exposed to Cd at 1 or 3 mg kg−1.
Figure 3. Effects of FeSO4 (1 g Fe kg−1), FeSO4+CaCO3 (1 g Fe kg−1 and 2 g kg−1 CaCO3), NZVI (1 g kg−1 NZVI), and NZVI+CaCO3 (1 g kg−1 NZVI and 2 g kg−1 CaCO3) on the concentrations of Mn, Cu, and Zn in brown rice, husk, leaf, stem, root and iron plaque of rice plant grown in Cd-contaminated soil. Error bars represent the standard error (n = 3). Different letters indicate the mean difference is significant among treatments at the 0.05 level.
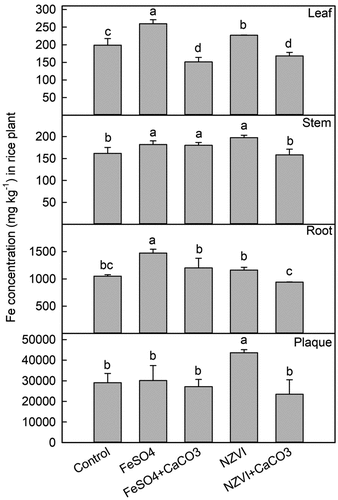
Figure 4. Effects of FeSO4 (1 g Fe kg−1), FeSO4+CaCO3 (1 g Fe kg−1 and 2 g kg−1 CaCO3), NZVI (1 g kg−1 NZVI), and NZVI+CaCO3 (1 g kg−1 NZVI and 2 g kg−1 CaCO3) on the concentrations of Fe in leaf, stem, root and iron plaque of rice plant grown in Cd-contaminated soil during the tillering stage. Error bars represent the standard error (n = 3). Different letters indicate the mean difference is significant among treatments at the 0.05 level.
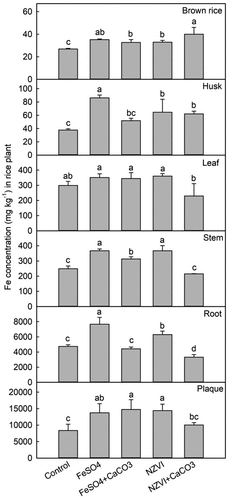
Effects of FeSO4/NZVI with or without CaCO3 on Fe concentration
During the tillering stage of rice, compared with the control group, no effect of FeSO4 was observed on root iron plaque, but it significantly increased the Fe concentration in root tissues, stems, and leaves (). The Fe concentrations in the root tissue, stem, and leaf increased by 40% (p < 0.001), 13% (p < 0.05), and 31% (p < 0.01), respectively upon FeSO4 addition. The application of FeSO4+CaCO3 notably enhanced the Fe concentration in rice stems by 11.34% (p < 0.05), while it markedly decreased the Fe concentration in the leaves by 23.99% (p < 0.001). The Fe concentrations in the iron plaque, root tissue, stem, and leaf significantly increased by 50% (p < 0.05), 11% (p < 0.05), 22% (p < 0.01), and 14% (p < 0.05), respectively, under the NZVI treatment. The application of NZVI+CaCO3 led to a significant 15.56% (p < 0.05) decrease in the Fe concentration of rice leaves.
During the maturation stage, the application of FeSO4 and NZVI significantly promoted the adsorption and absorption of Fe by rice plants (). Compared to other tissues, the Fe concentration was higher in the iron plaque and root tissue. The addition of FeSO4 increased the Fe concentrations in the iron plaque, root tissue, stem, leaf, rice husk, and brown rice by 65% (p < 0.05), 62% (p < 0.001), 48% (p < 0.001), 18% (p < 0.05), 129% (p < 0.01), and 31% (p < 0.001), respectively. The application of NZVI increased the Fe concentrations in the iron plaque, root tissue, stem, leaf, rice husk, and brown rice by 72% (p < 0.05), 33% (p < 0.05), 48% (p < 0.001), 21% (p < 0.05), 71% (p < 0.05), and 23% (p < 0.001), respectively. The FeSO4+CaCO3 treatment significantly enhanced the Fe concentrations in the iron plaque by 77% (p < 0.01), in the stem by 26% (p < 0.01), and in brown rice by 22% (p < 0.05), while it had no significant effect on the Fe concentrations in the root tissue, leaf, and husk. The NZVI+CaCO3 treatment significantly elevated Fe concentrations in husk and brown rice by 65% (p < 0.01) and 49% (p < 0.001), respectively, while reducing root tissue Fe by 30% (p < 0.01).
Over the 30- to 90-day period, compared to the control, FeSO4 addition significantly enhanced pore water Fe concentrations, with further significant increases observed at 30 and 90 days following the treatments with FeSO4+CaCO3 and NZVI (). The NZVI+CaCO3 treatment only enhanced the Fe concentration in the pore water for 90 days.
Figure 5. Effects of FeSO4 (1 g Fe kg−1), FeSO4+CaCO3 (1 g Fe kg−1 and 2 g kg−1 CaCO3), NZVI (1 g kg−1 NZVI), and NZVI+CaCO3 (1 g kg−1 NZVI and 2 g kg−1 CaCO3) on the concentrations of Fe in brown rice, husk, leaf, stem, root and iron plaque of rice plant grown in Cd-contaminated soil during the maturation stage. Error bars represent the standard error (n = 3). Different letters indicate the mean difference is significant among treatments at the 0.05 level.
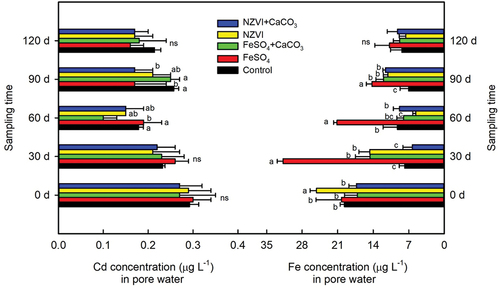
Our results indicate that the amount of iron plaque during the tillering stage was higher than that during the mature stage (). Yu et al. [Citation59], also reported maximum oxygen secretion by rice plants during the tillering stage, which could promote formation of iron plaque. Numerous studies have shown that within an appropriate application range, the addition of FeSO4 can promote the formation of iron plaques and increase the Fe content in plant tissues [Citation20,Citation23]. The application of FeSO4 promoted the concentration of Fe2+ in the rhizosphere (), which is conducive for enhanced iron plaque formation [Citation22]. In addition, long-term anaerobic flooding promotes the direct absorption of Fe2+ by rice roots from the surrounding rhizosphere zone through transport proteins such as OsYSL15 [Citation60]. NZVI can be oxidised to form Fe2+ under the action of oxygen [Citation61,Citation62], which provides more Fe sources for the formation of iron plaque and also explains the increased Fe concentration in the pore water observed in our results (). NZVI can further help to maintain a low redox potential under flooded conditions, which is beneficial for the dissolution and reduction of Fe2+ [Citation34]. These studies support our results that the application of both types of Fe significantly increases the formation of iron plaques and increases Fe content in rice plants.
Concentration and translocation of Cd in rice plant
During the rice tillering stage, the Cd concentration was highest in the root tissue (). The Cd concentration in the Fe plaque increased by 41% with FeSO4 (p < 0.05) and 33% with NZVI (p > 0.05), but the combined treatments of both Fe with CaCO3 did not significantly affect the Cd adsorbed by the iron plaque. Compared with the control group, FeSO4 increased the Cd concentrations in the root tissue by 55% (p < 0.05), whereas the remaining treatments did not significantly alter the Cd concentrations within the root tissue. The Cd concentration in the stem significantly decreased by 29% with FeSO4 (p < 0.05) and 34% with FeSO4+CaCO3 (p < 0.01), respectively. The Cd concentration in the leaf significantly decreased by 38% with NZVI (p < 0.05) and 46% with NZVI+CaCO3 (p < 0.05), respectively.
Figure 6. Effects of FeSO4 (1 g Fe kg−1), FeSO4+CaCO3 (1 g Fe kg−1 and 2 g kg−1 CaCO3), NZVI (1 g kg−1 NZVI), and NZVI+CaCO3 (1 g kg−1 NZVI and 2 g kg−1 CaCO3) on the concentrations of Fe and Cd in pore water of rhizosphere soil. Error bars represent the standard error (n = 3). Different letters indicate the mean difference is significant among treatments at the 0.05 level.
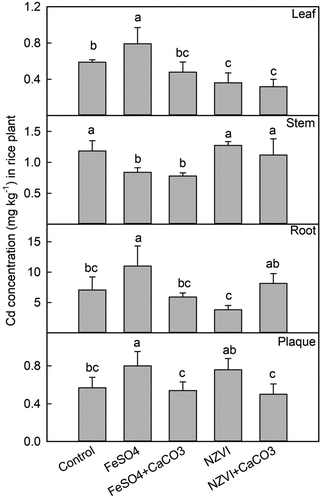
Figure 7. Effects of FeSO4 (1 g Fe kg−1), FeSO4+CaCO3 (1 g Fe kg−1 and 2 g kg−1 CaCO3), NZVI (1 g kg−1 NZVI), and NZVI+CaCO3 (1 g kg−1 NZVI and 2 g kg−1 CaCO3) on the concentrations of Cd in leaf, stem, root and iron plaque of rice plant grown in Cd-contaminated soil during the tillering stage. Error bars represent the standard error (n = 3). Different letters indicate the mean difference is significant among treatments at the 0.05 level.
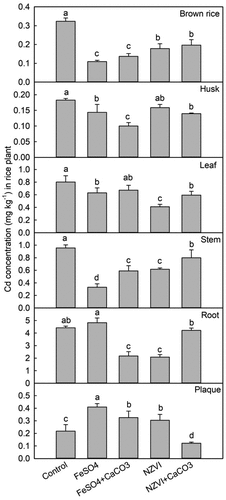
At the maturity stage, compared with the control, the Cd concentration in the iron plaque was significantly increased by 88% with FeSO4 (p < 0.001), by 49% with FeSO4+CaCO3 (p < 0.05), and 39% with NZVI (p < 0.05), respectively, but decreased by 44% with NZVI+CaCO3 (p < 0.05) (). Root Cd concentration was significantly reduced by 51% with FeSO4+CaCO3 (p < 0.001) and 53% with NZVI (p < 0.001), respectively. The Cd concentration in rice stems was significantly reduced by 66% with FeSO4 (p < 0.001), 38% with FeSO4+CaCO3 (p < 0.001), 36% with NZVI (p < 0.001), and 17% with NZVI+CaCO3 (p < 0.05), respectively. The Cd concentration in leaves was significantly reduced by 21% with FeSO4 (p < 0.05), 49% with NZVI (p < 0.001), and 26% with NZVI+CaCO3 (p < 0.01), respectively. The Cd concentration in rice husks was reduced by 21% with FeSO4 (p < 0.01), 45% with FeSO4+CaCO3 (p < 0.001), and 24% with NZVI+CaCO3 (p < 0.01), respectively. The Cd concentration in brown rice decreased significantly by 66% with FeSO4 (p < 0.001), 58% with FeSO4+CaCO3 (p < 0.001), 45% with NZVI (p < 0.001), and 39% with NZVI+CaCO3 (p < 0.001), respectively.
Figure 8. Effects of FeSO4 (1 g Fe kg−1), FeSO4+CaCO3 (1 g Fe kg−1 and 2 g kg−1 CaCO3), NZVI (1 g kg−1 NZVI), and NZVI+CaCO3 (1 g kg−1 NZVI and 2 g kg−1 CaCO3) on the concentrations of Cd in brown rice, husk, leaf, stem, root and iron plaque of rice plant grown in Cd-contaminated soil during the maturation stage. Error bars represent the standard error (n = 3). Different letters indicate the mean difference is significant among treatments at the 0.05 level.
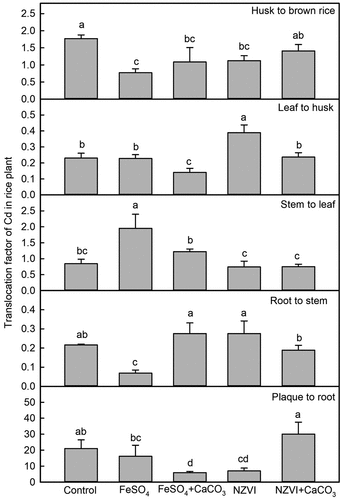
From 0 d to 120 d, the Cd concentration in the pore water gradually decreased (). In comparison to the control, the application of FeSO4+CaCO3 for 60 days, and NZVI+CaCO3 for 90 days, led to a decrease in Cd levels (p < 0.05) within the pore water.
Compared with the control, TFgrain/husk was significantly reduced by 56% with FeSO4 (p < 0.001), 23% with FeSO4+CaCO3 (p < 0.01), 37% with NZVI (p < 0.001), and 20% with NZVI+CaCO3 (p < 0.01), respectively (). TF husk/leaf was significantly reduced by 39% with FeSO4+CaCO3 (p < 0.01) and increased by 69% with NZVI (p < 0.001), respectively. The FeSO4 treatment significantly enhanced the TF leaf/stem by 131% (p < 0.001), and reduced TF stem/root by 68% (p < 0.01), respectively. TF root/iron plaque was significantly reduced by 72% with FeSO4+CaCO3 (p < 0.01).
In general, the Cd content in rice tissues during the tillering stage was higher than that during the mature stage (). The number of stem nodes in the rice plants varied during the tillering and maturity stages. Xia et al. [Citation63] showed that the Cd concentration in the first node (the topmost node) determines the accumulation of Cd in rice grains. Therefore, predicting the migration and enrichment of Cd in rice grains based on seedling experiments requires a comprehensive and careful consideration.
To adapt to the unfavourable anaerobic environments, wetland plants have evolved aeration tissues and efficient root metabolic systems [Citation64]. Iron plaque is a hydrated iron oxide formed during the evolution of wetland plants that can adsorb anions and cations from the environment [Citation22]. Rice, a wetland plant, can also form root iron plaques to adsorb heavy metals from the soil [Citation22]. The exogenous application of FeSO4 in soil promotes the formation of iron plaques [Citation24]. There are two possible reasons for the decrease in the Cd content in rice plants supplied with FeSO4 or NZVI. First, Fe plaques can adsorb Cd in the rhizosphere microenvironment. Iron plaque has been reported as an amphiphilic colloid that can adsorb Cd in the environment [Citation22], making it a barrier that restricts the migration and accumulation of Cd in rice plants. However, some studies minimal role of iron plaques in restricting Cd migration [Citation37], as the rice root Cd content is the highest, and the iron plaque Cd content is very low, which is consistent with the results of this study (). The second factor limiting the translocation of Cd from soil to rice is the competition between Fe and Cd for ion channel proteins. The application of FeSO4 or NZVI directly or indirectly provides more Fe2+ for plant uptake, which can compete with Cd2+ for ion channel proteins [Citation60,Citation65], thereby reducing the migration of Cd into the rice plants. In addition, our results showed that iron plaque induction promoted the absorption of Mn by rice grains, and that there was a significant negative correlation between Mn and Cd in the rice grains (). Yu et al. [Citation66] found that OsNramp5, a transporter protein, is responsible for transporting Cd and Mn in rice plants, implying competition between Cd and Mn in plant tissues. Another possible reason for reduced Cd accumulation under NZVI application in rice is the formation of Fe oxides in the soil environment for Cd adsorption. Following NZVI application, Fe (0) can be gradually oxidised to Fe3+ [Citation34], forming precipitates and allowing Cd to be adsorbed onto the surface of the iron oxide.
Figure 9. Cd translocation factor from iron plaque to root, root to stem, stem to leaf, leaf to husk, and husk to brown rice of rice plant grown in Cd-contaminated soil. Error bars represent the standard error (n = 3). Different letters indicate the mean difference is significant among treatments at the 0.05 level.
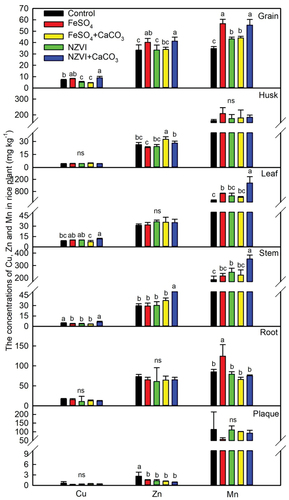
Figure 10. Correlation between concentrations of Cd in brown rice and the concentrations of Mn in brown rice, Cu in stems, and Zn in iron plaque of rice plants grown in Cd-contaminated soil.
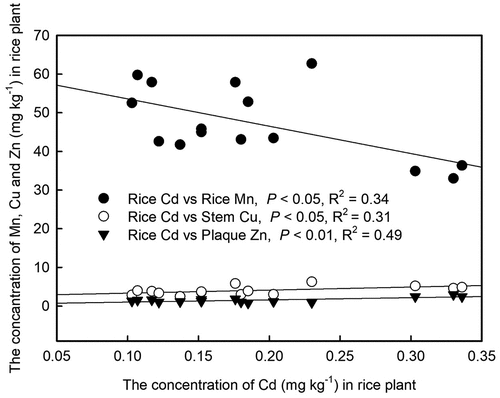
Our present study demonstrates that FeSO4 is superior in decreasing Cd levels in brown rice, likely due to the synergistic effects of multiple factors. Firstly, the Cd concentration in brown rice was positively correlated with that in the husk, but negatively correlated with the Fe concentration in the husk (). Additionally, brown rice Cd showed a negative correlation with stem Fe and a positive correlation with stem Fe (). Our finding showed that FeSO4 treatment increased Fe concentrations in rice stems and husks, potentially limiting the accumulation of Cd in brown rice (). Secondly, while soil pH levels did not significantly correlate with Cd content in brown rice (), there was a notable inverse relationship between soil pH and Cd levels in husk, which indirectly impacted Cd accumulation in the brown rice. Although the correlation between Fe and Cd in brown rice was not significant, the impact of Fe on Cd accumulation in brown rice should not be discounted. Zhang et al. [Citation67] revealed a significant inverse correlation between Fe content and Cd content in rice grain. Moreover, foliar Fe application has been found to elevate Fe content in brown rice while diminishing Cd levels, underscoring the significance of Fe in controlling Cd accumulation in rice grains [Citation68]. Our results revealed a significant negative correlation between Fe in iron plaque and Cd in brown rice (), aligning with Kong et al. [Citation69] who proposed that enhancing iron plaque formation can significantly reduce Cd migration to aerial parts. A significant negative correlation was demonstrated between Cd and Mn contents in brown rice, with the highest Mn levels observed in brown rice treated with FeSO4 (). This is in agreement with Zhao et al. [Citation70], who identified OsNRAMP1 as a membrane-localized transporter for both Mn and Cd, indicating the competitive uptake of these elements within the rice plant.
Figure 11. The Pearson correlation between soil pH and the concentrations of Fe and Cd in various tissues of rice plants. *means p < 0.05; **means p < 0.01; ***means p < 0.001.
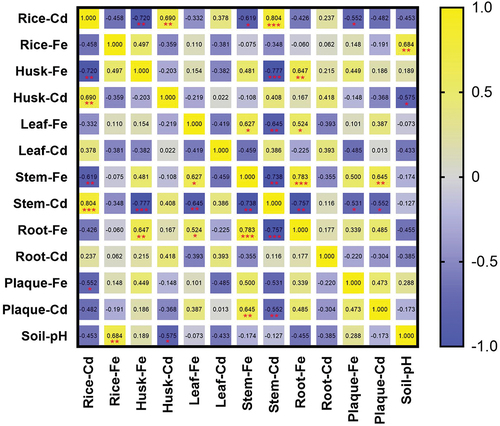
Our results showed that the combined application of CaCO3 and FeSO4/NZVI did not further enhance the effectiveness in reducing Cd content in brown rice compared to the use of FeSO4/NZVI alone. The reasons may be attributed to the amount and ratio of FeSO4/NZVI and CaCO3, as well as the timing of applying Fe to the soil. The amount of Fe utilized determined the distribution of Cd within rice plants. Under low Fe supply, the Fe content in rice plants decreased with increased Cd exposure, while elevated Fe supply maintained the Fe content regardless of further Cd exposure, significantly reducing Cd uptake and accumulation in rice plants [Citation43]. Kong et al. [Citation69] reported that Cd adsorbed onto iron plaques functioned as a source of Cd uptake by rice plants at iron plaque contents of 10–30 g/kg. Beyond this threshold, soil Cd was observed to bind firmly to the plaque surface, thereby decreasing the Cd uptake by the root tissues of the rice plants. Our findings indicate that, although the amount of iron plaque did not substantially alter with the FeSO4+CaCO3 treatment compared to FeSO4 alone, the Cd adsorbed to the iron plaque was diminished. Potentially, Cd desorbed from the iron plaque and re-migrated to the rice, resulting in an increase in Cd content in the rice grains, although the difference in Cd content in the brown rice compared to the control was not significant.
Similarly, the dosage of CaCO3 also plays a crucial role in the migration of Cd to rice. Dong et al. [Citation71] demonstrated that a dosage of only 32 mg kg−1 lime could significantly reduce Cd levels in brown rice compared to doses of 2, 4, 8, and 16 mg kg−1 lime. The co-application of 2 mg kg−1 CaCO3 with FeSO4, in contrast to FeSO4 alone, notably diminished Fe levels in the pore water but only decreased Cd concentrations in the pore water at 60 days, suggesting that this specific CaCO3 and FeSO4 combination did not substantially augment the reduction of Cd in brown rice (). Controlling the soil Cd availability during the rice filling stage is crucial for effectively reducing Cd accumulation in brown rice [Citation72]. Our present study confined the addition of FeSO4 to the rice tillering phase, neglecting the implications of FeSO4 application in isolation or in conjunction with CaCO3 at other growth stages on Cd accumulation in rice.
Conclusion
This study examined the impact of FeSO4/NZVI, with or without CaCO3, on iron plaque formation and Cd accumulation in rice plants cultivated in Cd-contaminated paddy soils. Results revealed that NZVI, FeSO4, and their combination with CaCO3 treatments significantly increased the pH of the soil. The amount of iron plaque at the tillering stage (23 − 44 g Fe kg−1) was higher than that at the maturation stage (8 − 15 g Fe kg−1). During the mature stage, the application of NZVI, FeSO4, and FeSO4+CaCO3 significantly increased the amount of iron plaque. At the mature stage, the Cd content adsorbed by iron plaque was enhanced by NZVI, FeSO4, and FeSO4+CaCO3 treatments, but decreased by NZVI+CaCO3. The four treatments diminished the Cd content across different tissues of the aerial parts in rice plants. The Cd content in brown rice was notably reduced by 66%, 58%, 45%, and 39% following treatments with FeSO4, FeSO4+CaCO3, NZVI, and NZVI+CaCO3, respectively, which was lower than the threshold values of the Chinese national food standard (0.2 mg kg−1). The correlation results indicated that the Cd content in brown rice was negatively correlated with Fe content in the rice stems and husks, Mn content in the husks, and positively correlated with Cd content in the stems and husks. These findings provide valuable insights into Cd accumulation in rice plants when using Fe-based materials, with or without CaCO3, and provide a critical reference for the safe utilization of farmland contaminated with Cd. In the future, the influence of variable dosages of exogenous Fe application, in conjunction with CaCO3 treatment, on the formation of iron plaques and the translocation of Cd from soil to the aboveground parts of rice plants across different growth stages requires clarification.
Acknowledgments
We sincerely appreciate the anonymous reviewers’ suggestions for revisions to this article.
Disclosure statement
No potential conflict of interest was reported by the author(s).
Additional information
Funding
References
- Wu Y, Li X, Yu L, et al. Review of soil heavy metal pollution in China: Spatial distribution, primary sources, and remediation alternatives. Resour Conserv Recycl. 2022;181:106261. doi: 10.1016/j.resconrec.2022.106261
- Napoletano P, Guezgouz N, Di Iorio E, et al. Anthropic impact on soil heavy metal contamination in riparian ecosystems of northern Algeria. Chemosphere. 2023;313:137522. doi: 10.1016/j.chemosphere.2022.137522
- Rashid A, J SB, Ulery A, et al. Heavy Metal Contamination in Agricultural Soil: Environmental Pollutants Affecting Crop Health. Agronomy. 2023;13(6):1521. doi: 10.3390/agronomy13061521
- Elango D, Devi KD, K JH, et al. Agronomic, breeding, and biotechnological interventions to mitigate heavy metal toxicity problems in agriculture. J Agri Food Res. 2022;10:100374. doi: 10.1016/j.jafr.2022.100374
- Briffa J, Sinagra E, Blundell R. Heavy metal pollution in the environment and their toxicological effects on humans. Heliyon. 2020;6(9):e04691. doi: 10.1016/j.heliyon.2020.e04691
- Tang X, Li Q, Wu M, et al. Review of remediation practices regarding cadmium-enriched farmland soil with particular reference to China. J Environ Manage. 2016;181:646–14. doi: 10.1016/j.jenvman.2016.08.043
- Xiong R, Wu Q, Trbojevich R, et al. Disease-related responses induced by cadmium in an in vitro human airway tissue model. Toxicol Lett. 2019;303:16–27. doi: 10.1016/j.toxlet.2018.12.009
- Wang X, Cui W, Wang M, et al. The association between life-time dietary cadmium intake from rice and chronic kidney disease. Ecotox Environ Safe. 2021;211:111933. doi: 10.1016/j.ecoenv.2021.111933
- Li Y, Chen C, Lu L, et al. Cadmium exposure in young adulthood is associated with risk of nonalcoholic fatty liver disease in midlife. Digest Dis Sci. 2022;67(2):689–696. doi: 10.1007/s10620-021-06869-8
- Obeng-Gyasi E. Chronic cadmium exposure and cardiovascular disease in adults. J Environ Sci Heal A. 2020;55(6):726–729. doi: 10.1080/10934529.2020.1737459
- Ma Y, Ran D, Shi X, et al. Cadmium toxicity: A role in bone cell function and teeth development. Sci Total Environ. 2021;769:144646. doi: 10.1016/j.scitotenv.2020.144646
- Feng Y, Lei Z, Tong X, et al. Spatially-explicit modeling and intensity analysis of China’s land use change 2000–2050. J Environ Manage. 2020;263:110407. doi: 10.1016/j.jenvman.2020.110407
- Chen W, Zhao X. Understanding global rice trade flows: network evolution and implications. Foods. 2023;12(17):3298. doi: 10.3390/foods12173298
- Y XX, P MS, A MA, et al. Growing rice aerobically markedly decreases arsenic accumulation. Environ Sci Technol. 2008;42(15):5574–5579. doi: 10.1021/es800324u
- Shi Z, Carey M, Meharg C, et al. Rice grain cadmium concentrations in the global supply-chain. Expos Health. 2020;12(4):869–876. doi: 10.1007/s12403-020-00349-6
- Zandi P, Yang J, Darma A, et al. Iron plaque formation, characteristics, and its role as a barrier and/or facilitator to heavy metal uptake in hydrophyte rice (Oryza sativa L.). Environ Geochem Hlth. 2023;45(3):525–559. doi: 10.1007/s10653-022-01246-4
- Xu B, Wang F, H ZQ, et al. Influence of iron plaque on the uptake and accumulation of chromium by rice (Oryza sativa L.) seedlings: Insights from hydroponic and soil cultivation. Ecotox Environ Safe. 2018;162:51–58. doi: 10.1016/j.ecoenv.2018.06.063
- Li Y, Zhao J, Zhang B, et al. The influence of iron plaque on the absorption, translocation and transformation of mercury in rice (Oryza sativa L.) seedlings exposed to different mercury species. Plant Soil. 2016;398(1):87–97. doi: 10.1007/s11104-015-2627-x
- Zou L, Zhang S, Duan D, et al. Effects of ferrous sulfate amendment and water management on rice growth and metal(loid) accumulation in arsenic and lead co-contaminated soil. Environ Sci Pollut R. 2018;25(9):8888–8902. doi: 10.1007/s11356-017-1175-8
- Xu B, Yu S. Root iron plaque formation and characteristics under N2 flushing and its effects on translocation of Zn and Cd in paddy rice seedlings (Oryza sativa). Ann Bot-London. 2013;111(6):1189–1195. doi: 10.1093/aob/mct072
- J LW, G ZY, Hu Y, et al. Arsenic sequestration in iron plaque, its accumulation and speciation in mature rice plants (Oryza sativa L.). Environ Sci Technol. 2006;40(18):5730–5736. doi: 10.1021/es060800v
- Khan N, Seshadri B, Bolan N, et al. Root iron plaque on wetland plants as a dynamic pool of nutrients and contaminants. Adv Agron. 2016;138:1–96. doi: 10.1016/bs.agron.2016.04.002
- Xu B, Y CJ, Yu JY, et al. Effects of 24-epibrassinolide and 28-homobrassinolide on iron plaque formation and the uptake of as and Cd by rice seedlings (Oryza sativa L.) in solution culture. Environ Technol Inno. 2020;19:19. doi: 10.1016/j.eti.2020.100802
- Yu J, Guo X, Luo Z, et al. Do brassinosteroids and iron plaque affect the accumulation of as and Cd in rice (Oryza sativa L.)? Environ Technol Inno. 2021;23:101660. doi: 10.1016/j.eti.2021.101660
- Liu HJ, Zhang JL, Zhang FS. Role of iron plaque in Cd uptake by and translocation within rice (Oryza sativa L.) seedlings grown in solution culture. Environ Exp Bot. 2007;59(3):314–320. doi: 10.1016/j.envexpbot.2006.04.001
- Saleem A, Zulfiqar A, Ali B, et al. Iron sulfate (FeSO4) improved physiological attributes and antioxidant capacity by reducing oxidative stress of Oryza sativa L. cultivars in alkaline soil. Sustainability. 2022;14(24):16845. doi: 10.3390/su142416845
- Mei J, Ji K, Su L, et al. Effects of FeSO4 dosage on nitrogen loss and humification during the composting of cow dung and corn straw. Bioresource Technol. 2021;341:125867. doi: 10.1016/j.biortech.2021.125867
- Galdames A, Ruiz-Rubio L, Orueta M, et al. Zero-valent iron nanoparticles for soil and groundwater remediation. Int J Environ Res Public Health. 2020;17(16):5817. doi: 10.3390/ijerph17165817
- Zhang N, Fang Z, Zhang R. Comparison of several amendments for in-site remediating chromium-contaminated farmland soil. Water Air Soil Pollut. 2017;228(10):400. doi: 10.1007/s11270-017-3571-6
- Tang B, Zi Y, e LC, et al. Effects of nano-zero-valent iron and earthworms on soil physicochemical properties and microecology in cadmium-contaminated soils. Water Air Soil Pollut. 2024;235(1):81. doi: 10.1007/s11270-023-06865-w
- Vítková M, Puschenreiter M, Komárek M. Effect of nano zero-valent iron application on As, Cd, Pb, and Zn availability in the rhizosphere of metal(loid) contaminated soils. Chemosphere. 2018;200:217–226. doi: 10.1016/j.chemosphere.2018.02.118
- Gong X, Huang D, Liu Y, et al. Stabilized nanoscale zerovalent iron mediated cadmium accumulation and oxidative damage of Boehmeria nivea (L.) gaudich cultivated in cadmium contaminated sediments. Environ Sci Technol. 2017;51(19):11308–11316. doi: 10.1021/acs.est.7b03164
- Zhou P, Zhang P, He M, et al. Iron-based nanomaterials reduce cadmium toxicity in rice (Oryza sativa L.) by modulating phytohormones, phytochelatin, cadmium transport genes and iron plaque formation. Environ Pollut. 2023;320:121063. doi: 10.1016/j.envpol.2023.121063
- Wang Y, Liu Y, Su G, et al. Transformation and implication of nanoparticulate zero valent iron in soils. J Hazard Mater. 2021;412:125207. doi: 10.1016/j.jhazmat.2021.125207
- Chen Z, T TY, Zhou C, et al. Mechanisms of Fe biofortification and mitigation of Cd accumulation in rice (Oryza sativa L.) grown hydroponically with Fe chelate fertilization. Chemosphere. 2017;175:275–285. doi: 10.1016/j.chemosphere.2017.02.053
- Wang X, Zhang D, Cao Y, et al. Safe utilization effect of passivator on mild to moderate cadmium contaminated farmland (in Chinese). Environ Sci. 2024;45(2):1098–1106. doi: 10.13227/j.hjkx.202303151
- J LH, L ZJ, Christie P, et al. Influence of iron plaque on uptake and accumulation of Cd by rice (Oryza sativa L.) seedlings grown in soil. Sci Total Environ. 2008;394(2–3):361–368. doi: 10.1016/j.scitotenv.2008.02.004
- Li T, Li J, Zhan X, et al. Application of Exogenous Iron Alters the Microbial Community Structure and Reduces the Accumulation of Cadmium and Arsenic in Rice (Oryza sativa L.). Nanomaterials. 2022;12(8):1311. doi: 10.3390/nano12081311
- Ghaley BB. Uptake and utilization of 5-split nitrogen topdressing in an improved and a traditional rice cultivar in the Bhutan highlands. Exp Agr. 2012;48(4):536–550. doi: 10.1017/S0014479712000440
- Jin Z, Luo D, Yu Y, et al. Soil pH changes in a small catchment on the Chinese Loess Plateau after long-term vegetation rehabilitation. Ecol Eng. 2022;175:106503. doi: 10.1016/j.ecoleng.2021.106503
- Tang L, Deng S, Tan D, et al. Heavy metal distribution, translocation, and human health risk assessment in the soil-rice system around Dongting Lake area, China. Environ Sci Pollut R. 2019;26(17):17655–17665. doi: 10.1007/s11356-019-05134-w
- Cuypers A, Plusquin M, Remans T, et al. Cadmium stress: an oxidative challenge. Biometals. 2010;23(5):927–940. doi: 10.1007/s10534-010-9329-x
- Shao G, Chen M, Wang W, et al. Iron nutrition affects cadmium accumulation and toxicity in rice plants. Plant Growth Regul. 2007;53(1):33–42. doi: 10.1007/s10725-007-9201-3
- A KM, James B, H CY, et al. Uptake, translocation, and accumulation of Cd and its interaction with mineral nutrients (Fe, Zn, Ni, Ca, Mg) in upland rice. Chemosphere. 2019;215:916–924. doi: 10.1016/j.chemosphere.2018.10.077
- Clemens S, M AMG, Thomine S, et al. Plant science: the key to preventing slow cadmium poisoning. Trends Plant Sci. 2013;18(2):92–99. doi: 10.1016/j.tplants.2012.08.003
- Meng H, Hua S, H SI, et al. Cadmium-induced stress on the seed germination and seedling growth of Brassica napus L. and its alleviation through exogenous plant growth regulators. Plant Growth Regul. 2009;58(1):47–59. doi: 10.1007/s10725-008-9351-y
- Huybrechts M, Hendrix S, Kyndt T, et al. Short-term effects of cadmium on leaf growth and nutrient transport in rice plants. Plant Sci. 2021;313:111054. doi: 10.1016/j.plantsci.2021.111054
- B SA, M RM, R IM, et al. Response of iron and cadmium on yield and yield components of rice and translocation in grain: health risk estimation. Front Environ Sci. 2021;9:9. doi: 10.3389/fenvs.2021.716770
- Afzal J, H SM, Batool F, et al. Role of ferrous sulfate (FeSO4) in resistance to cadmium stress in two rice (Oryza sativa L.) genotypes. Biomolecules. 2020;10(12):1693. doi: 10.3390/biom10121693
- S DS, Sharma V, K SA, et al. Biofortification of soybean (Glycine max L.) through FeSO4·7H2O to enhance yield, iron nutrition and economic outcomes in sandy loam soils of India. Agriculture. 2022;12(5):586. doi: 10.3390/agriculture12050586
- Torabian S, Zahedi M, Khoshgoftar AH. Effects of foliar spray of nano-particles of FeSO4 on the growth and ion content of sunflower under saline condition. J Plant Nutr. 2017;40(5):615–623. doi: 10.1080/01904167.2016.1240187
- Vansuyt G, Robin A, Briat J-F, et al. Iron acquisition from Fe-pyoverdine by Arabidopsis thaliana. Mol Plant Microbe Interact. 2007;20(4):441–447. doi: 10.1094/mpmi-20-4-0441
- Muneer S, Jeong BR, Decreases S. Fe deficiency responses by improving photosynthesis and maintaining composition of thylakoid multiprotein complex proteins in soybean plants (Glycine max. J Plant Growth Regul. 2015;34(3):485–498. doi: 10.1007/s00344-015-9484-y
- Kroh GE, Pilon M. Regulation of iron homeostasis and use in chloroplasts. Int J Mol Sci. 2020;21(9):3395. doi: 10.3390/ijms21093395
- J GK, H HK, J MLA, et al. Plant hemoglobins: important players at the crossroads between oxygen and nitric oxide. FEBS Lett. 2011;585(24):3843–3849. doi: 10.1016/j.febslet.2011.10.036
- Kobayashi T, Nozoye T, Nishizawa NK. Iron transport and its regulation in plants. Free Radial Bio Med. 2019;133:11–20. doi: 10.1016/j.freeradbiomed.2018.10.439
- Yan Z, Hu Z, Wang S, et al. Effects of lime content on soil acidity, soil nutrients and crop growth in rice-rape rotation system (in Chinese). Sci Agric Sin. 2019;52(23):4285–4295. doi: 10.3864/j.issn.0578-1752.2019.23.009
- Briat JF, Dubos C, Gaymard F. Iron nutrition, biomass production, and plant product quality. Trends Plant Sci. 2015;20(1):33–40. doi: 10.1016/j.tplants.2014.07.005
- Y YH, Wang X, Li F, et al. Arsenic mobility and bioavailability in paddy soil under iron compound amendments at different growth stages of rice. Environ Pollut. 2017;224:136–147. doi: 10.1016/j.envpol.2017.01.072
- Kobayashi T, Nishizawa NK. Iron uptake, translocation, and regulation in higher plants. Annu Rev Plant Biol. 2012;63(1):131–152. doi: 10.1146/annurev-arplant-042811-105522
- M ZD, Y JS, J WY, et al. Assessing the impact of iron-based nanoparticles on pH, dissolved organic carbon, and nutrient availability in soils. Soil Sediment Contam. 2012;21(1):101–114. doi: 10.1080/15320383.2012.636778
- Li XQ, Elliott DW, Zhang WX. Zero-valent iron nanoparticles for abatement of environmental pollutants: materials and engineering aspects. Crit Rev Solid State. 2006;31(4):111–122. doi: 10.1080/10408430601057611
- Xia R, Zhou J, Cui H, et al. Nodes play a major role in cadmium (Cd) storage and redistribution in low-Cd-accumulating rice (Oryza sativa L.) cultivars. Sci Total Environ. 2023;859:160436. doi: 10.1016/j.scitotenv.2022.160436
- Ismail MB, A, Ismail AM. Tolerance of anaerobic conditions caused by flooding during germination and early growth in rice (Oryza sativa L.). Front Plant Sci. 2013;4:4. doi: 10.3389/fpls.2013.00269
- L HX, K FS, Zhu J, et al. Iron supply prevents Cd uptake in Arabidopsis by inhibiting IRT1 expression and favoring competition between Fe and Cd uptake. Plant Soil. 2017;416(1):453–462. doi: 10.1007/s11104-017-3232-y
- Yu E, Wang W, Yamaji N, et al. Duplication of a manganese/cadmium transporter gene reduces cadmium accumulation in rice grain. Nat Food. 2022;3(8):597–607. doi: 10.1038/s43016-022-00569-w
- Zhang X, Zhang X, Zheng Y, et al. Accumulation of S, Fe and Cd in rhizosphere of rice and their uptake in rice with different water managements (in Chinese). Environ Sci. 2013;34(7):2837–2846. doi: 10.13227/j.hjkx.2013.07.015
- Wang X, Deng S, Zhou Y, et al. Application of different foliar iron fertilizers for enhancing the growth and antioxidant capacity of rice and minimizing cadmium accumulation. Environ Sci Pollut R. 2021;28(7):7828–7839. doi: 10.1007/s11356-020-11056-9
- Kong F, Zhou J, X GD, et al. Role of iron manganese plaque in the safe production of rice (Oryza sativa L.) grains: Field evidence at plot and regional scales in cadmium-contaminated paddy soils. Sci Total Environ. 2023;903:166183. doi: 10.1016/j.scitotenv.2023.166183
- D CJ, Huang S, Yamaji N, et al. OsNRAMP1 transporter contributes to cadmium and manganese uptake in rice. Plant Cell Environ. 2020;43(10):2476–2491. doi: 10.1111/pce.13843
- Dong H, Tang S, Ye S, et al. Effect of lime on the transfer of Cd and Pb in the soil-rice cultivation system and their accumulation in the rice grains (in Chinese). J Safe Environ. 2016;16(2):226–231. doi: 10.13637/j.issn.1009-6094.2016.02.044
- Wang P, Zhao F. The transfer and control of cadmium in the soil-rice systems (in Chinese). J Nanjing Agri U. 2022;45(5). doi: 10.7685/jnau.202205004