Abstract
Context: Delivery of drugs and dyes through intact blood–brain barrier (BBB) is extremely sought-after. A safe and reliable measurement of delivery efficacy in live animals is necessary.
Objective: To investigate the brain uptake of FITC-dextran MW 4000 (FD4) by CD71/OX-26 coated nanoparticles by microdialysis sampling and fluorescence/confocal microscopy.
Materials and methods: Methoxy-poly(ethylene glycol)-poly(lactide) (Met-PEG-PLA) and maleimide-poly(ethylene glycol)-poly(lactide) (Mal-PEG-PLA) nanoparticles were prepared by nanoprecipitation. The surfaces of the prepared nanoparticles were embellished with CD-71/OX-26 antibodies for brain targeting. Male Sprague Dawley rats received 0.4 mg/kg FD4 and equivalent nanoparticulate formulation through lateral tail vein. Animals were euthanized 24 h postadministration, after which the tissues were harvested and analyzed for FD4 concentrations. Tissues were fixed with paraformaldehyde, cryotomed to 20 µm sections, and analyzed by Total Internal Reflection microscopy.
Results: Particle sizes of 200 ± 25 nm and zeta potentials of −18 ± 1 mV were obtained. FD4 concentrations, determined using in vivo brain microdialysis, were high on the first day (~360 ng/mL) compared to 60 ng/mL on the following 2 days. The nanoparticle treated animals showed significantly higher (p < 0.05) FD4 concentrations in the brain than pure-FD4 treated animals. Immunopegylated nanoparticles sustained and enhanced Central nervous system (CNS) concentration of hydrophilic dye for at least 3 days.
Conclusion: Immunopegylated nanoparticles produce enhanced and sustained uptake of brain permeability marker FD4 relative to controls.
Introduction
This paper is the first to use in vivo brain microdialysis to detect the uptake of immunopegylated nanoparticles encapsulated with a hydrophilic dye across the blood brain–barrier (BBB). Colloidal drug delivery systems have for long been embellished with different surface-active agents, ligands and antibodies to enhance both the permeation of xenobiotics through the BBB. The BBB, composed of tight junctions of endothelial cells associated with astrocyte foot processes, prevents the travel of large hydrophilic molecules across it (CitationBallabh et al., 2004; CitationAbbott, 2005), making it a bottleneck in Central nervous system (CNS) drug development (CitationPardridge, 2005).
Nanoparticles coated with surface-active agents follow a receptor-mediated endocytotic pathway across endothelial cells (CitationGelperina et al., 2010). Many ligands specific to the overexpressed receptors on the luminal side of endothelial cells have been targeted to promote penetration into the brain parenchyma (CitationGarcia-Garcia et al., 2005).
The systemic administration of drug-loaded nanoparticles is limited by the reticuloendothelial system (RES). Polyethylene glycol (PEG) surface-coatings partially protect the nanoparticles from the RES (CitationBlasi et al., 2007). Pegylated nanoparticles embellished with targeting ligands such as transferrin improve the uptake of nanoparticles across the BBB (CitationUlbrich et al., 2009). Coating nanoparticles with polysorbate and poloxamer decreases their uptake into the liver, where 60–90% of intravenously injected doses end up (CitationStorm et al., 1995). Nanoparticles coated with polysorbate 80 increased the concentration of the poorly water-soluble opiod agonist loperamide in the brain compared to uncoated nanoparticles (CitationAlyautdin et al., 1997). Dalargin-loaded nanoparticles coated with surfactant and apo-E showed an antinociceptive effect superior to that obtained from uncoated nanoparticles (CitationAliautdin et al., 1996). The apolipoprotein pathway (LDL pathway) has been credited with the increased uptake of surfactant-coated nanoparticle (CitationKreuter et al., 1995; CitationKreuter et al., 1997; CitationKreuter, 2001; CitationKreuter, 2005). Pegylated nanoparticles enter rat brain endothelial cells using the LDL pathway (CitationKim et al., 2007). Pegylated immunonanoparticles have been prepared by attaching CD71/OX26 to the Mal-PEG-PLA portion of the polymeric matrix (CitationOlivier et al., 2002). This work involves the evaluation of a brain permeability marker in a similar nanoparticle matrix outfitted with a CD71/OX26 monoclonal antibody.
The uptake of nanoparticles across cell membranes is investigated using different tools such as cell culture, biodistribution studies, transmission electron microscopy (TEM) and fluorescence microscopy (CitationRaut et al., 2010; CitationGiustini et al., 2011; CitationKirthivasan et al., 2012). Preliminary investigations with dye-loaded nanoparticles highlight the uptake mechanisms of colloidal carriers (Citationvan Rooy et al., 2011). Brain-targeting efficacies in live animals are usually ascertained using behavioral studies (CitationKreuter et al., 2002; CitationBlackburn-Munro and Jensen, 2003; CitationDas and Lin, 2005). These approaches provide valuable information, but do not confirm the presence of the payload at the target site. They control for various stimuli and infer that the effect measured is the result of their treatment alone. Hence, these are conclusions of elimination.
Although the literature is replete with studies that measure the localization or even quantification of colloidal nanoparticles in some or all regions of the brain most have a serious limitation i.e. they fail to distinguish actual passage through from localization within the vicinity of the BBB. Therefore, a better solution is sought by using microdialysis for its heretofore-unreported potential to quantify the effectiveness of colloidal nanoparticle formulations of the type described in this report to penetrate the BBB.
In vivo brain microdialysis entails the implantation of a probe in a desired part of the brain. This probe is composed of a membrane with a specific cut-off. A liquid similar in composition to cerebrospinal fluid is perfused through the probe initiating a mass transfer between the perfused liquid and the extracellular fluid surrounding the probe. The concentration of a substance in the dialysate exiting the probe will be a fraction of its existing concentration in the extracellular fluid (CitationBoschi and Scherrmann, 2000).
Choosing a model payload for the measurement of brain-targeting efficacy using microdialysis has two caveats. (i) The payload should not penetrate the BBB naturally and (ii) It should be quantifiable in low concentrations. Fluorescein isothiocyanate dextran 4000 (FD4) is a large molecule of dextran (MW ~4000) attached to a fluorescein isothiocyanate tag, which makes it fluorescent. FD4 was employed because of its poor BBB permeability and ease of fluorescence detection (CitationHoffmann et al., 2011) ().
The in vivo biodistribution of the aforementioned nanoparticles was studied in animals as a proof of concept. Finally, three-day in vivo brain microdialysis was performed to investigate the enhancement of brain uptake of FD4 using pegylated immunonanoparticles. The null hypothesis of this research was that pegylated immunonanoparticles do not produce a higher brain uptake of FD4 than controls. Microdialysis was used to investigate the brain-targeting ability of a nanoparticle formulation.
Methods
Materials
(3S)-Cis-3, 6-Dimethyl-1, 4-dioxane-2, 5-dione (l-lactide), 98%, was purchased from Aldrich chemical company, Inc., USA. PEG 2000 monomethyl ether (Methoxy PEG), O-(2-Maleimidoethyl)-O′-methyl-polyethylene glycol 5000 (maleimide PEG), ethyl acetate 99.5% ACS reagent, stannous octoate, Traut’s reagent (2-Iminothiolane), 5′-dithio-bis (2-nitrobenzoic acid) (DTNB reagent), FD4, acetone, phosphate buffered saline (PBS), dimethyl sulfoxide (DMSO) were purchased from Sigma–Aldrich (St. Louis, MO, USA). Methanol, dichloromethane, ether 98%, toluene 99.8% HPLC grade were purchased from J.T. Baker (Phillipsburg, NJ, USA). Ketamine and xylazine were purchased from Henry Schein, NY. CD 71(OX-26) was purchased from Santa Cruz biotechnology, California. Anti-Goat IgG (whole molecule)-tetramethylrhodamine isothiocyanate (TRITC) and Anti-Mouse IgG (whole molecule)-gold were purchased from Sigma–Aldrich. All microdialysis accessories were purchased from BASi, Lafayette, IN. All organic chemicals and solvents used were of reagent grade. Male Sprague Dawley rats (300–350 g) purchased from Taconic Germantown NY were used for the in vivo experiments.
Preparation of nanoparticles
Copolymers (Methoxy-polyethylene glycol-polylactic acid (Met-PEG-PLA) & maleimide-polyethylene glycol-polylactic acid (Mal-PEG-PLA)) were synthesized in the lab according to CitationParikh et al. (2010). Met-PEG-PLA and Mal-PEG-PLA were accurately weighed in the ratio of 8:1. Nanoparticles were prepared using a modified nanoprecipitation method. The copolymers (450 mg) were dissolved in acetonitrile (60 mL). The solution prepared was taken in Syringe A, which was fixed on an automated syringe drive. Syringe B was attached to a rubber tubing with an inflow of air. In presence of flowing air, the syringe drive was maintained at a flow rate of 2.2 mL/min, which caused droplet formation at the end of a bent needle. The copolymer solution was then sprayed onto the precipitating liquid (deionized water, 200 mL) in a beaker with a distance of 5 cm between the origin of the spray and the liquid layer. A known weight of FD4 (100 mg) was added to water, which was kept under sonication while the copolymer solution was been sprayed. Nanoparticles were formed instantaneously following the diffusion of acetonitrile into water. Acetonitrile was then evaporated under reduced pressure. The suspension obtained was centrifuged at 14 000g for 30 min. The pellet of nanoparticles were washed twice, re-suspended in distilled water and centrifuged again. The collected nanoparticles were frozen and lyophilized to obtain dry nanoparticle powder.
Preparation of immunopegylated nanoparticles
The immunopegylated nanoparticles were prepared using the method by CitationOlivier et al. (2002, Citation2005). OX-26 monoclonal antibodies (200 µg/mL, 100 µL) were thiolated by 2-iminothiolane (Traut’s reagent) (6.9 mg/mL) in PBS (pH = 8) for 60 min and further concentrated by simple dialysis. The excess sulfhydryl groups were analyzed using Ellmann’s reagent. Collected thiolated antibodies were added to wet precipitate of pegylated nanoparticles at a ratio of 1:1 (thiolated OX-26: maleimide). Antibody conjugation was performed for 3 h on a shaker water bath. After 3 h, the nanoparticles were centrifuged and washed twice to remove the unconjugated antibodies. The pellet was obtained, frozen, vacuum dried and further characterized.
Characterization of nanoparticles
Transmission electron microscopy
Morphological evaluation of the pegylated and immunopegylated nanoparticles was performed by TEM. TEM was performed using a Jeol JEM-100CX II electron microscope at 80 kV. The nanoparticles were deposited on a 200 mesh formvar-coated copper grid followed by negative staining with 1 to 2% (w/v) phosphotungstic acid solution, pH 7. The conjugation of OX26 antibodies to the pegylated nanoparticles was visually confirmed by binding with a conjugate of 10-nm gold and an anti-mouse IgG secondary antibody (CitationOlivier, 2005).
Particle size and zeta potential
Particle size and zeta potential measurements were performed by dynamic light scattering (DLS) using Zeta potential/Particle sizer (Nicomp 380 ZLS). For particle size measurement, 1 mg of nanoparticles was dispersed in water using water-bath type sonicator for 5 min, and the size was measured using polystyrene cuvettes. The same suspension was used for zeta potential measurement.
FD4 assay and dye loading
A series of standards (1–1000 ng/mL) were prepared and analyzed with Triad Multimode Detector (Dynex technologies, Belgium) using an excitation/emission of 485/535 nm. The limit of quantification (LOQ) was 1 ng/mL. The FD4-loaded nanoparticles were dissolved in DMSO, diluted, and analyzed by a 96-well plate assay and substituting in the equation.
In vivo studies
Biodistribution studies
The animals received intravenous administration of FD4-loaded formulation (Pegylated and immunopegylated nanoparticles) at 0.4 mg/kg FD4 equivalent and neat FD4 solution as control. The animals were euthanized at the end of 24 h. Brain, liver, kidney, lungs, and spleen were harvested and washed three times with PBS. Tissues were minced, after which the FD4 was extracted with DMSO and further quantified by fluorimetric 96-well plate assay. The FD4 quantified here is a sum of FD4 naturally released from nanoparticles and FD4 released as a result of nanoparticles dissolving in the DMSO used for extraction from tissues.
In vivo brain microdialysis
All animal procedures were performed in accordance with the IACUC Animal guidelines of St. John’s University (Protocol#1659.0). Male Sprague Dawley rats (body weight 300–350 g) were anesthetized (Ketamine 60 mg/kg i.p., and Xylazine 10 mg/kg i.p.) and stereotaxically implanted with a U-shaped microdialysis probe (BAS) in the ventral hippocampus (VHIP). The coordinates for the placement of the tip of the guide cannula were (A/P: 5.91 mm, L: 1.75 mm, D/V: 3.00 mm) for the VHIP with bregma as reference zero and to the brain surface (according to the atlas of CitationPaxinos and Watson, 1986). All experiments were carried out 3 days after the implantation of the guide cannula, on freely moving, conscious rats during the light phase of the day/night cycle. The probe was implanted just before conducting an experiment.
The probe was perfused with artificial cerebrospinal fluid solution (NaCl 140 mM, KCl 3.35 mM, MgCl2 1.15 mM, and CaCl2 1.26 mM, pH 7.4) at a flow rate of 4.0 µL/min using a BAS microperfusion pump. The dialysis sample (120 µL) was collected in a 96-well plate. In vitro recovery was performed at three concentrations (10, 100, and 1000 ng/mL). Retrodialysis was performed at 4 µL/min (each sample was collected for 30 min giving a total sample volume of 120 µL) with a probe recovery of 25.0 ± 0.4% of the concentration in the solution surrounding the probe. After recovery, the animals were used for microdialysis experiments according to the experimental design shown in . The rats received 0.4 mg/kg of FD4 as neat solution or in nanoparticle form by intravenous administration. The study was performed for three days in the light cycle after administration and the samples were analyzed for FD4 by fluorimetry. Probe placement was confirmed by histology and the data from incorrect probe placement were discarded.
Fluorescence and confocal microscopy
Fixed tissues were cryotomed and visualized for FD4 using the fluorescence microscope (NIKON eclipse 80i) with reference to control FD4 treated animals. Immunopegylated nanoparticles were injected through lateral tail vein and the animals were sacrificed at the end of 24 h. Whole brains were harvested, washed and fixed in 4% paraformaldehyde. The fixed tissues were cryotomed to 20 µm thick sections and placed on a glass slide. Anti-Goat IgG (whole molecule)-TRITC was added to the section and was incubated for 2 to 3 h. Excess antibody was washed using PBS and the section was visualized under LSM 510 META (Carl Zeiss, Thornwood, NY, USA).
Results and discussion
Characterization of immunopegylated nanoparticles
Transmission electron microscopy
The nanoparticles prepared were spherical, as shown by TEM pictures (). The pegylated nanoparticles, prepared by nanoprecipitation, were conjugated with OX-26 antibodies to form immunopegylated nanoparticles. The attachment of OX-26 antibody to the maleimide moieties was visualized using a secondary antibody associated with 10 nm gold particles using primary-secondary antibody complexation. The particle size was 140–160 nm.
Particle size and zeta potential
DLS showed the hydrodynamic diameter of the nanoparticles to be 200 ± 25 nm. The particle size from TEM was 20–30 nm less than the hydrodynamic diameter of the nanoparticles. The probable reason could be the swelling of the polymer and free orientations of the maleimide and pegylated fragments on the surface of the nanoparticles. These visual results are in agreement with CitationOlivier et al. (2002), who pioneered the preparation of antibody-modified nanoparticles. The particles had a zeta potential of −18 ± 1 mV.
FD4 loading
The immunopegylated nanoparticles had an FD4-loading of 1% w/w. The hydrophilic nature of the dye as well as the loss of dye during incubation with antibodies can be used to explain the low loading.
In vivo studies
Biodistribution studies
Animals were sacrificed at the end of the 24-h study, after which the tissues were harvested and analyzed. As seen in , both nanoparticle formulations were severed by the RES. The immunopegylated nanoparticles showed a higher distribution in the brain than pegylated nanoparticles (p < 0.05) and neat FD4 solution (p < 0.001). Whereas pegylation offers some stealth to the nanoparticles, and the antibody targets some of the nanoparticles to the brain, neither approach offers ultimate protection from the RES, as seen from the fluorescence in the liver and kidney extract homogenates. Even though this method of targeting does not prevent the clearance of nanoparticles, it results in a more efficient allocation of dose in the target area, which is a requirement of all targeting systems.
Three-day in vivo brain microdialysis
The concentrations of FD4 in the brain obtained for the neat FD4 and pegylated nanoparticles were below the quantification limit of 1 ng/mL, whereas the targeted nanoparticles showed concentrations as high as 360 ng/mL (corrected values based on probe recovery data). Hence, OX-26-labeled nanoparticles target toward the VHIP of the brain. Finite and measurable tissue concentrations of FD4 after administration of neat FD4 and pegylated nanoparticles suggest that these administrations resulted in systemic presence of FD4, but no brain uptake. Immunopegylated nanoparticles reached the target site to show concentrations of 360 ng/mL 1 h after administration and 60 ng/mL 2 days later (). Though the flow rate of 4 µL/min compromised the in vivo recovery of FD4, it facilitated sampling with minimal inductive stress to the operated-on animals. CitationVaka et al. (2009) successfully used in vivo brain microdialysis to quantify nerve growth factor in the VHIP for an intranasal formulation. In our study, in vivo brain microdialysis was successfully integrated with intravenously injected nanoparticulate formulation.
Figure 5. Three-day in vivo brain microdialysis results: concentration of FD4 (corrected for probe recovery) in VHIP of animals receiving antibody-coated pegylated nanoparticles containing FD4. VHIP, ventral hippocampus.
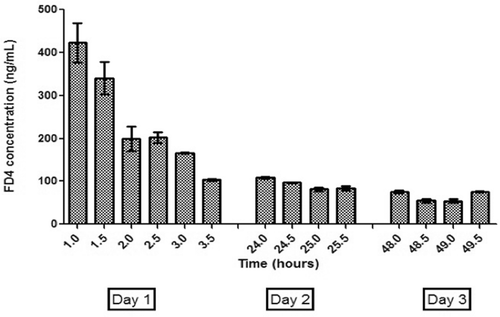
Commendable attempts have been made to evaluate brain targeting using modified nanoparticles (CitationAktas et al., 2005; CitationParikh et al., 2010; CitationKirthivasan et al., 2012), but we believe their methods are found wanting. Most of the current brain-targeting approaches rely on careful studies of the brain tissue post sacrifice of the animals. In other words, the act of observation could taint the results. With in vivo brain microdialysis, however, we are obtaining data from living, freely moving animals, with a probe planted directly in the target tissue. Though such an approach has never been used to evaluate the targeting ability of nanoparticles, we posit that not only is it a viable alternative, it might also lead to new information.
Fluorescence and confocal microscopy
Immunofluorescence confocal imaging () showed the presence of both the green fluorescence associated with FD4 and red fluorescence associated with the conjugated secondary antibody labeled with TRITC. Hence, some of the administered nanoparticle dose has reached the target of action, VHIP and is releasing the FD4. Another reason could be the leakage of the nanoparticles though the blood–CSF barrier (CitationBéduneau et al., 2007). CitationMoos and Morgan (2001) suggest restricted movement of OX-26 beyond the endothelial cells. Immunohistochemical mapping showed little or no OX-26 in the brain parenchyma, leading to the conclusion that the little OX-26 seen in the cerebral parenchyma could be diffusion through blood–CSF barrier (CitationMoos and Morgan, 2001). In our case, immunopegylated nanoparticles showed a high intensity of red fluorescence associated with OX-26 antibodies in the brain parenchyma especially VHIP.
Figure 6. Immunofluorescence confocal imaging of animal tissue after exposure to (A) FD4 saline solution, (B) Peglyated nanoparticles containing FD4, (C) Antibody-coated pegylated nanoparticles containing FD4. Green: FD4, Red: Secondary antibody associated with TRITC.
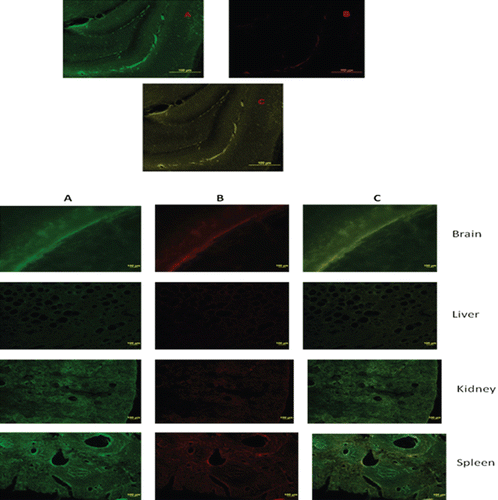
Fluorescence microscopic pictures () of animal brain tissue after administration of FD4-containing immunopegylated nanoparticles showed higher intensity than those obtained in the case of free FD4 and pegylated nanoparticles containing FD4. Absence of fluorescence in the control animals showed that the FD4 does not penetrate the tight junctions of the BBB by itself. Though the fluorimetric results showed some fluorescence in case of pegylated nanoparticles, the microscopic pictures did not reveal any fluorescence in the brain specimens, thereby implying that our quantification limit for fluorescence was lower than that which could be seen by the naked eye. Moreover, this provides visual evidence that the immunopegylated nanoparticles outperform the other two groups in increasing FD4 uptake in the brain. These results concur with CitationAktas et al. (2005) who compared the fluorescence of the brain specimens, treated with the coated and noncoated OX26 nanoparticulate formulations.
Possible mechanism
Receptor-mediated endocytosis could explain the results mentioned in this paper (). Transferrin receptors are overexpressed on the endothelial cells of the BBB, and are relatively abundant in the ventral hippocampal region. OX-26, which is specific to transferrin receptors, could facilitate the uptake of the immunopegylated nanoparticles through that route. The two mechanisms discussed ad infinitum regarding cellular internalization of nanoparticles are receptor-mediated and adsorptive-mediated endocytosis. In case of receptor-mediated endocytosis, either the internalized nanoparticles release their payload in the cytoplasm of the endothelial cells after which the encapsulated dye/drug (now free) diffuses into brain parenchyma, or the nanoparticles themselves are transcytotically released into the brain parenchyma. Hence, the encapsulated dye/drug need not possess the physicochemical properties required to diffuse into the parenchyma on its own. Adsorptive-mediated endocytosis is less specific, and implies the engulfment of nanoparticles by endothelial cell membranes. The surface charge of the nanoparticles plays a major role in this phenomenon (CitationParikh et al., 2010). The surface charge of our immunopegylated nanoparticles was negative and too high in magnitude to be engulfed by the endothelial cell membrane through adsorption. Hence the targeting effect of the antibody was the most likely cause of nanoparticle internalization. The confocal images of the brain sections of animals that received the immunopegylated nanoparticles suggest the same. Though not confirmatory, the results strongly suggest that the OX-26-coated nanoparticles were taken up by the brain. This could be a promising delivery system for CNS actives.
Conclusions
In vivo brain microdialysis was successfully integrated with the evaluation of a CNS-targeted formulation. Fluorescence and confocal images corroborated the microdialysis results. The data do not conflict with existing theories of brain uptake. Preliminary attempts at orthogonal verification of intact, targeted antibody-coated nanoparticle penetration through BBB by TEM continue to be a focus of ongoing studies in this lab. Successful implementation of brain microdialysis in the development of targeted strategies for the brain delivery would help us get closer to narrowing down the mechanism of brain targeting with nanoparticles.
Declaration of interest
The authors report no conflicts of interest.
References
- Abbott NJ. (2005). Physiology of the blood brain barrier and its consequences for drug transport to the brain. Int Congr Ser 1277:3–18.
- Aktas Y, Yemisci M, Andrieux K, Gürsoy RN, Alonso MJ, Fernandez-Megia E, Novoa-Carballal R, Quiñoá E, Riguera R, Sargon MF, Celik HH, Demir AS, Hincal AA, Dalkara T, Capan Y, Couvreur P. (2005). Development and brain delivery of chitosan-PEG nanoparticles functionalized with the monoclonal antibody OX26. Bioconjug Chem 16:1503–1511.
- Aliautdin RN, Petrov VE, Ivanov AA, Kreuter J, Kharkevich DA. (1996). [Transport of the hexapeptide dalargin across the hemato-encephalic barrier into the brain using polymer nanoparticles]. Eksp Klin Farmakol 59:57–60.
- Alyautdin RN, Petrov VE, Langer K, Berthold A, Kharkevich DA, Kreuter J. (1997). Delivery of loperamide across the blood-brain barrier with polysorbate 80-coated polybutylcyanoacrylate nanoparticles. Pharm Res 14:325–328.
- Ballabh P, Braun A, Nedergaard M. (2004). The blood–brain barrier: an overview: Structure, regulation, and clinical implications. Neurobiol Dis 16:1–13.
- Béduneau A, Saulnier P, Hindré F, Clavreul A, Leroux JC, Benoit JP. (2007). Design of targeted lipid nanocapsules by conjugation of whole antibodies and antibody Fab’ fragments. Biomaterials 28:4978–4990.
- Blackburn-Munro G, Jensen BS. (2003). The anticonvulsant retigabine attenuates nociceptive behaviours in rat models of persistent and neuropathic pain. Eur J Pharmacol 460:109–116.
- Blasi P, Giovagnoli S, Schoubben A, Ricci M, Rossi C.. (2007). Solid lipid nanoparticles for targeted brain drug delivery Adv Drug Deliv Rev 59:454–477.
- Boschi G, Scherrmann J.. (2000). Microdialysis in mice for drug delivery research Adv Drug Deliv Rev 45:271–281.
- Das D, Lin S. (2005). Double-coated poly (butylcynanoacrylate) nanoparticulate delivery systems for brain targeting of dalargin via oral administration. J Pharm Sci 94:1343–1353.
- Garcia-Garcia E, Andrieux K, Gil S, Couvreur P. (2005). Colloidal carriers and blood-brain barrier (BBB) translocation: a way to deliver drugs to the brain? Int J Pharm 298:274–292.
- Gelperina S, Maksimenko O, Khalansky A, Vanchugova L, Shipulo E, Abbasova K, Berdiev R, Wohlfart S, Chepurnova N, Kreuter J. (2010). Drug delivery to the brain using surfactant-coated poly(lactide-co-glycolide) nanoparticles: influence of the formulation parameters. Eur J Pharm Biopharm 74:157–163.
- Giustini AJ, Ivkov R, Hoopes PJ. (2011). Magnetic nanoparticle biodistribution following intratumoral administration. Nanotechnology 22:345101.
- Hoffmann A, Bredno J, Wendland M, Derugin N, Ohara P, Wintermark M. (2011). High and Low Molecular Weight Fluorescein Isothiocyanate (FITC)-Dextrans to Assess Blood-Brain Barrier Disruption: Technical Considerations. Transl Stroke Res 2:106–111.
- Kim HR, Gil S, Andrieux K, Nicolas V, Appel M, Chacun H, Desmaële D, Taran F, Georgin D, Couvreur P. (2007). Low-density lipoprotein receptor-mediated endocytosis of PEGylated nanoparticles in rat brain endothelial cells. Cell Mol Life Sci 64:356–364.
- Kirthivasan B, Singh D, Bommana MM, Raut SL, Squillante E, Sadoqi M. (2012). Active brain targeting of a fluorescent P-gp substrate using polymeric magnetic nanocarrier system. Nanotechnology 23:255102.
- Kreuter J. (2001). Nanoparticulate systems for brain delivery of drugs. Adv Drug Deliv Rev 47:65–81.
- Kreuter J. (2005). Application of nanoparticles for the delivery of drugs to the brain. Int Congr Ser 1277:85–94.
- Kreuter J, Alyautdin RN, Kharkevich DA, Ivanov AA. (1995). Passage of peptides through the blood-brain barrier with colloidal polymer particles (nanoparticles). Brain Res 674:171–174.
- Kreuter J, Petrov VE, Kharkevich DA, Alyautdin RN. (1997). Influence of the type of surfactant on the analgesic effects induced by the peptide dalargin after its delivery across the blood brain barrier using surfactant-coated nanoparticles. J Control Release 49:81–87.
- Kreuter J, Shamenkov D, Petrov V, Ramge P, Cychutek K, Koch-Brandt C, Alyautdin R. (2002). Apolipoprotein-mediated transport of nanoparticle-bound drugs across the blood-brain barrier. J Drug Target 10:317–325.
- Moos T, Morgan EH. (2001). Restricted transport of anti-transferrin receptor antibody (OX26) through the blood-brain barrier in the rat. J Neurochem 79:119–129.
- Olivier JC. (2005). Drug transport to brain with targeted nanoparticles. NeuroRx 2:108–119.
- Olivier JC, Huertas R, Lee HJ, Calon F, Pardridge WM. (2002). Synthesis of pegylated immunonanoparticles. Pharm Res 19:1137–1143.
- Pardridge WM. (2005). The blood-brain barrier: bottleneck in brain drug development. NeuroRx 2:3–14.
- Parikh T, Bommana MM, Squillante E 3rd. (2010). Efficacy of surface charge in targeting pegylated nanoparticles of sulpiride to the brain. Eur J Pharm Biopharm 74:442–450.
- Paxinos G, Watson C. (1986). The rat brain in stereotaxic coordinates Sydney; Orlando: Academic Press.
- Raut SL, Kirthivasan B, Bommana MM, Squillante E, Sadoqi M. (2010). The formulation, characterization and in vivo evaluation of a magnetic carrier for brain delivery of NIR dye. Nanotechnology 21:395102.
- Storm G, Belliot SO, Daemen T, Lasic DD. (1995). Surface modification of nanoparticles to oppose uptake by the mononuclear phagocyte system. Adv. Drug Deliv. Rev. 17:31–48.
- Ulbrich K, Hekmatara T, Herbert E, Kreuter J. (2009). Transferrin- and transferrin-receptor-antibody-modified nanoparticles enable drug delivery across the blood-brain barrier (BBB). Eur J Pharm Biopharm 71:251–256.
- Vaka SR, Sammeta SM, Day LB, Murthy SN. (2009). Delivery of nerve growth factor to brain via intranasal administration and enhancement of brain uptake. J Pharm Sci 98:3640–3646.
- van Rooy I, Cakir-Tascioglu S, Hennink WE, Storm G, Schiffelers RM, Mastrobattista E. (2011). In vivo methods to study uptake of nanoparticles into the brain. Pharm Res 28:456–471.