Abstract
A magnesium-dependent cysteinyl-glycine hydrolyzing enzyme from the gastropod mollusk Patella caerulea was purified to electrophoretic homogeneity through a simple and rapid purification protocol. The molecular masses of the native protein and the subunit suggest that the enzyme has a homohexameric structure. Structural data in combination with kinetic parameters determined with Cys-Gly and compared with Leu-Gly as a substrate, indicate that the purified enzyme is a member of the peptidase family M17. The finding that an enzyme of the peptidase family M17 is responsible also in mollusks for the breakdown of Cys-Gly confirms the important role of this peptidase family in the glutathione metabolism.
Introduction
The use of mollusks as sentinels of pollution has been proposed for monitoring the levels of a variety of environmental contaminants in aquatic systems due to their ability to concentrate pollutants present in waterCitation1. The accumulation of pollutants within the body of mollusks often results in impairment in homeostasis and an altered growthCitation2–4. One of the most common features of the accumulation of pollutants within cells and tissues is increased oxidative stressCitation5–8 that can be balanced by a number of antioxidant defenses, including glutathione. Much information is available on glutathione and its metabolism in vertebrates. However, despite the role that glutathione and its metabolism may play in the redox balance of mollusks, little is known about the metabolism of glutathione in this phylum.
Increasing our knowledge on the glutathione metabolism in mollusks can contribute to a better understanding of the balance between stress conditions and defenses in this group of animals. Glutathione is catabolized by the enzyme γ-glutamyl transpeptidase, which transfers the residue of glutamate to α-amino acid producing a γ-glutamyl-amino acid and Cys-GlyCitation9. This dipeptide can promote oxidative conditions, especially in the presence of transition metal ions, such as Fe3+ and Cu2+, eliciting the generation of a reactive species of oxygenCitation10–14. Thus Cys-Gly, which has also been reported as having a signaling functionCitation15–17, can intervene in the processes responsible for the alteration of the redox status of protein cysteine residuesCitation18–21. Due to its prooxidant action, the intracellular levels of Cys-Gly need to be taken under control to prevent cellular damageCitation15–17. Enzyme(s) capable of catabolizing Cys-Gly are therefore relevant factors in the homeostasis of the reduced state of the cell. Cys-Gly hydrolase activity has been associated with the brush border aminopeptidase M (alanyl aminopeptidase, EC 3.4.11.2) or the membrane-bound dipeptidase (EC 3.4.13.19)Citation22,Citation23. However, a growing body of evidence, mainly obtained for rat liverCitation24 and bovine lensCitation25, has recently led to the association of Cys-Gly hydrolase activity in mammals with leucyl aminopeptidase (E.C 3.4.11.1), a metallopeptidase that belongs to the MEROPS peptidase family M17. While enzymes able to hydrolyze Cys-Gly have been characterized in vertebrates and microorganismsCitation26, to our knowledge no information is available on Cys-Gly hydrolase activities in mollusks.
In this paper, we describe a very rapid method for the purification of Cys-Gly hydrolyzing activity from Patella caerulea, and report evidence that the activity may be associated with a M17 family metallopeptidase.
Material and methods
Materials
Cys-Gly, Leu-Gly, 2,2-dihydroxyindane-1,3-dione (ninhydrin), bovine serum albumin (BSA), dithiothreitol (DTT), iodoacetamide (IAA), sequencing-grade trypsin, and molecular mass markers were purchased from Sigma (St. Louis, MO). DEAE-cellulose (DE-52) was obtained from Whatman. Sephacryl S-300 was from Pharmacia Biotech (Uppsala, Sweden). μZip-TipC18 desalting tips were from Millipore (Darmstadt, Germany). All inorganic chemicals were of reagent grade from BDH (Poole, UK). All solvents were HPLC-grade from J.T. Baker Chemicals (Deventer, The Netherlands).
Limpets (P. caerulea) were removed from the rock, immediately frozen on dry ice and transferred at −80 °C until used.
Enzyme assays
Cys-Gly hydrolase activity was assayed as previously describedCitation25, by a colorimetric method based on the reaction between cysteine produced in the hydrolysis of Cys-Gly and ninhydrin. Ninhydrin specifically reacts with cysteine in the presence of a mixture of acetic acid and hydrochloric acid, forming a purple complex with a maximum of absorbance at 560 nm.
The activity of the enzyme towards Leu-Gly was measured by following the decrease in absorbance at 225 nm associated with the hydrolysis of the dipeptide.
One unit of enzyme activity is defined as the amount of enzyme that catalyzes the hydrolysis of 1 μmol of dipeptide per minute under the assay conditions.
Purification of Cys-Gly hydrolase
All purification steps were performed at 4 °C unless otherwise specified.
Preparation of crude extract
Frozen limpets were shelled, weighed, cut into small pieces and homogenized with a hand-driven Potter Elvejhem glass homogenizer in 5 volumes of 50 mM Tris HCl, pH 7.4. The homogenate was centrifuged at 12 000g for 60 min. The supernatant, which is referred to as the “crude extract”, was subjected to the following purification steps.
Ion-exchange chromatography
The crude extract was added to 0.25 volumes of DE-52 previously equilibrated with 10 mM Tris-HCl, pH 7.4 and stirred in an ice-cold bath for 40 min. After stirring, the suspension was centrifuged at 12 000g for 10 min and the supernatant discarded; the precipitated resin was resuspended in 50 mM Tris-HCl pH 7.4, supplemented with 5 mM MgCl2, 2 mM DTT and 0.2 M NaCl. After stirring for 20 min, the suspension was centrifuged at 12 000g for 20 min. The supernatant containing the enzyme activity was withdrawn and subjected to chloroform precipitation.
Chloroform/octanol precipitation
The supernatant from the previous step was added to an equal volume of a mixture of chloroform/octanol (19:1) and the mixture was vigorously shaken on a vortex shaker at room temperature (20 °C) for 2 min. The emulsion was then subjected to centrifugation at 250g for 3 min. The aqueous phase, containing the enzyme activity, was collected by suction. Chloroform/octanol treatment was repeated until clear chloroform/octanol was present at the bottom of the tubes.
Gel filtration
The supernatant resulting from the chloroform/octanol step was concentrated on a vacuum lyophilizer and applied to a Sephacryl S-300 column (1.5 × 90 cm) equilibrated with 50 mM Tris-HCl buffer pH 7.8 supplemented with 2 mM DTT and 5 mM MgCl2. The column flow was 16 ml/h and 2 ml fractions were collected. The active fractions were pooled, analyzed by SDS/PAGE, and stored for subsequent analysis at −80 °C.
Protein identification by mass spectrometry
The SDS-PAGE band of the purified enzyme, appearing at a mass of approximately 54 kDa, was carefully cut from the gel, triturated, washed with water, in-gel reduced with DTT, S-alkylated with IAA, and then in-gel digested with trypsin. The resulting peptide mixture was desalted by μZip-TipC18 using 50% (v/v) acetonitrile, 5% (v/v) formic acid as eluent. Recovered peptides were then analyzed for protein identification by nLC-ESI-LIT-MS/MS, using an LTQ XL mass spectrometer (ThermoFisher, San Jose, CA) equipped with a Proxeon nanospray source connected to an Easy-nanoLC (Proxeon, Denmark). Peptides were separated on an Easy C18 column (100 mm × 0.075 mm, 3 μm) (Proxeon, Denmark). Mobile phases were 0.1% (v/v) formic acid (solvent A) and 0.1% (v/v) formic acid in acetonitrile (solvent B), running at a total flow rate of 300 nL/min. Linear gradient was initiated 20 min after sample loading; solvent B ramped from 5% to 35% over 45 min, from 35% to 60% over 10 min, and from 60% to 95% over 20 min. Spectra were acquired in the range m/z 400 – 2000. Peptide sample was analyzed under collision-induced dissociation (CID)-MS/MS data-dependent product ion scanning procedure, enabling dynamic exclusion (repeat count 1 and exclusion duration 60 s) over the three most abundant ions. Mass isolation window and collision energy were set to m/z 3 and 35%, respectivelyCitation27.
Raw data from nLC-ESI-LIT-MS/MS analysis were searched by MASCOT search engine (version 2.2.06, Matrix Science, London, UK) against an updated (2015/09/12) NCBI non-redundant database (taxonomy Metazoa), in order to identify proteins from the gel slice. Database searching was performed by using Cys carbamidomethylation and Met oxidation as fixed and variable modifications, respectively, a mass tolerance value of 1.8 Da for precursor ion and 0.8 Da for MS/MS fragments, trypsin as proteolytic enzyme, and a missed cleavage maximum value of 2.
Other MASCOT parameters were kept as default. Protein candidates assigned on the basis of at least two sequenced peptides, or one sequenced peptide with at least 2 MS/MS repetitions referring to two different peptide charge states, and with an individual peptide expectation value < 0.05 (corresponding to a confidence level for peptide identification > 95%) were considered confidently identified. Definitive peptide assignment was always associated with manual spectra visualization and verification.
Other methods
SDS-PAGE of the purified enzyme was performed according to LaemmliCitation28, and gels were stained with silver nitrate following WrayCitation29.
ColorPlus™ Prestained Protein Ladder (New England Biolabs, Ipswich, MA) was used for the determination of the apparent molecular weight of the subunit by SDS-PAGE.
The proteins used as molecular weight standards for the determination of the apparent molecular weight of the native enzyme by gel filtration on S-300 were thyroglobulin (MW 669 kDa), apoferritin (MW 443 kDa), β-amylase (MW 200 kDa), alcohol dehydrogenase (MW 150 kDa) and chymotrypsin (MW 25 kDa).
Protein concentration was estimated by the Coomassie blue binding assayCitation30 with BSA as the standard.
Kinetic constants of the enzyme were calculated by non-linear regression analysis of at least five different rate measurements.
Results
Cys-Gly hydrolase purification
A soluble Cys-Gly hydrolase activity was purified from P. caerulea using a procedure including DE-52 ion exchange chromatography, treatment with chloroform/octanol, and Sephacryl S-300 gel filtration (). The purification was very rapid and the overall process was completed in two days. summarizes the purification procedure of the enzyme to apparent electrophoretic homogeneity.
Figure 1. Gel chromatography on Sephacryl S-300 column. See materials and methods for details. Inset: SDS/PAGE analysis of Cys-Gly hydrolase activity purified from P. caerulea.
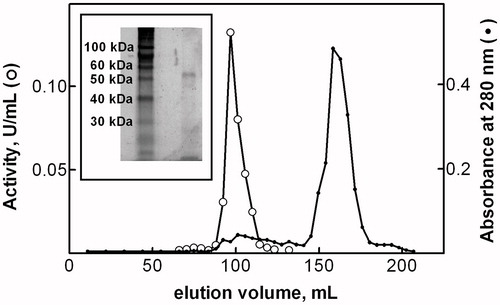
Table 1. Purification of P. caerulea Cys-Gly hydrolase.
Cys-Gly hydrolase activity was stable for at least one month at −20 °C in the preparation derived from the DE-52 batch step and for at least two weeks at 4 °C in the supernatant from chloroform/octanol treatment. MgCl2 was fundamental for the stability of the enzyme: the omission of MgCl2 before chloroform/octanol treatment or in the buffer used for S-300 chromatography resulted in the complete inactivation of the enzyme. Pure enzyme was stable for at least two weeks if stored at −80 °C.
Characterization of the pure enzyme
Purified Cys-Gly hydrolase activity showed a single band on SDS/PAGE with an apparent molecular mass of approx. 54 kDA (, inset). A molecular mass of approx. 319 kDA was evaluated for the native enzyme by gel filtration on Sephacryl S-300 (), thus suggesting a homo-hexameric structure for the enzyme.
The Cys-Gly hydrolase activity of the purified enzyme was completely lost if EDTA was added to the incubation mixture () and was recovered by the addition of an excess of MgCl2 or MnCl2 (data not shown). The susceptibility to inhibition by bestatin, a classical inhibitor of M17 family peptidases, was evaluated for the Patella enzyme. The results shown in indicate a great effectiveness of the inhibitor with an IC50 of 90 ± 7 nM as determined by non-linear regression analysis.
Figure 2. Effect of EDTA and bestatin on Cys-Gly hydrolase activity. Panel A: Purified enzyme was incubated both in the absence and in the presence of different concentrations of EDTA ranging from 0.25 to 10 mM. Panel B: Purified enzyme was incubated both in the absence and in the presence of different concentrations of bestatin ranging from 1 nm to 20 mM. Data are reported as percentage residual activity.
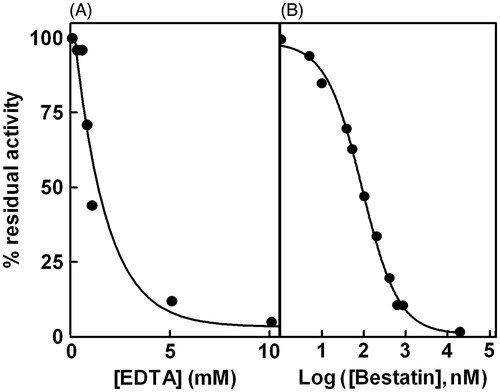
The effect of pH on the Cys-Gly hydrolase activity of the purified enzyme was evaluated both in terms of kcat and KM. The results of measurements at five different pHs are reported in . The affinity of the enzyme for Cys-Gly was approximately constant in the range of pH 6.5 to 8.5, while the turnover number appeared to increase with the increase in pH toward an apparent plateau at higher pH values (pH 8.0–8.5).
Table 2. Kinetic parameters of P. caerulea Cys-Gly hydrolase activity.
As a comparison, kinetic parameters of the purified enzyme were also determined at pH 7.5 and pH.8.5 for Leu-Gly, a common substrate of aminopeptidases. In this case, KM and turnover number values of 0.93 ± 0.19 mM and 12 000 ± 800 min− 1 and of 0.88 ± 0.29 mM and 20 000 ± 1500 min−1 were measured at pH 7.5 and pH 8.5, respectively. These values account for specificity constant values of 12 903 ± 2799 mM− 1 min−1 and 22 727 ± 7885 mM− 1 min− 1 at pH 7.5 and 8.5, respectively, that appear higher but still of the same order of magnitude as the specificity constants for hydrolysis of Cys-Gly (). For Cys-Gly hydrolysis, in fact, the lower turnover number compared to Leu-Gly hydrolysis is paralleled by a significant decrease in KM ().
Mass spectrometry characterization
To identify the purified enzyme, a sample of the SDS-PAGE band migrating at about 54 kDa was subjected to hydrolysis with trypsin and subsequent analysis by nLC-ESI-LIT-MS/MS; protein search of resulting MS data was performed against a non-redundant NCBI sequence database (selected taxonomy: Metazoa). The results are showed in Table S1 (Supplementary Table S1). Among the nine identified proteins, six of these (NCBI accessions: 676481827, 340374423, 675370293, 676494566, 669318572, 676446443) resulted as putative aminopeptidases of peptidase M17 family from various organisms, as it was confirmed by protein-protein BLAST search (http://blast.ncbi.nlm.nih.gov/Blast.cgi). These peptidases share parts of their sequences in highly conserved manner, as shown in a multiple sequence alignment (by MUSCLE v. 3.8, http://www.ebi.ac.uk/Tools/msa/muscle/) (), where the peptides sequenced are highlighted. This figure also shows that the identified peptide TVEINNTDAEGR is common to three out of the six aminopeptidases, and also to other 316 proteins from different organisms (Homo sapiens, Bos taurus, Mus musculus, and others) (see the column “Proteins” in the Table S1). Other sequenced peptides were specific for a single organism. For example, the peptides LGIPTIDIGAPQLSMHSIR, SYMLSADQAHAIHPNYSEK, TPSPFHAVDELK, VLVKNGDKIEHR identified unequivocally an aminopeptidase (NCBI accession: 676481827) from Lottia gigantea, a species of sea snail belonging to the Gastropoda class (similarly to P. caerulea). Analogously, the peptide IVDMPCAEMNTDDFITEIR (together with TVEINNTDAEGR) identified another aminopeptidase (NCBI accession: 676494566) from L. gigantea.
Discussion
Patella caerulea, a gastropod in the family Patellidae, lives on hard surfaces in the intertidal zone and is abundant in the Mediterranean Sea. Like other mollusks it is often exposed to extreme environmental conditions (UV irradiation, exsiccation/hydration cycles, environment pollutants) that can cause oxidative stress. The Cys-Gly hydrolase activity exhibited by different animal species is considered as part of the antioxidant mechanism that minimizes the pro-oxidant action of Cys-Gly, product of GSH breakdown.
The Cys-Gly hydrolase activity purified from P. caerulea displays a molecular mass of approximately 54 kDa in SDS-PAGE and appears as a homo-hexameric structure in its native state. The sensitivity of the enzyme to metal chelators reveals another distinctive feature of the purified enzyme in terms of its reversible depletion of functional prosthetic metal ions. In fact, treatment of the native enzyme with EDTA determines a complete loss of activity, which is, however, recovered upon the addition of an excess of Mg+ 2 or Mn+ 2 ions. Another distinctive feature common to peptidases of the M17 family, which is exhibited by the Cys-Gly hydrolase from Patella is its remarkable sensitivity to bestatin, able to inhibit the enzyme with an IC50 in the nM range. Moreover, the pH dependence of the kinetic parameters of the purified enzyme is consistent with the proposed mechanism of action of M17 family peptidases, that, as generally acceptedCitation25,Citation31, can catalyze peptide hydrolysis only when the α-aminogroup of the substrate is unprotonated. All these features and the mass spectrometry characterization of the purified enzyme lead to the conclusion that also in mollusks, Cys-Gly hydrolase activity is associated with a protein of the peptidase family M17. This association is a further evidence that enzymes of this family may have an important role in the metabolism of glutathione both by recruiting GSH-derived Cys and by reducing the pro-oxidant action of Cys-Gly.
Since it has been demonstrated that the activity of peptidases belonging to family M17 varies depending on the metal ions present in the active site, the enzymes of this family have been adopted as model enzymes to clarify the mechanisms of metal ion-dependent enzymatic catalysisCitation32–34. Our purification of the enzyme from Patella is an extremely fast procedure. The yield (approximately 5 mg of pure enzyme per liter of the mollusk extract) is well comparable with enzyme production by DNA recombinant techniques without the need of further manipulation, such as the removal of polyhistidine tag, to obtain the native enzyme formCitation35,Citation36. Thus, given the abundance and availability of the starting material, purification could make large amounts of protein available for structural studies.
Recently, metallopeptidases belonging to the M17 family have been reported to be involved in parasitic infections in humansCitation37–40; they help parasites to survive by acting in the preliminary stages of their development, as, for example, eggs hatch in SchistosomaCitation41 or the maturation of immature trophozoites in P. falciparumCitation42. Thus, the search for inhibitors of this enzyme activity has been proposed as a promising strategy for the treatment of various parasitic diseases. Studies on Cys-Gly hydrolase purified from Patella, while helping to clarify the role of metallopeptidases in organisms other than vertebrates, will provide insights into a new target to develop effective inhibitors against M17 family peptidases.
Conclusions
A Cys-Gly hydrolase activity from the mollusk P. caerulea was purified to electrophoretic homogeneity through a simple and fast purification protocol. Structural and kinetic studies allowed identifying the purified activity as a M17 family metallopeptidase. The potential of the Cys-Gly hydrolase from Patella as a target of inhibition is considered.
Supplementary material available online
Supplementary Table S1
IENZ_1158170_Supplemental_Information.pdf
Download PDF (245.1 KB)Declaration of interest
This work was supported by the University of Pisa.
References
- Oehlmann J, Schulte-Oehlmann U. Molluscs as bioindicators. In: Markert BA, Breure AM, Zechmeister HG, eds. Trace metals and other contaminants in the environment. Vol. 6. Bioindicators & biomonitors. Amsterdam: Elselvier; 2003:577–635
- Cranford PJ, Gordon DC, Lee K, et al Chronic toxicity and physiological disturbance effects of water- and oil-based drilling fluids and some major constituents on adult sea scallops (Placopecten magellanicus). Mar Environ Res 1999;48:225–56
- Widdows J, Donkin P, Brinsley MD, et al Scope for growth and contaminant levels in North Sea mussels Mytilus edulis. Mar Ecol Prog Ser 1995;127:131–48
- Wo KT, Lam PKS, Wu RSS. A comparison of growth biomarkers for assessing sublethal effects of cadmium on a marine gastropod, Nassarius festivus. Mar Pollut Bull 1999;39:165–73
- Alves de Almeida E, Celso Dias Bainy A, Paula de Melo Loureiro A, et al Oxidative stress in Perna perna and other bivalves as indicators of environmental stress in the Brazilian marine environment: antioxidants, lipid peroxidation and DNA damage. Comp Biochem Physiol A 2007;146:588–600
- Gillis PL, Higgins SK, Jorge MB. Evidence of oxidative stress in wild freshwater mussels (Lasmigona costata) exposed to urban-derived contaminants. Ecotoxicol Environ Saf 2014;102:62–9
- Jara C, Gaete H, Lobos G, Hidalgo ME. Oxidative stress in the mollusk Echinolittorina peruviana (Gasteropoda: Littorinidae, Lamarck, 1822) and trace metals in coastal sectors with mining activity. Ecotoxicology 2014;23:1099–108
- Valavanidis A, Vlahogianni T, Dassenakis M, Scoullos M. Molecular biomarkers of oxidative stress in aquatic organisms in relation to toxic environmental pollutants. Ecotoxicol Environ Saf 2006;64:178–89
- Tate SS, Meister A. Gamma-glutamyl transpeptidase: catalytic, structural and functional aspects. Mol Cell Biochem 1981;39:357–68
- Enoiu M, Aberkane H, Salazar JF, et al Evidence for the pro-oxidant effect of gamma-glutamyltranspeptidase-related enzyme. Free Radic Biol Med 2000;29:825–33
- Giustarini D, Campoccia G, Fanetti G, et al Minor thiols cysteine and cysteinylglycine regulate the competition between glutathione and protein SH groups in human platelets subjected to oxidative stress. Arch Biochem Biophys 2000;380:1–10
- Glass GA, Stark AA. Promotion of glutathione-gamma-glutamyl transpeptidase dependent lipid peroxidation by copper and ceruloplasmin: The requirement for iron and the effects of antioxidants and antioxidant enzymes. Environ Mol Mutagen 1997;29:73–80
- Stark AA, Glass G. Role of copper and ceruloplasmin in oxidative mutagenesis induced by the glutathione-gamma-glutamyl transpeptidase system and by other thiols. Environ Mol Mutagen 1997;29:63–72
- Stark AA, Zeiger E, Pagano DA. Glutathione metabolism by gamma-glutamyltranspeptidase leads to lipid peroxidation: characterization of the system and relevance to hepatocarcinogenesis. Carcinogenesis 1993;14:183–9
- Del Bello B, Paolicchi A, Comporti M, et al Hydrogen peroxide produced during gamma-glutamyl transpeptidase activity is involved in prevention of apoptosis and maintainance of proliferation in U937 cells. FASEB J 1999;13:69–79
- Maellaro E, Dominici S, Del Bello B, et al Membrane gamma-glutamyl transpeptidase activity of melanoma cells: effects on cellular H2O2 production, cell surface protein thiol oxidation and NF-kappa B activation status. J Cell Sci 2000;113:2671–8
- Perego P, Paolicchi A, Tongiani R, et al The cell-specific anti-proliferative effect of reduced glutathione is mediated by gamma-glutamyl transpeptidase-dependent extracellular pro-oxidant reactions. Int J Cancer 1997;71:246–50
- Cappiello M, Amodeo P, Mendez BL, et al Modulation of aldose reductase activity through S-thiolation by physiological thiols. Chem Biol Interact 2001;130–132:597–608
- Del Corso A, Vilardo PG, Cappiello M, et al Physiological thiols as promoters of glutathione oxidation and modifying agents in protein S-thiolation. Arch Biochem Biophys 2002;397:392–8
- Dominici S, Valentini M, Maellaro E, et al Redox modulation of cell surface protein thiols in U937 lymphoma cells: the role of gamma-glutamyl transpeptidase-dependent H2O2 production and S-thiolation. Free Radic Biol Med 1999;27:623–35
- Jones DP, Carlson JL, Mody VC, et al Redox state of glutathione in human plasma. Free Radic Biol Med 2000;28:625–35
- Grau EM, Marathe GV, Tate SS. Rapid purification of rat kidney brush borders enriched in gamma-glutamyl transpeptidase. FEBS Lett 1979;98:91–5
- Robinson DS, Birnbaum SM, Greenstein JP. Purification and properties of an aminopeptidase from kidney cellular particulates. J Biol Chem 1953;202:1–26
- Josch C, Klotz LO, Sies H. Identification of cytosolic leucyl aminopeptidase (EC 3.4.11.1) as the major cysteinylglycine-hydrolysing activity in rat liver. Biol Chem 2003;384:213–18
- Cappiello M, Lazzarotti A, Buono F, et al New role for leucyl aminopeptidase in glutathione turnover. Biochem J 2004;378:35–44
- Chu L, Lai Y, Xu X, et al A 52-kDa leucyl aminopeptidase from Treponema denticola is a cysteinylglycinase that mediates the second step of glutathione metabolism. J Biol Chem 2008;283:19351–8
- Renzone G, Arena S, Scaloni A. Proteomic characterization of intermediate and advanced glycation end-products in commercial milk samples. J Proteomics 2015;117:12–23
- Laemmli UK. Cleavage of structural proteins during the assembly of the head of bacteriophage T4. Nature 1970;227:680–5
- Wray W, Boulikas T, Wray VP, Hancock R. Silver staining of proteins in polyacrylamide gels. Anal Biochem 1981;118:197–203
- Bradford MM. A rapid and sensitive method for the quantitation of microgram quantities of protein utilizing the principle of protein-dye binding. Anal Biochem 1976;72:248–54
- Hanson H, Frohne M. Cristalline leucine aminopeptidase from lens. Methods Enzymol 1976;45:504–20
- Allen MP, Yamada AH, Carpenter FH. Kinetic parameters of metal-substituted leucine aminopeptidase from bovine lens. Biochemistry 1983;22:3778–83
- Cappiello M, Alterio V, Amodeo P, et al Metal ion substitution in the catalytic site greatly affects the binding of sulfhydryl-containing compounds to leucyl aminopeptidase. Biochemistry 2006;45:3226–34
- Carpenter FH, Vahl JM. Leucine aminopeptidase (bovine lens). Mechanism of activation by Mg2+ and Mn2+ of the zinc metalloenzyme, amino acid composition, and sulfhydryl content. J Biol Chem 1973;248:294–304
- Morty RE, Morehead JJ. Cloning and characterization of a leucyl aminopeptidase from three pathogenic Leishmania species. J Biol Chem 2002;277:26057–65
- Modak JK, Roujeinikova A. Cloning, purification and preliminary crystallographic analysis of the Helicobacter pylori leucyl aminopeptidase-bestatin complex. Acta Crystallogr Sect F 2013;69:1011–14
- Cadavid-Restrepo G, Gastardelo TS, Faudry E, et al The major leucyl aminopeptidase of Trypanosoma cruzi (LAPTc) assembles into a homohexamer and belongs to the M17 family of metallopeptidases. BMC Biochem 2011;12:46
- Changklungmoa N, Chaithirayanon K, Kueakhai P, et al Molecular cloning and characterization of leucine aminopeptidase from Fasciola gigantica. Exp Parasitol 2012;131:283–91
- Lee JY, Song SM, Seok JW, et al M17 leucine aminopeptidase of the human malaria parasite Plasmodium vivax. Mol Biochem Parasitol 2010;170:45–8
- Song SM, Park JH, Kim J, et al Identification and characterization of Paragonimus westermani leucine aminopeptidase. Parasitol Int 2008;57:334–41
- Rinaldi G, Morales ME, Alrefaei YN, et al. RNA interference targeting leucine aminopeptidase blocks hatching of Schistosoma mansoni eggs. Mol Biochem Parasitol 2009;167:118–26
- Maric S, Donnelly SM, Robinson MW, et al The M17 leucine aminopeptidase of the malaria parasite Plasmodium falciparum: importance of active site metal ions in the binding of substrates and inhibitors. Biochemistry 2009;48:5435–9