Abstract
Supercritical fluid extrusion (SCFX) is a recent technological development for production of expanded starch-based foams in which formation of a microcellular structure is achieved by injection of supercritical CO2 into the melt. The high effective diffusivity of CO2 in the porous matrix favors escape of the gas to the environment, reducing the amount available for diffusion into the bubbles, thus posing an important challenge. This study utilized two approaches to address this problem: (a) increasing the nucleation rate and thus the final bubble density in the foam, and (b) reducing the melt temperature. The former was achieved by decreasing the nozzle diameter in order to achieve a higher pressure drop rate as the starch-CO2 melt flows through the nozzle. The second approach was evaluated by introducing a cooling zone prior to the entry of the melt into the nozzle. Bubble density increased more than fourfold when the nozzle radius was decreased from 3.00 to 1.50 mm. A higher bubble density led to a greater barrier or resistance to diffusion of CO2 to the environment, and increased expansion ratio by as much as 160%. Cooling of the melt resulted in a decrease in diffusion coefficient of CO2 in the starch melt, and thus reduced CO2 loss to the environment. The expansion ratio increased by 34% as the melt temperature decreased from 60 to 40°C. The above-mentioned strategies can be useful in controlling and enhancing expansion, which ultimately determines the textural attributes of the expanded food product.
Introduction
Supercritical fluid extrusion (SCFX) is a significant development in extrusion research. This technology has been used for production of highly expanded bio-polymeric foams (mainly starch-based) in which carbon dioxide, above its critical pressure (7.38 MPa) and temperature (31°C), is used as a blowing agent, a nutrient carrier, and, if required, an in-line process modifier.Citation1–4 As the use of supercritical carbon dioxide (SC-CO2) obviates the need for steam for expansion, reduced shear, and low product temperatures (<100°C) are attainable, which offer several advantages over the conventional method of steam puffing including reduced product degradation, decreased machine wear, and the potential for adding heat-sensitive nutrients, flavors, and colorings in-line.Citation[3] Moreover, in SCFX processing, the subtle phase change of injected CO2 from supercritical to gas is exploited to obtain precisely controlled, homogenous, and closed cell microcellular structures and unique product textures. This represents a quantum leap over puffed food products produced by conventional extrusion where the more explosive phase change from water to steam leads to very coarse and open cell structures.Citation[3]
The SCFX process for starch-based foams consists of the following major steps: (1) development of a melt with gas holding rheological properties by gelatinization and mixing of the feed in the extruder barrel, (2) injection of SC-CO2 loaded with solutes, if desired, into the melt and mixing in the extruder barrel to create a melt-CO2 solution, (3) pressure drop in the extruder nozzle leading to a thermodynamic instability and consequent nucleation of bubbles, and (4) expansion caused by diffusion of CO2 into the nucleated bubbles as the extrudate proceeds through the nozzle and immediately after its exit from the nozzle. In the final stage, after the nascent foam exits the nozzle, further diffusion of CO2 into the bubbles and their consequent expansion cause the bubble walls to decrease progressively in thickness, and increase the rate at which CO2 diffuses out of the foam to the environment. This causes the amount of CO2 available for bubble growth to decrease and could even lead to foam collapse as observed in SCFX processing,Citation[3] which also has been reported for polymeric foams.Citation[5] By controlling the effective diffusivity of CO2 through the starch-based foam, potential exists for controlling the cellular structure and increasing product expansion, thus manipulating the mechanical and textural properties of the end product. The main focus of this study was to test this hypothesis.
Effective diffusivity of gas (D eff) in a porous material with predominantly closed-cell structure, as in SCFX extrudates, can be modeled as the sum of two pathways: (a) diffusion through the solid or semi-solid bio-polymer surrounding the gas cells, and (b) diffusion through the gas cells and their walls. An expression for D eff in the porous extrudate can be derived from the approach proposed by Holl et al.,Citation[6] as described below:
Table 1 Nozzle geometry, calculated pressure drop rate, experimentally determined bubble density, and expansion ratio for SCFX extrudates
The objective of this study was to utilize two approaches to control D eff of CO2 in the starch-based foam: (a) increase the nucleation rate and thus the final bubble density in the foam (n in Eq. (Equation4)), which would provide a greater barrier or resistance to diffusion, and (b) decrease the diffusion coefficient in the starch melt (D in Eq. (Equation4)) by reducing the product temperature. The former was achieved by varying the nozzle dimensions in order to achieve a higher pressure drop rate as the starch-CO2 melt flowed through the nozzle, as has been shown in studies involving production of polymeric foams.Citation[5] Citation[7] Product temperature was reduced by introducing a cooling zone prior to the entry of the melt into the nozzle. The experimental design for evaluating the above two strategies for enhancing foam expansion is described in the next section.
Materials and Methods
Formulation
The base formulation used in all extrusion runs was established in earlier studies,Citation[3] and consists of pregelatinized corn starch (49.5 kg/100 kg dry mix) (Cerestar, Hammond, IN), pregelatinized potato starch (24 kg/100 kg dry mix) (Residol, Decatur, IL), pure cane confectioners sugar (24 kg/100 kg dry mix) (Tate and Lyle North American Sugars, Baltimore, MD), salt (1 kg/100 kg dry mix) (Drescher, Liverpool, NY), and distilled monoglyceride (1.5 kg/100 kg dry mix) (Danisco, New Century, KS). In order to improve the structural stability of the extrudates, whey protein concentrate (WPC-34) (Mid-American Dairymen, Springfield, MO) was added at a concentration of 7 kg/100 kg base mix. In-barrel moisture content of the starch melt was maintained at 35% (w.b.) by injecting water in the extruder barrel.
Extruder Details and Operating Conditions
A co-rotating twin screw Wenger TX-57 Magnum extruder (Wenger Manufacturing, Sabetha, KS) with 9 heads, barrel diameter of 52 mm, and L/D ratio of 28.5 was specially modified for supercritical fluid extrusionCitation[3] and operated at a screw speed of 100 rpm and a dry feed rate of 35 kg/h (9.72 × 10−3 kg/s). Typical SCFX screw configuration and barrel pressure profile are described in detail by Alavi et al.Citation[3] The pressure at the end of the extruder barrel (prior to the nozzle) was built up to 10 MPa. Extruder barrel temperature was maintained at around 40°C by circulating chilled brine (−10°C) through barrel jackets. A pilot-scale supercritical fluid injection system was used for injecting SC-CO2 into the starch melt through four valves located around the extruder barrel at a short distance from the nozzle (head 8). A constant SC-CO2 flow rate of 0.28 kg/h (7.8 × 10−5 kg/s) was maintained to achieve a concentration of 0.008 kg CO2/ kg dry feed. The specific mechanical energy (SME) input to the extruder was in the range of 480–550 kJ/kg.
Product temperature for all experiments was maintained at 60°C by adjusting the extruder parameters. For cooling experiments, a slit-die with a jacket for circulating chilled brine (at 10°C) was used after the barrel and prior to the nozzle in order to obtain a lower product temperature of 40°C.
Nozzle Dimensions
Figure shows the geometry of nozzles used in this study. For the nozzle experiments, nozzle land length (L) was maintained constant at 18 mm, while the nozzle radius (R) was 1.50, 2.25, or 3.00 mm. Only one nozzle (R = 2.25 mm, L = 18 mm) was used for the cooling experiments.
Sample Collection and Evaluation
Cylindrical extrudates emerging from the die were collected on metal trays and cut to a length of approximately 1 m. Trays containing the extrudates were dried in an oven at a temperature of 70°C for nozzle experiments and at 85°C for cooling experiments and then placed at room temperature for 24 h. Final moisture content of extrudates was 5% w.b. as determined by the oven drying method.Citation[8]
Expansion ratio (ER) was calculated by dividing the cross-sectional area of the extrudate by that of the nozzle. Piece density (ρ) was calculated as the ratio of the mass of a sample to that of its total volume including the voids. For this purpose, mass of 5 mm long sample pieces were measured and their volume obtained from the average diameter and length. For each batch, average ER and ρ were measured from at least five samples.
A scanning electron microscope (Leica 440 SEM) was used for examining the cellular structure of extrudates and measuring the average cell size. Samples were cut into 5 mm thick slices perpendicular to the longitudinal axis, mounted on aluminum stubs with double side conductive carbon tape, and sputter-coated with gold before being placed in the SEM for imaging. An image processing software (Image-Pro Plus™) was used to measure the average bubble diameters (D bubble) from the SEM images. The bubble density for each sample was calculated using the following equation as described by Shimbo et al.,Citation[9]
Results and Discussion
Nozzle Experiments
At a constant overall pressure drop (10 MPa) and SC-CO2 concentration (0.8 kg/100 kg dry mix), the nucleation rate, and hence the final bubble density in extrudate, is a function of the pressure drop rate (ΔP/Δt) in the nozzle, and a higher ΔP/Δt would lead to an increase in N bubble. Citation[5] Citation[7] For a non-Newtonian power-law fluid (usually the case for starch melts), ΔP/Δt in the nozzle can be calculated using the method described by Park et al.Citation[7] The pressure drop ΔP across a length ΔL of the nozzle is given by the following equation.
The average residence time in the nozzle Δt is given by Eq. (Equation7), where v avg is the average velocity of the melt in the nozzle.
Therefore, the pressure drop rate in the nozzle ΔP/Δt at a constant flow rate is a function of nozzle radius alone. The following equation gives ΔP/Δt.
In the above equation, C 1 is constant for a constant flow rate (Q).
Based on the equation proposed by Parker et al.Citation[10] for corn starch doughs, K was calculated to be 6.24 kPa s n and n was 0.40. A total flow rate (Q) of 1.102 × 10−5 m3/s was calculated based on the dry feed rate, rate of water injection in the barrel, and assumed melt density of 1.30 × 103 kg/m3 from a correlation given by Schwartzberg et al.Citation[11] Using Eq. (Equation8), ΔP vs. t was calculated and plotted for the three nozzles used in this study (Fig. ). According to the solubility data determined by our research group,Citation[12] CO2 dissolved in the starch melt (at a concentration of 0.008 kg CO2/ kg of dry starch) becomes supersaturated and comes out of solution at a pressure of 2000 kPa or below. Therefore, it follows from Fig. that nucleation started in the straight section of the nozzle for all three radii. As nozzle radius R decreased from 3.00 to 1.50 mm, calculated ΔP/Δt in the straight nozzle section increased from 22.5 to 413 MPa/s, and, thus, the bubble density was also expected to increase.
Figure 2 Theoretical pressure profiles in extruder nozzle for three different nozzle radii, R—(i) 3.00 mm, (ii) 2.25 mm, and (iii) 1.50 mm. In the flow restrictor valve and spacer element prior to the nozzle, P drops from 10 MPa to a value determined by R. ΔP/Δt refers to the calculated pressure drop rates in the straight section of the nozzles. The inset shows the nozzle profile with tapered (AB) and straight (BC) sections.
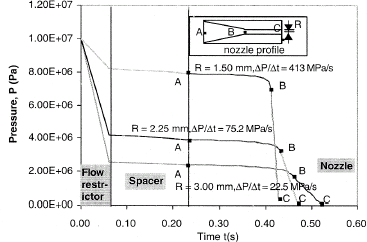
Experimental results confirmed the above reasoning. The average bubble diameter (D bubble) and bubble density (N bubble) of extrudates produced by nozzle radii in the range of 1.50–3.00 mm are plotted in Fig. . As the nozzle diameter decreased from 3.00 to 1.50 mm, N bubble increased more than fourfold, from 0.27 × 106/cm3 to 1.14 × 106/cm3. On the other hand, D bubble decreased by 20% from 266 to 209 microns for the same range of nozzle diameters. Similar results have been reported for high impact polystyrene (HIPS) foams produced by injection of SC-CO2,Citation[7] where N bubble increased by more than 50 times as nozzle radius decreased from 0.60 to 0.23 mm, although corresponding changes in D bubble or the overall expansion of the foams was not reported.
Figure 3 Average bubble diameter (D bubble) and nucleation density (N bubble) vs. nozzle radius for SCFX extrudates.
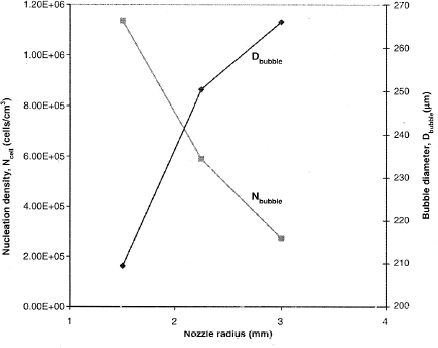
The expansion ratio (ER) and piece density (ρ) of SCFX samples vs. the nozzle radius are plotted in Fig. . It is apparent that samples expanded more as the nozzle radius decreased. Piece density ρ decreased by 40% as nozzle radius decreased from 3.00 to 1.50 mm, while ER increased by 160% for the same range of nozzle radii. As extrudate N bubble increased, the effective diffusivity (D eff) of CO2 reduced and less gas escaped out, leaving a larger amount for diffusion into the bubbles. This led to enhanced expansion, although the average bubble diameter was reduced because the CO2 was distributed over a greater number of bubbles. These results confirm the hypothesis that as N bubble increases, effective diffusivity D eff of CO2 in the foam would decrease, as predicted by Eq. (Equation4). Although with increasing N bubble, the cell wall thickness W would also decrease, but the overall effect is that of reduction in D eff. Effect of nozzle dimensions on expansion of steam-puffed corn starch has been studied in the past.Citation[13] It was reported that as nozzle L/D ratio increased, the expansion ratio increased and reached a maximum, and, thereafter, decreased. However, the initial increase in expansion had little to do with the bubble nucleation rate and was attributed to an increase in gas holding capacity of the starch melt and enhanced degree of gelatinization caused by higher barrel pressures, which increased the residence time and shear rate experienced by the corn starch melt.
Cooling Experiments
The melt temperature for the control run was 60°C, which was reduced to 40°C by circulation of chilled brine (10°C) through the slit-die jacket prior to the extruder nozzle. Figure shows the microcellular structure of control extrudates (with no cooling) and those produced by cooling the melt. Figure shows the average bubble diameter, expansion ratio, and piece density of these samples. As the melt temperature decreased from 60 to 40°C, average bubble diameter increased more than 10% from 385 to 430 microns and expansion ratio increased 34% from 8.0 to 10.8. The piece density of samples decreased 20%, from 0.20 to 0.16 g/cm3.
Figure 5 Scanning electron micrographs of SCFX extrudates showing the cellular structure obtained at two melt temperatures: (a) 60°C and (b) 40°C. Lowering the melt temperature led to an increase in average bubble diameter and greater overall expansion.
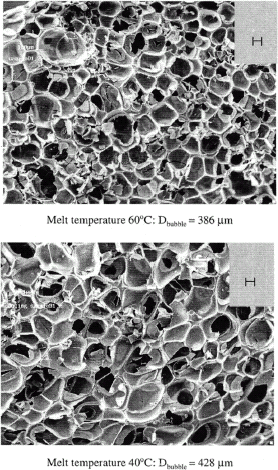
Figure 6 Effect of melt temperature (40 and 60°C) on (a) average bubble diameter, (b) expansion ratio, and (c) piece density of SCFX extrudates.
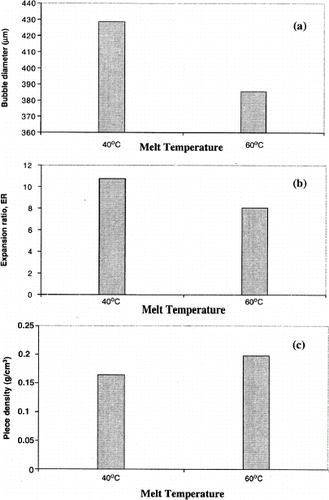
Diffusivity (D) of CO2 in starch melts in the range of SCFX processing moisture is of the order of 10−10 m2/s,Citation[12] Citation[14] but it varies with temperature. De Cindio and CorreraCitation[14] described a correlation for diffusivity of CO2 in starch as a function of temperature (T in K) and moisture (X w in fractional dry basis) (Eq. (Equation10)).
Using the above relation, a decrease in D by 6% was predicted when temperature reduced from 60 to 40°C. Thus, it was safe to conclude that a reduction in melt temperature by 20°C decreased the effective diffusivity of CO2 through the porous starch foam, lowered the amount of CO2 escaping to the environment, and was at least partially responsible for the enhanced foam expansion observed experimentally. Another factor, which could have contributed to the increase in expansion, is the increase in yield stress of the starch melt, which would prevent the collapse of extrudates on exit from the extruder nozzle.Citation[15]
Only two temperatures were evaluated for the cooling study; however, it is expected that if melt temperature is further decreased, there would be an optimum temperature below which expansion would actually decrease, because lowering the melt temperature leads to increase in melt viscosity which would tend to resist expansion.Citation[5]
Conclusions
In SCFX processing, the high effective diffusivity of CO2 in the porous matrix favors escape of the gas to the environment, reducing the amount available for diffusion into the bubbles, thus posing an important challenge. Control of the effective diffusivity of CO2 through the expanded starch-based foam provides an important tool for controlling and increasing the product expansion. This study utilized two approaches to address this problem—(a) increasing the nucleation rate and, thus, the final bubble density in the foam, and (b) reducing the melt temperature. The former was achieved by decreasing the nozzle diameter in order to achieve a higher pressure drop rate as the starch-CO2 melt flows through the nozzle. Bubble density increased more than fourfold when the nozzle radius was decreased from 3.00 to 1.50 mm. A higher bubble density led to a greater barrier or resistance to diffusion of CO2 to the environment and increased expansion ratio by as much as 160%. The second approach of reducing the melt temperature was also evaluated successfully by introducing a cooling zone prior to the entry of the melt into the nozzle. Cooling of the melt from 60 to 40°C resulted in a decrease in the diffusion coefficient of CO2 in the starch melt by 6% (as predicted by a model equation), and, thus, led to a reduction in CO2 loss to the environment. The expansion ratio increased by 34% and piece density decreased by 20% as melt temperature decreased from 60 to 40°C. Therefore, it was concluded that decrease in effective diffusivity of CO2 due to cooling of the melt was at least partially responsible for the enhanced expansion. The above-mentioned strategies can be useful in controlling and enhancing expansion, which ultimately determines the textural attributes of the expanded food product.
Acknowledgments
The authors would like to thank Mr. Esref Dogan for his assistance in extrusion runs and Wenger Manufacturing, Inc. for their contribution through the Cornell-Wenger Extrusion Program.
References
- Rizvi , S.S.H. and Mulvaney , S.J. Extrusion Processing with Supercritical Fluids . US Patent 5,120,559 . 1992 .
- Mulvaney , S.J. and Rizvi , S.S.H. 1993 . Extrusion processing with supercritical fluids . Food Technology , 47 : 74 – 82 .
- Alavi , S.H. , Gogoi , B.K. , Khan , M. , Bowman , B.J. and Rizvi , S.S.H. 1999 . Structural properties of protein-stabilized starch-based supercritical fluid extrudates . Food Research International , 32 : 107 – 118 .
- Gogoi , B.K. , Alavi , S.H. , Khan , M. , Bowman , B.J. and Rizvi , S.S.H. 2000 . Mechanical properties of protein-stabilized starch-based supercritical fluid extrudates . I. J. F. P. , 3 ( 1 ) : 37 – 58 .
- Park , C.B. 2000 . “ Continuous production of high-density and low-density microcellular plastics in extrusion ” . In Foam Extrusion. Principles and Practice Edited by: Lee , S.-T. Lancaster, PA : Technomic Publishing Company, Inc. .
- Holl , M.R. , Garbini , J.L. , Murray , W.R. and Kumar , V. 1994 . “ Dynamic modeling of the solid state microcellular foam process: part 1—system analysis and model development ” . In Cellular and Microcellular Materials Edited by: Kumar , V. and Seeler , K.A. New York : The American Society of Mechanical Engineers .
- Park , C.B. , Baldwin , D.F. and Suh , N.P. 1995 . Effect of pressure drop rate on cell nucleation in continuous processing of microcellular polymers . Polymer Engineering and Science , 35 ( 5 ) : 432 – 440 .
- AOACI. 1995 . Official Methods of Analysis, , 6th Ed. Arlington, VA : Association of Official Analytical Chemists International .
- Shimbo , M. , Baldwin , D.F. and Suh , N.P. 1995 . The viscoelastic behavior of micro-cellular plastics with varying cell size . Polymer Engineering and Science , 35 ( 17 ) : 1387 – 1393 .
- Parker , R. , Ollett , A.-L. , Lai-Fook , R.A. and Smith , A.C. 1990 . “ The rheology of food “melts” and its application to extrusion processing ” . In Rheology of Food, Pharmaceutical and Biological Materials with General Rheolog Edited by: Carter , R.E. Essex, , England : Elsevier Applied Science .
- Schwartzberg , H.G. , Wu , J.P.C. , Nussinovitch , A. and Mugerwa , J. 1995 . Modelling deformation and flow during vapor-induced puffing . Journal of Food Engineering , 25 : 329 – 372 .
- Singh , B. , Rizvi , S.S.H. and Harriott , P. 1996 . Measurement of diffusivity and solubility of carbon dioxide in gelatinized starch at elevated pressures . Industrial Engineering and Chemistry Research , 35 : 4457 – 4463 .
- Chinnaswamy , R. and Hanna , M.A. 1987 . Nozzle dimension effects on the expansion of extrusion cooked corn starch . Journal of Food Science , 52 ( 6 ) : 1746 – 1747 .
- De Cindio , B. and Correra , S. 1995 . Mathematical modeling of leavened cereal goods . Journal of Food Engineering , 24 : 379 – 403 .
- Alavi , S.H. , Rizvi , S.S.H. and Harriott , P. 2003 . Process dynamics of starch-based microcellular foams produced by supercritical fluid extrusion. II: numerical simulation and experimental evaluation . Food Research International , 36 : 321 – 330 .