ABSTRACT
In this study, biochars were prepared by pyrolysis of tea waste and rice husk and hexavalent chromium (Cr(VI) sorption was obtained after post-modification of biochars with magnetic iron nano/micro-particles. The maximum sorption of Cr(VI) by magnetic tea waste biochar (MTWB) was 99.9% followed by 97.36% for magnetic rice husk biochar (MTRHB) at pH 5.2. Among studied competing ions, sulfate ions (SO42-) considerably reduced the sorption of Cr(VI) up to ~66.5% for both biochars. The surface functional groups such as – OH, C-H, Fe-O and porous biochars structure loaded with iron nanoparticles helped in Cr(VI) adsorption by ion exchange and electrostatic interactions as indicated by FTIR, XPS and SEM-EDS data. Both Cr(III) and Cr(VI) species were observed on biochars surface confirming the Cr(VI) removal process by reduction and direct adsorption of Cr(VI) ions. Hence, this study provides an excellent option for the application of magnetic biochars to treat Cr(VI) containing wastewater.
1. Introduction
Heavy metals in surface and groundwater pose serious health and ecological risks because of their toxic, mobile and cancer-causing nature [Citation1–3]. As a result of extensive use in tanning and dye production industries, chromium (Cr) as Cr(VI) is found at elevated levels thus attracted tremendous attention due to its toxicity and detrimental effects in water and other segments of the environment [Citation4]. The US-EPA has categorized Cr as a priority pollutant and International Agency for Research on Cancer designated Cr as a ranked one carcinogen [Citation5,Citation6]. Moreover, intake of Cr-contaminated water in humans can cause cardiovascular diseases, genotoxic, mutagenic and respiratory effects as well as reproductive disorders [Citation7]. Some other non-carcinogenic effects include cramps, stomach and intestinal bleeding, diarrhea, kidney and liver diseases [Citation8]. Considering the extremely toxic nature of Cr(VI), WHO and Pak-EPA has provided the allowable limit of 0.05 mg L−1 in drinking water and 1 mg L−1, in water supplies, respectively [Citation9,Citation10].
The Cr(VI) species (i.e Cr2O72-, HCrO4− and CrO42-) are generally more poisonous, mobile and soluble in aqueous solutions [Citation11,Citation12]. While in contrast, Cr(III) shows about 100 times less solubility, mobility, and toxicity and may easily make precipitate such as Cr(OH)3 in water [Citation13]. Therefore, the efficient techniques with dual function such as direct Cr(VI) adsorption as well as reduction to more stable Cr(III) have gained great interest to decontaminate Cr(VI)-rich aqueous environment [Citation14,Citation15]. It decreases the related health and environmental threats by reducing the total Cr concentration in water.
Although various methods including ion exchange, precipitation and activated carbon adsorption have been developed to eliminate Cr(VI) but its mobility and toxicity still persist [Citation16,Citation17]. Above-mentioned conventional methods are costly, time consuming and generate massive amount of wastes for example sludge, gypsum and metal hydroxides. Moreover, the competition for active sites or steric effect by other ions and organic matter in Cr(VI)-rich water leads to low Cr(VI) removal rate in traditional adsorption process. In recent past, the porous carbonaceous material has been recognized as an excellent contender to treat Cr(VI)-rich solutions [Citation18,Citation19]. Considering this, biochar has emerged as a carbon-based material for successful Cr(VI) removal, because of its low-cost and presence of numerous functional groups on the surface [Citation20,Citation21]. Biochar has been widely applied as a potential biosorbent to eradicate poisonous heavy metal ions (arsenic, lead or cadmium) from contaminated water [Citation22,Citation23]. The heavy metals removal mechanism by biochar usually includes complexation with functional groups, physical sorption, ion exchange and surface precipitation [Citation24]. Thus, the final removal mechanism could be a combination of aforementioned processes and the subsequent features of heavy metals sorption are largely associated with the feedstock of biochar.
Tea waste is a significant waste which can be an excellent feedstock for the production of biochar since massive amount of tea waste is produced worldwide for example nearly 190,400 t/year of tea waste is generated in India [Citation25]. Rice husk can also be used to produce biochar since it is generated in large amount and about 1376.6 thousand metric tons/year of rice husk is produced in Pakistan [Citation26].
Nevertheless, the pristine or raw biochar usually provides less Cr(VI) sorption capacity due to inadequate active binding sites and less reduction reactions by functional groups [Citation27,Citation28]. Thus, to improve the removal capacity of Cr(VI) and reaction time several modification/activation methods have been adopted for example pretreatment with nanomaterials, acid/bases and functional groups [Citation29,Citation30]. Magnetic biochar produced through the addition of iron-based compounds has been employed for the decontamination of Cr(VI)-rich water due to its high sorption potential and relatively easy separation process [Citation31,Citation32]. Interestingly, magnetization of biochars usually reduces the BET surface area because of presence of secondary iron (hydro)oxides after the modification [Citation33]. While in contrast, the availability of these minerals on biochar surface significantly increased the heavy metals (Cd, Pb) sorption capacity by adsorbing them on these precipitated (hydro)oxides [Citation33]. It is relevant to mention that magnetic biochars are ferromagnetic, thus can easily be separated from water after the treatment process using a strong magnet [Citation34,Citation35]. Previously, magnetic biochars have been produced for Cr(VI) removal from different feedstock including carboxymethyl cellulose [Citation36], herb-residue [Citation37], corncobs and peanut hulls [Citation38], oak powder [Citation39], Enteromorpha prolifera [Citation40] and Melia azedarach [Citation41]. However, to our knowledge limited data is reported on microwave-assisted magnetization of biochar. Microwave heating is considered as rapid and selective technique having high energy and uniform heat distribution thus proving to be more beneficial compared to conventional heating methods [Citation42]. The major benefit of microwave heating is the sharp increase in temperature of the major portion of the sample owing to production of intense microplasma spots as a result of microwaves application. These localized spots lead to pores opening of the material thus significantly improving number of active sites for adsorption [Citation43].
Although, post-modification of biochars has been done widely in previous research which experimentally proved enhanced sorption efficiency for heavy metals [Citation44,Citation45]. However, still there is a dire need to explore the Cr(VI) sorption mechanisms after the magnetization of biochar using advanced techniques such as X-ray photoelectron spectroscopy (XPS).
The two prepared biochars, tea waste biochar (TWB) and rice husk biochar (RHB) were post-modified by applying microwaves using iron oxide to make magnetically responsive biochars and subjected to sorption experiments. Moreover, the performance of magnetic TWB (MTWB) and magnetic RHB (MRHB) in the presence of co-existing ions were determined which is also a missing link in the previous research. The effect of different environmental conditions such as pH, Cr(VI) concentration and biochar dose was also studied to test the performance of magnetic biochars in varying environmental conditions. The underlying mechanism during Cr(VI) sorption by magnetic biochars was also revealed with the help of spectroscopic analyses such as XPS, SEM-EDS and FTIR.
2. Material and methods
2.1. Materials
Rice husk was obtained from rice mill and tea waste was collected from teashop. These biomaterials were washed with distilled water (DW) followed by sun-drying (2 days) and oven-drying at 65 °C (3 days) in order to remove moisture content completely. The organic biomaterials were ground and sieved carefully. The required Cr(VI) stock solution of 4000 mg L−1 was made by adding a weighed amount of Cr salt (K2Cr2O7) and sorption working solutions were prepared via appropriate dilution of Cr(VI) stock solution. All the chemical including K2Cr2O7, FeSO4·7 H2O and sodium hydroxide were purchased from Sigma-Aldrich.
2.2. Preparation of magnetically responsive biochar
The washed biomaterials were pyrolyzed for 2 h at 450 °C to make biochar following the methodology of [Citation46]. In brief, tea waste and rice husk were obtained and air- dried for 48 hours. The air-dried material was pyrolyzed using typically designed lab-scale stainless-steel 10 kg capacity furnace equipped with a gas burner. The natural gas from outside was used for the ignition and temperature was monitored using a top mounted thermo-gauge. The fire ignited was not in direct contact with the feedstock and pyrolysis temperature and time were carefully noted during biochar preparation. Following pyrolysis, the newly produced biochars were left in furnace for a few minutes for cooling and then removed, ground, and sieved (2 mm size).
The method of [Citation45] was followed to make the magnetic biochar. In brief, 100 mL DW was added in 800 mL beaker and 1 g of FeSO4·7 H2O was put in the same beaker. During the mixing of FeSO4·7 H2O and DW, the pH value of the solution was increased till 12.0 by adding 1 M potassium hydroxide or sodium hydroxide solution. Iron hydroxide precipitates were formed during this process. By adding more DW, the suspension was diluted to 200 mL volume and microwaved (700 W, 2450 MHz) in a regular kitchen microwave oven for 10 minutes with maximum power. After that, the magnetic suspension beaker was removed and neutral pH was attained by repeated washing of magnetic iron oxide nano- and micro-particles with DW.
Magnetically responsive biochar was prepared by mixing 2 mL nano- and micro-particles of microwave iron oxide suspension and 1 g of biochar in a small beaker. Microwave iron oxide suspension contained four parts of deionized water and one part of sediment iron oxide particles. Microwaved iron oxide particles were vigorously mixed with biochar by using laboratory spoon or spatula for homogeneous distribution. The mixture was dried for 48 hours at 60 °C. To obtain the small particles, the dried material was reground.
2.3. Experimental setup
Generally, 25 mL of 120 mg L−1 Cr(VI) working solution and 0.015 g (0.6 g L−1) of biochar was mixed in 50 mL plastic tubes with screw caps and agitated for 2 hours. For investigating the effect of initial pH, study was conducted in 120 mg L−1 Cr(VI) working solution and pH was varied from 3 to 10 for 2 h reaction time. The kinetic experiments were carried out at pH 5.2 using a range of time intervals (0.016–24 h) in above-mentioned Cr(VI) working solution. Various Cr(VI) concentrations from 0.1 to 1.3 g L−1 were employed for examining the biochar dosage effect and the mixture was shaken for 2 h to obtain equilibrium. The effects of anions on Cr(VI) adsorption and reduction was also tested with 50 mg L−1 of each NO3–, PO43– and SO42–. The remaining Cr(VI) in samples were estimated using Atomic absorption spectrophotometer (AAS) after filtration.
2.4. Characterization and statistical analysis
The FTIR spectrometer (TENSOR-II, Bruker Ettlingen, Germany) was employed to determine functional groups of MTWB and MRHB. The surface morphological features and bulk composition of MTWB and MRHB were examined using Field Emission Scanning Electron Microscope (FESEM-7600F JEOL) with Energy Dispersive X-ray Spectroscopy (EDS-Oxford Instrument INCA). The features of charge recombination ratios in this experiment were analyzed using photoluminescence (PL) measurements (spectro-fluorophotometer; Shimadzu RF-5301PC) with 325 nm excitation wavelength. The shift of surface elemental state was estimated using XPS (PHI 5000 Versa Probe II). Microsoft® Excel 2010 and Sigma Plot version 10 were used for statistical analyses.
2.5. Equilibrium modeling
Various isotherm and kinetic models were used to understand Cr sorption mechanism by biochars [Citation47].
3. Results and discussion
3.1. Aqueous pH
The aqueous pH during sorption process plays critical role by influencing the surface chemistry of biosorbent and elemental speciation [Citation48]. The Cr(VI) sorption at different values of pH was observed with MTWB and MRHB. The sorption was noted to be pH dependent as the results show the major role of pH in Cr(VI) removal from aqueous medium . Increasing trend of Cr(VI) sorption with MTWB and MRHB was observed because the increasing pH value from 3 to 5.2 facilitated the removal efficiency of Cr(VI) - 93.45 to 99.9% with MTWB while 90.25% to 97.36% with MRHB. The Cr(VI) removal efficiency of MTWB was slightly higher (2–3%) than MRHB as shown in . Percentage of Cr(VI) removal was decreased considerably from 99.9–64.14% and 97.36–60.13% by MTWB an MRHB, respectively, when aqueous pH value was increased from 5.2 to 10. The maximum Cr(VI) removal efficiency (>99%, achieved at 2–5 pH could be explained as follows: Firstly, at pH 2–5, the existing HCrO4− species were very suitable for adsorption by electrostatic interactions owing to their low sorption free energy [Citation49]; secondly, the surface of both biochars was positively charged owing to the modification with iron oxides particles and protonized due to the occurrence of more H+ ions, which are favourable for anionic species sorption through electrostatic interaction [Citation45,Citation50]; thirdly, the presence of iron oxides particles exhibited more reduction performance which is helpful in eliminating Cr(VI) from solution [Citation51,Citation52]. On the other hand, at relatively alkaline pH (6–10), the negative charge (hydroxyl ions) on surface and available hydroxide precipitate might cause low removal efficiency for Cr(VI) [Citation53].
3.2. Contact time
shows the removal efficiency of both biochars (MTWB and MRHB) for Cr(VI) with different contact time. Kinetics of sorption has two stages including slower and rapid stage in order to approach the equilibrium. In this study, significant Cr(VI) removal was achieved at initial stage since MTWB and MRHB removed 96.2 and 94.9% Cr(VI), respectively, in 1st hour of contact time. When contact time reached to 2 hours, 98.2% Cr(VI) was removed with MTWB while 97.1% removal of Cr(VI) was obtained with MRHB from the solution .
No major increase in Cr(VI) removal efficiency was recorded with increasing reaction time (>2 h) since the system reached equilibrium. In aqueous medium huge number of sorption sites are available at initial contact time thus the biosorbents adsorbed maximum Cr(VI) when the contact time was 2 hours [Citation54]. At initial contact time, maximum number of sorption sites was available and ions of Cr(VI) easily diffused along the micropores of biochars. After 2 hours contact time, adsorption sites were believed to be almost fully saturated and restricted the adsorption of remaining ions. Furthermore, competition between the Cr(VI) ions and less number of available active surface sites reduced the rate of sorption with increase in contact time. Comparison with previous studies also showed that sorption rate was higher at initial stages of contact time [Citation17,Citation55].
3.3. Biochar dose
The biochar dosage could influence adsorption cavities, economic cost and reduction process. Due to the shortage of binding sites, initially the Cr(VI) removal efficiency was less, however, it was increased from 74.5 to 86.35% and 72.25 to 85.84% with MTWB and MRHB, respectively, when biosorbent dose was enhanced from 0.1 to 0.6 g L−1 as shown in . Increase in biosorbent dose possibly increases the number of surface and exchangeable sites for Cr(VI) sorption [Citation14,Citation55]. The Cr (VI) sorption efficiency was decreased from 86.35% to 58.1% with MTWB and from 85.84% to 56.28% with MRHB when dosage increased from 0.6 to 1.3 g L−1. High dose of MTWB and MRHB showed less Cr(VI) removal efficiency due to the adsorption sites remaining unsaturated and high amount of biochars which is not suitable for large-scale application. Moreover, at higher dosage, sorption performance of Cr(VI) is reduced owing to sorbent particles overcrowding and gradual overlapping of active sites [Citation56]. Thus, at a definite Cr(VI) initial concentration (120 mg L−1), considering the removal efficiency, 0.6 g L−1 of MTWB and MRHB was optimal and thus applied in this study.
3.4. Competing ions effect
Industrial wastewater contains many anions such as PO4−3, SO4−2 and NO3−. Sorption of heavy metal(loid)s with biosorbents could be disturbed in the presence of these anions. describes the performance MTWB and MRHB for Cr(VI) ions removal in the occurrence of these anions. It was clear from the that the occurrence of PO4−3, SO4−2 and NO3− anions (50 mg L−1) in water severely affected Cr(VI) removal efficiency of MTWB and MRHB. Interestingly, sorption of Cr(VI) with MTWB faced maximum decline with only 67.5% Cr(VI) removal was obtained under SO4−2 ions presence while PO4−3 and NO3− in sorption solution showed relatively less influence with 80 and 77% Cr(VI) removal, respectively, from aqueous solution.
Similarly with MRHB, the removal efficiency of Cr(VI) was only 66.50, 76.50 and 80% when the SO4−2, NO3− and PO4−3 ions, respectively, were present in solution. Overall, the presence of SO4−2 ions in solution showed lowermost removal of Cr(VI) compared to other anions with both biochars (MTWB and MRHB). Our results are in agreement with previous studies which also showed less sorption under SO4−2 ions presence [Citation14,Citation57].
3.5. Isotherm modeling
Langmuir and Freundlich isotherm models were applied and model calculated parameters values are shown in . The R2 values of Langmuir model was higher (0.96 and 0.97) than R2 of Freundlich model (0.89, 0.89) for MTWB and MRHB, respectively (). Thus, it was confirmed that Cr(VI) sorption by MTWB and MRHB was because of monolayer adsorption mechanism.
Table 1. Isotherm modeling parameters for MTWB and MRHB.
3.6. Kinetic modeling
Kinetic modeling data showed that R2 for pseudo-second order (PSO) model (0.99, 0.99) was greater as compared to pseudo-first order (PFO) model (0.94, 0.83) for for MTWB and MRHB, respectively (). Furthermore, qe values of PSO were significantly higher compared to PFO model (). Our results demonstrated the suitability of PSO model thus confirming that chemisorption mechanism was responsible for Cr removal by both biochars.
Table 2. Kinetic modeling parameters for MTWB and MRHB.
3.7. Characterization and Cr(VI) adsorption mechanism
3.7.1. FTIR spectroscopy
In , peaks of –OH group containing lignin, cellulose and pectin were indicated at the values of 3572 cm−1, 3676 cm−1 for Cr(VI) unloaded and 3545 cm−1, 3689 cm−1 for Cr(VI) loaded MTWB [Citation58]. Bands of Cr(VI) unloaded and loaded on MTWB were noted at the peaks 2362 cm−1 and 2367 cm−1, respectively, for stretching of C-H group containing methoxy, methyl and methylene functional groups [Citation58,Citation59]. The peaks at 1339, 1457, 1541, 1748 cm−1 of MTWB before Cr(VI) uptake and 1350, 1489, 1559, 1716 cm−1 after loading of Cr(VI) indicate the involvement of COO- ionic carboxylic groups containing hemicellulose, protein and pectin [Citation60].
The FTIR data showed the involvement of – OH groups containing macromolecules (cellulose, lignin and pectin) indicated with the peaks at 3649, 3566 cm−1 of MRHB before Cr(VI) uptake (). After loading of Cr(VI), the peaks at 3689. 3629 cm−1 were observed for –OH groups confirming their involvement in adsorption () [Citation34]. The peaks at 2358 cm−1 and 2364 cm−1 were assigned to Cr(VI) unloaded and loaded MRHB, respectively, which might be due to the vibration of C-H groups containing methyl, methylene and methoxy functional groups [Citation61]. Carboxylic groups (COO-) were involved with functional groups having hemicellulose, pectin and protein at peaks 1339, 1684, 1716 cm−1 of unloaded Cr (VI) and 1396, 1647, 1748 cm−1 after Cr (VI) loading on MRHB [Citation62]. The bands at 1092 cm−1 and 1093 cm−1 of Cr (VI) unloaded and loaded, respectively, on surface of MRHB might be accredited to the widening of NH2 vibrations which is a group of proteins [Citation59].
Changes in spectral band positions after Cr(VI) loading could be denoted to the important role of surface functional groups. The –OH, C-H and COO− were mainly involved in sorption of Cr(VI) on MTWB and MRHB by ion exchange mechanism as reported before as well [Citation63].
3.7.2. SEM-EDS and PL measurements
The SEM images of MTWB and MRHB are shown in . It was observed that MTWB surface had rough and fluffy surface containing numerous iron particles. The iron nanoparticles loading on tea waste biochar resulted in wrinkled surface and high porosity thus improving the number of micropores on MTWB surface [Citation64]. In addition, the porous surface structure of MTWB facilitated the contact between biochar and Cr(VI) ions in the solution thereby increasing the adsorption of Cr(VI). While in case of MRHB, iron micro and nanoparticles successfully dispersed on the surface and in the pores of biochar thus providing a relatively coarse surface owing to biochar pores covered with the iron particles. In summary, both these biochars exhibited high adsorption capacity due to Cr(VI) attachment with pores or electrostatic reactions with iron particles.
The SEM-EDS spectra of MTWB and MRHB () showed that in addition to Fe, other elements such as C, O, Ca, Mg and K were detected when Fe was dispersed on the surface of both biochars. Moreover, before adsorption process, Cr(VI) ions were not found but after adsorbing Cr(VI) ions, Cr peaks also appeared (). It is worth noting that MTWB showed strong Cr peak than MRHB thus indicating comparatively higher Cr(VI) removal efficiency. In summary, the results provided the evidence that Cr(VI) ions were successfully adsorbed on both biochars.
Figure 4. EDS analyses of unloaded (a) MTWB (c) MRHB and Cr(VI) loaded (b) MTWB (d) MRHB (e) Photoluminescence (PL) measurements of MTWB and MRHB.
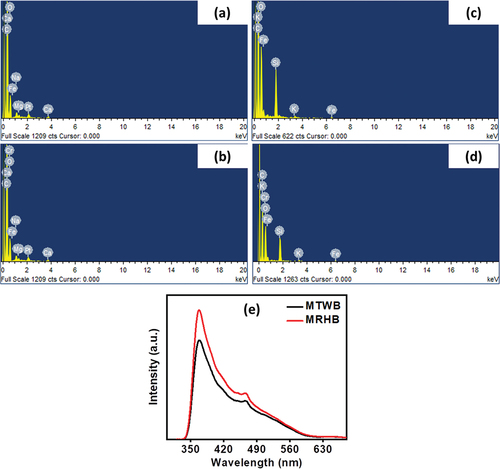
The adsorption ability of MTWB and MRHB were also proved by photoluminescence (PL) measurements in this study. The charge recombination ratios influence the adsorption ability of biochars since the higher intensity charge displays the reduced adsorption performance of a material. Moreover, the intensity of PL is also attributed to shallow and deep defects. The deep defects are related to the enhancement in the charge recombination ratio of the subjected material while shallow defects indicate the reduction in charge recombination ratio also documented by [Citation65]. In this experiment, the PL results show the decline in the PL intensity for MTWB compared to MRHB confirming the improvement in shallow defects which is favourable for high adsorption capacity [Citation65].
3.7.3. XPS analyses
The C1s analysis for MTWB and MRHB is shown in . The peaks were observed around 283.8 eV, 285.6 and 289.0 eV attributed to C-C, C-O and O-C=O, respectively [Citation66–68]. The contribution of C-C for MTWB was 49.65% while for MRHB it was 46.21%. The MTWB has C-O and O-C=O around 44.3 and 6.03% whereas MRHB has around 45.36 and 8.43%.
shows the peaks of O1s observed about 530.2 eV and 532.4 eV, which were assigned as portion of oxide attached with metal most probably Fe, (OMetal) and hydroxide (OH) [Citation67,Citation69].
Chemical stat analysis of Cr2p is illustrated in . In case of MTWB, the Cr 2p3/2 have two peaks around 576.5 and 578.2 eV that are attributed, respectively, to Cr(III) and Cr(VI) [Citation67]. The Cr 2p3/2 consisted of three peaks around 585.12, 586.62 and 588.48 eV that endorsed as Cr(OOH) Cr(III) and Cr(IV) [Citation67]. Similarly for MRHB, the Cr 2p3/2 showed two peaks around 576.39 and 577.51 eV which showed the presence of Cr(III) and Cr(VI), respectively [Citation67]. The Cr 2p3/2 consisted of three peaks at 584.61, 586.93 and 587.76 eV that were ascribed to as Cr(OOH) Cr(III) and Cr(IV) [Citation67]. The results of XPS analyses confirmed the presence of both Cr(III) and Cr(IV) on MTWB and MRHB indicating the Cr(VI) removal process by direct adsorption and/or after reduction to Cr(III).
The Fe2p3 XPA spectra of MTWB and MRHB are shown in . The Fe2p1/2 indicated peaks at 724.42 and 723.11 eV for MTWB and MRHB, respectively, and they were assigned to Fe2O3. Similarly, Fe2p3/2 showed bands at 710.80 eV and 709.91 eV for MTWB and MRHB, respectively, which were due to the presence of Fe3O4 [Citation70]. Thus, presence of iron oxide species such as Fe2O3 and Fe3O4 confirmed that magnetization of biochars was successfully done and iron species on the surface of biochars helped in removal of Cr(VI) from water. The successful removal was also confirmed by presence of Cr species on biochar surface as indicated by XPS spectra ().
3.8. Chromium (VI) removal mechanism
The Cr(VI) removal mechanism could be suggested by three types from above experimental results and analyses: (1) Cr(VI) was exchanged with –OH, C-H and COO- functional groups by ion exchange process, (2) Cr(VI) was sorbed on both biochars by electrostatic interactions with Fe-O species and (3) Cr(III) was reduced to Cr(IV) on MTWB and MRHB.
4. Conclusions
Impregnation with magnetic iron particles enhanced the Cr(VI) sorption from aqueous medium which indicates that magnetization of biochar is more effective for removing Cr ions from contaminated water. Magnetic tea waste biochar (MTWB) and magnetic rice husk biochar (MRHB) were prepared for Cr(VI) adsorption and subjected to various environmental conditions. The MTWB adsorbed maximum Cr(VI) as 99.9% while MRHB showed 97.36% Cr(VI) adsorption from contaminated water at pH value 5.2. The effect of co-occurring anions data indicated that as compared to phosphate and nitrate, the presence of sulfate significantly reduced the sorption rate of Cr(VI) on magnetic biochar. FTIR and XPS results described the major role of –OH, C-H and Fe-O functional groups on biochars surface in Cr(VI) adsorption by ion exchange and electrostatic interactions. SEM-EDS micrographs showed the highly porous structures of biochars with plenty of iron particles, while XPS data confirmed presence of both Cr(VI) and C(III) species on magnetic biochars. Finally, it was concluded that magnetization of biochar was favourable and provided high sorption potential for both biochars.
Acknowledgments
Authors are thankful to the Government College University Faisalabad Pakistan and University of the Punjab, Lahore, Pakistan. The authors express their sincere appreciation to the Researchers Supporting Project Number (RSP2024R48), King Saud University, Riyadh, Saudi Arabia.
Disclosure statement
No potential conflict of interest was reported by the author(s).
Additional information
Funding
References
- Sahu UK, Sahu S, Mahapatra SS, et al. Cigarette soot activated carbon modified with Fe3O4 nanoparticles as an effective adsorbent for as (III) and as (V): material preparation, characterization and adsorption mechanism study. J Mol Liq. 2017;243:395–11. doi: 10.1016/j.molliq.2017.08.055
- Irshad MA, Sattar S, Nawaz R, Al-Hussain SA, Rizwan M, Bukhari A, Waseem M, Irfan A, Inam A and Zaki ME. Enhancing chromium removal and recovery from industrial wastewater using sustainable and efficient nanomaterial: a review. Ecotoxicol Environ Saf. 2023;263:115231. doi: 10.1016/j.ecoenv.2023.115231
- Kurniawan TA, Othman MHD, Adam MR, et al. Chromium removal from aqueous solution using natural clinoptilolite. Water. 2023;15(9):1667. doi: 10.3390/w15091667
- Sahu UK, Chen J, Ma H, et al. As (III) removal from aqueous solutions using simultaneous oxidation and adsorption process by hierarchically magnetic flower-like Fe3O4@ C-dot@ MnO2 nanocomposite. J Environ Health Sci Eng. 2023;21(1):47–61. doi: 10.1007/s40201-022-00834-x
- Guo J, Li Y, Dai R, et al. Rapid reduction of Cr(VI) coupling with efficient removal of total chromium in the coexistence of Zn(0) and silica gel. J Hazard Mater. 2012;243(4):265–271. doi: 10.1016/j.jhazmat.2012.10.028
- IARC. Chromium (VI) compounds. International agency for research on cancer monographs on the evaluation of carcinogenic risks to humans. 2012;100C:147–168.
- Hossain MN, Howladar MF, Khan MI, et al. Appraisal of trace metals toxicity and human health risk using a novel approach in wastewater of four gas fields. Groundwater For Sustain Devel. 2024;25:101080. doi: 10.1016/j.gsd.2024.101080
- Ayele A, Godeto YG, Zhang Y. Bioremediation of chromium by microorganisms and its mechanisms related to functional groups. J Chem. 2021;2021:1–21. doi: 10.1155/2021/7694157
- PAK-EPA. National standards for drinking water quality (NSDWG); Pakistan environmental protection agency (PAK-EPA). Islamabad, Pakistan: Ministry of Environment: Government of Pakistan; 2008.
- WHO. Chromium in drinking-water, draft background document for development of WHO guidelines for Drinking-Water Quality World Health Organization, Geneva, Switzerland. 2019.
- Sricharoenvech P, Siebecker MG, Tappero R, et al. Chromium speciation and mobility in contaminated coastal urban soils affected by water salinity and redox conditions. J Hazard Mater. 2024;462:132661. doi: 10.1016/j.jhazmat.2023.132661
- Zou Y, Wang X, Khan A, et al. Environmental remediation and application of nanoscale zero-valent iron and its composites for the removal of heavy metal ions: a review. Environ Sci Technol. 2016;50(14):7290–7304. doi: 10.1021/acs.est.6b01897
- Wang L, Wang Y, Ma F, et al. Mechanisms and reutilization of modified biochar used for removal of heavy metals from wastewater: a review. Sci Total Environ. 2019;668:1298–1309. doi: 10.1016/j.scitotenv.2019.03.011
- Khalil U, Shakoor MB, Ali S, et al. Selective removal of hexavalent chromium from wastewater by rice husk: kinetic, isotherm and spectroscopic investigation. Water. 2021;13(3):263. doi: 10.3390/w13030263
- Zhang N, Reguyal F, Sarmah AK. Effect of iron nanoparticles on chromium adsorption in aqueous solution using magnetic biochar: a site energy distribution analysis. Environ Pollut. 2024;346:123593. doi: 10.1016/j.envpol.2024.123593
- Liu B, Xin YN, Zou J, et al. Removal of chromium species by adsorption: fundamental principles, newly developed adsorbents and future perspectives. Molecules. 2023;28(2):639. doi: 10.3390/molecules28020639
- Qiao K, Tian W, Bai J, et al. Synthesis of floatable magnetic iron/biochar beads for the removal of chromium from aqueous solutions. Environ Technol Innov. 2020;19:100907. doi: 10.1016/j.eti.2020.100907
- Ameha B, Nadew TT, Tedla TS, et al. The use of banana peel as a low-cost adsorption material for removing hexavalent chromium from tannery wastewater: optimization, kinetic and isotherm study, and regeneration aspects. RSC Adv. 2024;14(6):3675–3690. doi: 10.1039/D3RA07476E
- Chatzimichailidou S, Xanthopoulou M, Tolkou AK, et al. Biochar derived from rice by-products for arsenic and chromium removal by adsorption: a review. J Composites Sci. 2023;7(2):59. doi: 10.3390/jcs7020059
- Sahu UK, Tripathy S, Gouda N, et al. Activated carbon–modified iron oxide nanoparticles for Cr (VI) removal: optimization, kinetics, isotherms, thermodynamics, regeneration, and mechanism study. Water Air Soil Pollut. 2023;234(9):561. doi: 10.1007/s11270-023-06588-y
- Shakoor MB, Ye ZL, Chen S. Engineered biochars for recovering phosphate and ammonium from wastewater: a review. Sci Total Environ. 2021;779:146240. doi: 10.1016/j.scitotenv.2021.146240
- Tian L, Li H, Chang Z, et al. Biochar modification to enhance arsenic removal from water: a review. Environ Geochem Health. 2023;45(6):2763–2778. doi: 10.1007/s10653-022-01462-y
- Wilson K, Iqbal J, Obaid Abdalla Obaid Hableel A, et al. Camel dung-derived biochar for the removal of Copper (II) and Chromium (III) Ions from aqueous solutions: adsorption and kinetics studies. ACS Omega. 2024;9(10):11500–11509.
- Tan X, Liu Y, Zeng G, et al. Application of biochar for the removal of pollutants from aqueous solutions. Chemosphere. 2015;125:70–85. doi: 10.1016/j.chemosphere.2014.12.058
- Wasewar KL, Atif M, Prasad B, et al. Batch adsorption of zinc on tea factory waste. Desalinat. 2009;244(1–3):66–71. doi: 10.1016/j.desal.2008.04.036
- Yaseen M, Abbas F, Shakoor MB, et al. Biomass for renewable energy production in Pakistan: current state and prospects. Arabian J Geosci. 2020;13(2):1–13. doi: 10.1007/s12517-019-5049-x
- Lian G, Wang B, Lee X, et al. Enhanced removal of hexavalent chromium by engineered biochar composite fabricated from phosphogypsum and distillers grains. Sci Total Environ. 2019;697:134119. doi: 10.1016/j.scitotenv.2019.134119
- Zhang K, Yi Y, Fang Z. Remediation of cadmium or arsenic contaminated water and soil by modified biochar: a review. Chemosph. 2023;311:136914. doi: 10.1016/j.chemosphere.2022.136914
- Chen L, Chen Z, Chen D, et al. Removal of hexavalent chromium from contaminated waters by ultrasound-assisted aqueous solution ball milling. J Environ Sci. 2017;52:276–283. doi: 10.1016/j.jes.2016.04.006
- Xu X, Huang H, Zhang Y, et al. Biochar as both electron donor and electron shuttle for the reduction transformation of Cr (VI) during its sorption. Environ Pollut. 2019;244:423–430. doi: 10.1016/j.envpol.2018.10.068
- Lyu H, Tang J, Huang Y, et al. Removal of hexavalent chromium from aqueous solutions by a novel biochar supported nanoscale iron sulfide composite. Chem Eng J. 2017;322:516–524. doi: 10.1016/j.cej.2017.04.058
- Zou H, Zhao J, He F, et al. Ball milling biochar iron oxide composites for the removal of chromium (Cr (VI)) from water: performance and mechanisms. J Hazard Mater. 2021;413:125252. doi: 10.1016/j.jhazmat.2021.125252
- Mohan D, Kumar H, Sarswat A, et al. Cadmium and lead remediation using magnetic oak wood and oak bark fast pyrolysis bio-chars. Chem Eng J. 2014;236:513–528. doi: 10.1016/j.cej.2013.09.057
- Chen B, Chen Z, Lv S. A novel magnetic biochar efficiently sorbs organic pollutants and phosphate. Biores Technol. 2011;102(2):716–723. doi: 10.1016/j.biortech.2010.08.067
- Li R, Wang Z, Zhao X, et al. Magnetic biochar-based manganese oxide composite for enhanced fluoroquinolone antibiotic removal from water. Environ Sci Pollut Res. 2018;25(31):31136–31148. doi: 10.1007/s11356-018-3064-1
- Zhang S, Lyu H, Tang J, et al. A novel biochar supported CMC stabilized nano zero-valent iron composite for hexavalent chromium removal from water. Chemosph. 2019;217:686–694. doi: 10.1016/j.chemosphere.2018.11.040
- Shang J, Pi J, Zong M, et al. Chromium removal using magnetic biochar derived from herb-residue. J Taiwan Inst Chem Eng. 2016;68:289–294. doi: 10.1016/j.jtice.2016.09.012
- Xin O, Yitong H, Xi C, et al. Magnetic biochar combining adsorption and separation recycle for removal of chromium in aqueous solution. Water Sci Technol. 2017;75(5):1177–1184. doi: 10.2166/wst.2016.610
- Shafiee M, Foroutan R, Fouladi K, et al. Application of oak powder/Fe3O4 magnetic composite in toxic metals removal from aqueous solutions. Adv Powder Technol. 2019;30(3):544–554. doi: 10.1016/j.apt.2018.12.006
- Chen Y, Wang B, Xin J, et al. Adsorption behavior and mechanism of Cr (VI) by modified biochar derived from enteromorpha prolifera. Ecotoxicol Environ Saf. 2018;164:440–447. doi: 10.1016/j.ecoenv.2018.08.024
- Zhang X, Lv L, Qin Y, et al. Removal of aqueous Cr (VI) by a magnetic biochar derived from Melia azedarach wood. Biores Technol. 2018;256:1–10. doi: 10.1016/j.biortech.2018.01.145
- Natarajan P, Suriapparao DV, Vinu R. Microwave torrefaction of Prosopis juliflora: experimental and modeling study. Fuel Process Technol. 2018;172:86–96. doi: 10.1016/j.fuproc.2017.12.007
- Govindaraju K, Vinu R, Gautam R, et al. Microwave-assisted torrefaction of biomass kappaphycus alvarezii–based biochar and magnetic biochar for removal of hexavalent chromium [Cr (VI)] from aqueous solution. Biomass Convers Biorefin. 2024;14(3):3643–3653. doi: 10.1007/s13399-022-02512-2
- Cheng S, Meng W, Xing B, et al. Efficient removal of heavy metals from aqueous solutions by Mg/Fe bimetallic oxide-modified biochar: experiments and DFT investigations. J Clean Prod. 2023;403:136821. doi: 10.1016/j.jclepro.2023.136821
- Trakal L, Veselská V, Šafařík I, et al. Lead and cadmium sorption mechanisms on magnetically modified biochars. Bioresour Technol. 2016;203:318–324. doi: 10.1016/j.biortech.2015.12.056
- Qayyum MF, Abid M, Danish S, et al. Effects of various biochars on seed germination and carbon mineralization in an alkaline soil. Pak. J. Agric. Sci. 2015;51:977–982.
- Kokab T, Ashraf HS, Shakoor MB, et al. Effective removal of Cr (VI) from wastewater using biochar derived from walnut shell. Int J Environ Res Public Health. 2021;18(18):9670. doi: 10.3390/ijerph18189670
- Babel S, Kurniawan TA. Cr (VI) removal from synthetic wastewater using coconut shell charcoal and commercial activated carbon modified with oxidizing agents and/or chitosan. Chemosph. 2004;54(7):951–967. doi: 10.1016/j.chemosphere.2003.10.001
- Zhuang L, Li Q, Chen J, et al. Carbothermal preparation of porous carbon-encapsulated iron composite for the removal of trace hexavalent chromium. Chem Eng J. 2014;253(7):24–33. doi: 10.1016/j.cej.2014.05.038
- Mohan D, Rajput S, Singh VK, et al. Modeling and evaluation of chromium remediation from water using low cost bio-char, a green adsorbent. J Hazard Mater. 2011;188(1–3):319–333. doi: 10.1016/j.jhazmat.2011.01.127
- Huang G, Zhang H, Shi JX, et al. Adsorption of chromium (VI) from aqueous solutions using cross-linked magnetic chitosan beads. Ind Eng Chem Res. 2009;48(5):2646–2651. doi: 10.1021/ie800814h
- Yang Z, Shan C, Zhang W, et al. Temporospatial evolution and removal mechanisms of As(V) and Se(VI) in ZVI column with H2O2 as corrosion accelerator. Water Res. 2016;106:461–469. doi: 10.1016/j.watres.2016.10.030
- Calderon B, Fullana A. Heavy metal release due to aging effect during zero valent iron nanoparticles remediation. Water Res. 2015;83:1–9. doi: 10.1016/j.watres.2015.06.004
- Hameed BH, Din AM, Ahmad AL. Adsorption of methylene blue onto bamboo-based activated carbon: kinetics and equilibrium studies. J Hazard Mater. 2007;141(3):819–825. doi: 10.1016/j.jhazmat.2006.07.049
- Zhu S, Huang X, Wang D, et al. Enhanced hexavalent chromium removal performance and stabilization by magnetic iron nanoparticles assisted biochar in aqueous solution: mechanisms and application potential. Chemosph. 2018;207:50–59. doi: 10.1016/j.chemosphere.2018.05.046
- Magioglou E, Frontistis Z, Vakros J, et al. Activation of persulfate by biochars from valorized olive stones for the degradation of sulfamethoxazole. Catalysts. 2019;9(5):419. doi: 10.3390/catal9050419
- Khalil U, Shakoor MB, Ali S, et al. Tea waste as a potential biowaste for removal of hexavalent chromium from wastewater: equilibrium and kinetic studies. Arab J Geosci. 2018;11(19):1–9. doi: 10.1007/s12517-018-3932-5
- Yuan YC, Chen BR, Wang RX. Studies of properties and preparation of chitosan resin crosslinked by formaldehyde and epichlorohydrin. Polym Eng Sci. 2004;20(1):53–57.
- Zhao N, Zhao C, Lv Y, et al. Adsorption and coadsorption mechanisms of Cr (VI) and organic contaminants on H3PO4 treated biochar. Chemosphere. 2017;186:422–429. doi: 10.1016/j.chemosphere.2017.08.016
- Özçimen D, Ersoy-Meriçboyu A. Characterization of biochar and bio-oil samples obtained from carbonization of various biomass materials. Renewable Energy. 2010;35(6):1319–1324. doi: 10.1016/j.renene.2009.11.042
- Reddy DHK, Lee SM. Magnetic biochar composite: facile synthesis, characterization, and application for heavy metal removal. A Physicochem Eng Asp. 2014;454:96–103. doi: 10.1016/j.colsurfa.2014.03.105
- Tuna AÖA, Özdemir E, Şimşek EB, et al. Removal of as (V) from aqueous solution by activated carbon-based hybrid adsorbents: impact of experimental conditions. Chem Eng J. 2013;223:116–128. doi: 10.1016/j.cej.2013.02.096
- Pan J, Jiang J, Xu R. Adsorption of Cr (III) from acidic solutions by crop straw derived biochars. Int J Environ Sci. 2013;25(10):1957–1965. doi: 10.1016/S1001-0742(12)60305-2
- Zhang JY, Zhou H, Gu JF, et al. Effects of nano-Fe3O4-modified biochar on iron plaque formation and Cd accumulation in rice (Oryza sativa L.). Environ Pollut. 2020;260:113970. doi: 10.1016/j.envpol.2020.113970
- Choudhury B, Dey M, Choudhury A. Shallow and deep trap emission and luminescence quenching of TiO2 nanoparticles on Cu doping. Appl Nanosci. 2014;4(4):499–506. doi: 10.1007/s13204-013-0226-9
- Bartis EA, Luan P, Knoll AJ, et al. Polystyrene as a model system to probe the impact of ambient gas chemistry on polymer surface modifications using remote atmospheric pressure plasma under well-controlled conditions. Biointerphases. 2015;10(2):029512. doi: 10.1116/1.4919410
- Moulder JF. Handbook of X-Ray photoelectron spectroscopy. Phys Electron. 1992;1:230–232.
- Wang DH, Hu Y, Zhao JJ, et al. Holey reduced graphene oxide nanosheets for high performance room temperature gas sensing. J Mater Chem. 2014;2(41):17415–17420. doi: 10.1039/C4TA03740E
- Yu B, Wang X, Qian X, et al. Functionalized graphene oxide/phosphoramide oligomer hybrids flame retardant prepared via in situ polymerization for improving the fire safety of polypropylene. RSC Adv. 2014;4(60):31782–31794. doi: 10.1039/C3RA45945D
- Huang P, Ye Z, Xie W, et al. Rapid magnetic removal of aqueous heavy metals and their relevant mechanisms using nanoscale zero valent iron (nZVI) particles. Water Res. 2013;47(12):4050–4058. doi: 10.1016/j.watres.2013.01.054