ABSTRACT
This study investigated the effect of adding vanadium (V) to natural manganese oxide (NMO) in ammonia (NH3) selective catalytic reduction (SCR). The addition of V to NMO decreased the catalytic activity at low temperatures by blocking the active site. However, the enhancement of catalytic activity was achieved by controlling NH3 oxidation at high temperatures. From the NH3 temperature programmed desorption and oxygen on/off test, it was confirmed that the amount of Lewis acid site and active lattice oxygen of the catalyst affects the catalytic performance at low temperature
Recently, NMO and manganese oxide have been reported as SCR catalysts. They usually have only reported the reaction characteristics and catalytic activity on the NH3 SCR over NMO or manganese/metal oxide catalysts. There are no studies about the effect of addition of V to NMO. Therefore, this study investigates the catalytic activity and reaction characteristics on the NH3 SCR over NMO and V/NMO, and a new application is proposed based on the conclusions of this study.
INTRODUCTION
Recent attempts to solve some of the numerous environmental problems caused by rapid industrialization have been unsuccessful because of ever-increasing energy consumption.Citation1 These environmental problems have various classifications, but air pollution has emerged as the focus of international regulation because it is not contained in its country of origin. Indeed, air pollution with its unique ability to diffuse into adjacent areas and countries is now threatening the global environment. Oxides of nitrogen (NOx) and sulfur (SOx) are two of the main source materials causing air pollution. The amount of SOx exhaust produced annually is being reduced by the removal of sulfur content in fuels, installation of flue-gas desulfurization facilities, use of clean fuels, etc. More than 70% of NOx originates from a fixed source such as a thermal power station or industrial facility. Such NOx species are a continuing problem because their removal is more diffi-cult than the SOx. At the moment, selective catalytic reduction (SCR) using ammonia (NH3) as a reductant for NOx diffusion from a fixed source is recognized as the most technically and economically efficient removal method,Citation2 for which vanadium(V) oxide (V2O5) is the most widely used catalyst. The most essential technology of an SCR process is the catalyst.
Bosch and JanssenCitation3 classified the basic metallic oxides for SCR with NH3 (NH3 SCR) as V2O5, iron(III) oxide (Fe2O3), copper(II) oxide (CuO), cobalt oxide (Co3O4), chromium(III) oxide (Cr2O3), nickel(II) oxide (NiO), cerium(IV) oxide (CeO2), lanthanum(III) oxide (La2O3), praseodymium oxide (Pr6O11), neodymium(III) oxide (Nd2O3), gadolinium oxide (Gd2O3), ytterbium oxide (Yb2O3), and so on, all of which have been studied extensively. Among these, V2O5 was found to have the highest catalytic activity. Furthermore, the catalyst support used in SCR also plays an important role in the efficiency of reduction.Citation4 Bauerle et al.Citation4a reported that V2O5 has the highest catalytic activity when titanium dioxide (TiO2) or aluminum oxide (Al2O3) is used as support. Shikada et al.Citation5 found through a systematic study that catalytic activity increases with the use of the TiO2 support and that the catalytic activities using various supports are in the following order: TiO2-silicon dioxide (SiO2) >γ-Al2O3 > SiO2. Additionally, Pearson et al.Citation6 showed that a TiO2 support in the form of anatase rather than rutile has higher catalytic activity.
Previously, Markvart et al.Citation7 and Choi et al.Citation8 reported that manganese oxide showed excellent catalytic activity in NH3 SCR at low temperatures, and its reactivity was verified using manganese oxide itself. However, there is almost no report of manganese oxide being positioned as a support but used as a catalyst. Therefore, the role of a support in SCR is very important and can potentially be the basis for manufacture of future catalysts.
Accordingly, this study examined the reaction characteristics and catalytic activity of manganese oxide impregnated with vanadium (V) in NH3 SCR at low temperatures. The authors further attempted to investigate the factors controlling the catalytic activity and reaction characteristics.
EXPERIMENTAL PROCEDURES
Catalysts Preparation
Natural manganese oxide (NMO) exists mostly in two forms—pyrolusite and psilomelane—and in various other forms such as manganite, braunite, and hausmanite. The NMO used in this study was pyrolusite, composed mainly of β-MnO2, and its physicochemical properties are listed in .
Table 1a. Chemical properties of NMO
Table 1b. Physical properties of NMO
To increase the catalytic activity of NMO, the amount of V to be impregnated was calculated according to the desired composition ratio, and the amount of calculated ammonium metavanadate (NH4VO3; Aldrich Chemical) was dissolved in distilled water heated to 60 °C. To determine the effect of pH on the sample, V/NMO was prepared in the presence of oxalic acid (OA; Aldrich Chemical). OA, corresponding to a 1:1 molar ratio of the calculated quantity of V, was added. After the mixture was prepared in slurry state, it was agitated for more than 1 hr, and its moisture content was evaporated at 70 °C using a rotary vacuum evaporator (NN Series, Eyela).
After drying the material in a dry oven at 110 °C for 24 hr, the samples were prepared by calcination by heating to the desired temperature at a rate of 5 °C/min in a tubular furnace under air for 4 hr. The samples prepared by the addition of V are denoted as “1% V/NMO and 2% V/NMO” and the sample prepared with the addition of OA is denoted as “1% V/NMO OA.”
Catalytic Activity Measurement
The activity test equipment included a gas injector, reactor, and reaction gas analyzer. The flow rate of gas (nitric oxide [NO], nitrogen [N2], oxygen [O2], and NH3) into the reactor from each cylinder was controlled using a mass flow controller (MFC; MKS). Additionally, moisture was supplied to the reactor by injection of N2 containing water vapor through the bubbler, and this level of moisture was constantly maintained by the circulation of water at a fixed temperature of 50 °C by a circulator outside of the jacket-type bubbler. The entire gas supply pipe was made of stainless steel and was wrapped with a heating band set at 180 °C so that the moisture would not condense.
The continuous-flow fixed-bed reactor that was used had a quartz pipe with an inner diameter of 8 mm and height of 60 cm and quartz wool for fixing the sample layer. The temperature of the reactor was controlled by a proportional-integral-derivative (PID) temperature controller using a K-type thermocouple positioned at the lower part of the fixed bed. Furthermore, the difference between the before and after sample layer temperatures was measured by installing another thermocouple on the upper part of the sample layer to measure the temperature of the gas inlet.
To measure the concentrations of the reactants and products of NO, Uras 10E (Hartman & Braun) and 42C HL (Thermo Instruments) were used. In the case of nitrogen dioxide (NO2), a detector tube (9L, Gastech) was used when more than 5 parts per million (ppm) of NH3 was exhausted, and the abovementioned 42C analyzer was used when less than 5 ppm of NH3 was exhausted. The concentration of NH3 was measured using the detector tube (3M, 3La, 3L, Gastech).
Characterizations
The specific surface area of the samples was measured with ASAP 2010C from Micromeritics. Here, each specimen was analyzed after being degassed into a vacuum state at 110 °C for 3–5 hr.
To observe the crystal structure of NMO and V/NMO, X-ray diffraction (XRD) analysis was performed and XRD patterns were analyzed with a D/Max-III (3 kW) diffractometer (Rigaku). Cu Kα (λ= 0.1506 nm) was used as a radiation source, and measurements were made in the range of 2θ= 10–90° at a scanning rate of 4°/min.
NH3 temperature programmed desorption (TPD) measurements were carried out with 50 mg of the sample from room temperature to 400 °C at a rate of 10 °C in flowing argon (Ar) gas (50 ml/min) after exposure of the NH3/Ar gas (1:99 v/v mixtures with a total flow of 50 ml/min).
X-ray photoelectron spectroscopy (XPS) analysis was performed using ESCALAB 210 (VG Scientific), and Al Kα monochromate (1486.6 eV) was used as an excitation source. After moisture contained in the samples was removed completely through drying at approximately 100 °C for 24 hr, the samples were analyzed without surface sputtering and etching to maintain the vacuum pressure of the XPS equipment at 10−6 Pa.
RESULTS AND DISCUSSION
Characterizations and Catalytic Activities of NMO and V/NMO
The analysis results of NMO and V/NMO used in this study are listed in . The pH of the solution used during preparation of the samples ranged from 3.8 to 6.8 as per the amount of added OA. The pH of the 1% V/NMO OA sample solution had a decreasing tendency, whereas that of the V/MNO samples showed a higher pH than that of NMO. Additionally, among OA-added samples, the more the content of V increased, the less the pH decreased. As pointed out earlier, the amount of OA added depends on the amount of V impregnated. A greater amount of impregnated V requires the addition of a greater amount of OA, with pH decreasing drastically. With increasing V contents, specific surface area shows a little decreasing tendency. In , the crystalline structure of NMO and V/NMO used in this test, determined by XRD analysis, is shown; all of the samples showed the structure of typical β-MnO2.
Table 2. Physicochemical properties and NOx conversion over NMO and V/NMO
In , the activities of NMO, V/NMO, and V/NMO OA are shown. Although NMO shows the highest catalytic activity in the low-temperature range of less than 350 °C, this activity drastically decreases at 300 °C. In the case of V/NMO, the more the content of V increases, the less the activity decreases and the less activity the OA-added samples show. The cause of these phenomena can be explained by the XPS results in . The OA-added samples have a greater number of V atoms exposed on the surface than the samples without any OA added. The number of atoms exposed on the surface is used in the same sense as a degree of dispersion, which is often used for representing the turnover frequency (TOF) of catalyst.Citation9 Accordingly, the above result implies that the degree of dispersion increased by the low pH. The samples by V content also show that an increase in the V content results in more V atoms being exposed on the surface. The increased number of V atoms exposed on the surface controls the exposure of NMO on the surface. Accordingly, because V/NMO shows lower activity than NMO in the range of low temperatures because of V atoms having blocked the active site of the NMO surface, it can be concluded that the activity of V/NMO samples at a low temperature is determined by the degree of dispersion of V
NH3 and NO Oxidation
To evaluate the SCR reactive characteristics of NMO and V/NMO in the high-temperature range, NH3 and NO oxidation tests were conducted. The NH3 oxidation is shown in . The experimental conditions were the same as those in the catalytic activity test, except that there was no injection of NO in the oxidation test. The results show that NMO promoted the highest rate of NH3 oxidation throughout the temperature range, and a higher quantity of V resulted in decreased NH3 oxidation. NMO showed complete oxidation of NH3 at 300 °C, whereas the V/NMO samples exhibited incomplete NH3 oxidation at 350 °C. All samples showed the specific phenomena (lack of nitrogen components regarding balance) in an aspect of nitrogen balance when regarding the NH3 oxidation, generating NO and NO2. Therefore, an experiment using mass spectroscopy was performed to explain the phenomena, as presented in . With increasing temperature, the amount of NH3 decreased and N2, water, and NO increased. Therefore, it was assumed that the generated N2 corresponded to the lack of nitrogen components in .
Figure 3. Effect of temperature on NH3 oxidation over NMO and V/NMO (SV = 60,000 hr−1, NH3 = 225 ppm, water vapor = 8%, O2 = 15%, NO = 0, NO2 = 0).
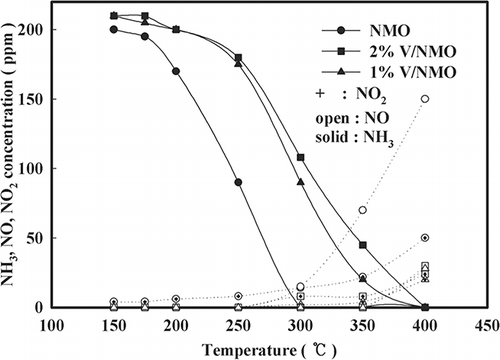
Figure 4. Effect of temperature on NH3 oxidation over NMO as detected by mass spectroscopy (SV = 60,000 hr−1, NH3 = 225 ppm, water vapor = 8%, O2 = 15%, NO = 0, NO2 = 0).
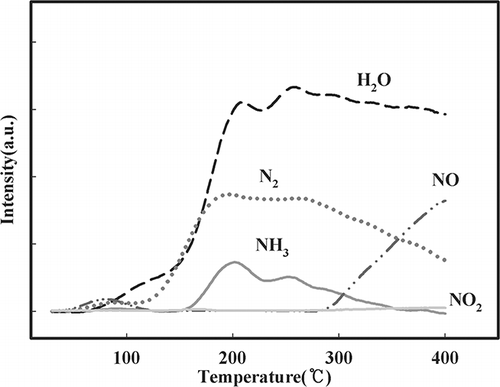
The NO oxidation is shown in . The NO oxidation test was carried out under the same conditions as the NH3 oxidation test (). NMO had the highest conversion rate of NO to NO2 at all reaction temperatures, and a higher quantity of impregnated V resulted in a reduced conversion rate. A higher ratio of NO2 content in the reactant gas caused an increase in the SCR rate, as in Equationeq 2 of standard SCR (Equationeq 1), which in turn resulted in increased catalytic activity.Citation10 However, the SCR reaction over NMO at high temperature in this study was very slow because there was a shortage of NH3 because of the conversion of NO to NO2 (by the fast SCR). Therefore, it was assumed that the low catalytic activity of NMO at high temperature (300 °C), as shown in , was because of the fast rate of NO oxidation and a shortage of NH3 as reductant. Additionally, in the case of V/NMO, the addition of V blocked the active site on NMO and resulted in low activity at low temperature.
Figure 5. Effect of temperature on NO oxidation over NMO and V/NMO (SV = 60,000 hr−1, NO = 190 ppm, NO2 = 20 ppm, water vapor = 8%, O2 = 15%).
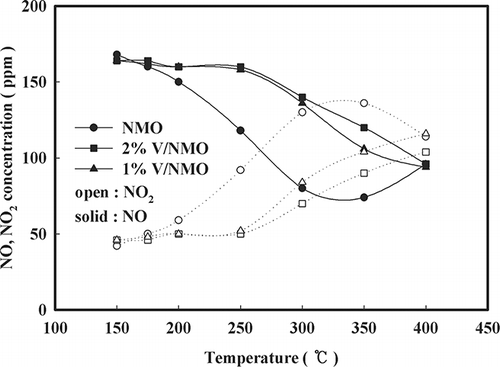
Characteristics of NH3 Adsorption and Desorption
Determining the characteristics of NH3 adsorption to and desorption from the sample surface is integral to understanding SCR with NH3. Therefore, a TPD test was performed on NMO impregnated with V.
Results of a typical NH3 TPD test with NMO are shown in . According to Lietti et al.,Citation11 the ad sorbed NH3 species present on the surface of V2O5/TiO2 are of two types: the molecular form of NH3 adsorbed at Lewis acid sites (hereafter referred to as “L acid sites”) and NH4 + adsorbed at Bronsted acid sites (hereafter referred to as “B acid sites”). As previously shown by Busca et al.,Citation12,Citation13 the NH3 species adsorbed at L acid sites is more stable than that adsorbed at B acid sites at high temperatures. In the results presented here, the characteristics of the two types of NH3 species absorbed to NMO were the same as those for V2O5/TiO2. Specifically, NH3 that desorbed at temperatures lower than 100 °C was adsorbed at B acid sites, which implies that the stable form adsorbed at L acid sites at temperatures higher than 100 °C. The NH3 species adsorbed at L acid sites required a high temperature for desorption on the basis of the strong interaction with the surface. In contrast, the species desorbed from the weak acid sites was in the form of NO, nitrous oxide (N2O), or N2. The oxygen of the produced material originated from the active lattice oxygen or the oxygen adsorbed to the surface because no oxygen was injected.
Qingya et al.Citation14 reported that the most important factor in NH3 SCR is the amount of NH3 adsorbed at L acid sites. Accordingly, the correlation between the quantity of NH3 adsorbed at the various acid sites and catalytic activity was surveyed. The values that indicate the amount of NH3 adsorbed to the weak and strong acid sites as well as the amount of NH3 adsorbed to the weak acid sites of NMO and V/NMO are shown in , along with the conversion rates of NOx. It was difficult to observe any correlation between NOx conversion rates at low temperatures and the amount of NH3 adsorbed to the weak and strong acid sites. Accordingly, the correlation characteristics between the amount of NH3 adsorbed at L acid sites and catalytic activity are shown in . The amount of NH3 adsorbed at L acid sites significantly affects the catalytic activity at low temperatures but does not follow a linear relationship at high temperatures. This is because the catalytic activity of NMO decreased rapidly. As mentioned previously, the catalytic activity of NMO decreased rapidly at temperatures higher than 250 °C upon the fast oxidation of NH3. These characteristics seem to suggest that the amount of NH3 adsorbed at L acid sites is correlated only with catalytic activity at low temperatures. Additionally, the above results imply that the rate of NOx conversion at high temperatures is more dependent on the catalytic oxidation rate than on the amount of adsorbed NH3.
Redox Characteristics
The main factors affecting SCR are the redox and adsorption properties of the sample, especially adsorption to the acid sites. As such, the redox properties at low temperatures play an important role in SCR.Citation15–17 These redox properties depend on the lattice oxygen of the sample, the reductant NH3, and gaseous oxygen. However, the role of oxygen is controversial. Absence of NO from the reaction because of decomposition to N2 and O2 encourages the participation of the other two types of oxygen—the active lattice oxygen and gaseous oxygen—in the reaction. Therefore, the reaction mechanism can be categorized into the following three types according to the different types of oxygen.
The first category involves the lattice oxygen supplemented with gaseous oxygen. The second involves direct participation of gaseous oxygen in the reaction. Finally, the last category comprises oxygen changing the oxidation state of the sample without participation in the reaction.
Regarding the reaction mechanism of oxygen, Lietti et al.Citation17 stated that SCR occurs by a redox mechanism and that the oxygen participates in the reoxidation of the reduced catalyst.
To determine the degree of participation of the lattice oxygen in the sample reaction, an O2 on/off test was performed, and the results are shown in . Upon ceasing the administration of oxygen, the rate of NOx conversion decreased instantaneously, which was followed by increases at 200 °C and 180 °C as well as another decrease as time passed. This result is attributed to the gaseous oxygen and the lattice oxygen participating in the SCR reaction. Additionally, the higher the reaction temperature, the higher was the conversion rate. Therefore, the degree of participation of the lattice oxygen in the reaction increased with increasing temperature.
Figure 9. Decrease in NOx conversion with time after O2 on-off over NMO (SV = 60,000 hr−1, NO = 190 ppm, NO2 = 20 ppm, water vapor = 8%, O2 = 15%, NOx/NH3 = 1).
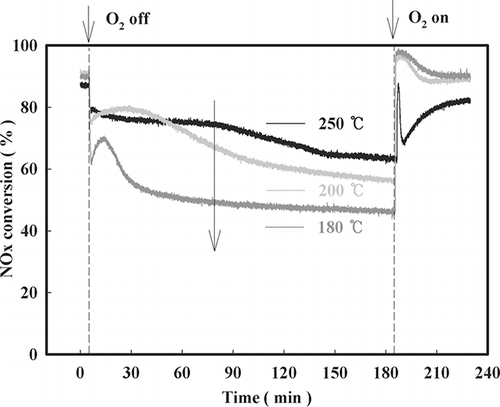
The O2 on/off test was performed to determine the degree of participation of the lattice oxygen of NMO and V/NMO in the SCR reaction (). Upon ceasing the administration of oxygen, NMO impregnated with V experienced a significant decrease in the rate of NOx conversion that continued to decrease over time. On the other hand, although simple NMO also initially experienced a significant decrease in the rate of NOx conversion, this rate remained constant for approximately 30 min.
Figure 10. Decrease in NOx conversion with time after O2 on-off over NMO, 1%V/NMO, and 2%V/NMO (SV = 60,000 hr−1, NO = 190 ppm, NO2 = 20 ppm, water vapor = 8%, O2 = 15%, NOx/NH3 =1 at 200 °C).
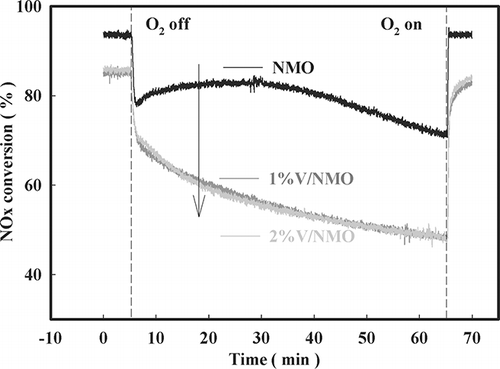
Thereafter, the rate of NOx conversion showed the same gradually decreasing trend as observed for V/NMO. The active lattice oxygen participates in the SCR reaction when no other oxygen is available. However, impregnation of the sample with V results in a decrease in lattice oxygen participation. The amount of active lattice oxygen was calculated relatively, and the calculation result is listed in . Choi et al.Citation8 and Seo et al.Citation18 reported that the higher the amount of activated lattice oxygen, as calculated by the O2 on/off test, the higher is the catalytic activity at low temperatures. In this study, the high catalytic activity of NMO at low temperatures was therefore due to a large amount of active lattice oxygen.
Table 3. Relative amount of active lattice oxygen in NMO and V/NMO
CONCLUSIONS
The following conclusions were drawn based on results of various tests of NMO impregnated with V: • Samples impregnated with V resulted in decreased catalytic activity at low temperatures. However, V/NMO exhibited higher activity than NMO at high temperatures. This result is closely linked with the oxidation rate of NH3 and is mainly attributed to decreased catalytic activity at high temperatures because of the shortage of reductant NH3.
• | The activity of the SCR catalyst at lower temperatures is closely linked to the amount of NH3 adsorbed at L acid sites on the catalyst bed and to the amount of active lattice oxygen. Therefore, the reaction characteristics and activity of SCR at low temperatures using NMO impregnated with V are important factors affecting the adsorption characteristics of NH3 and the participation of lattice oxygen in the reaction. | ||||
• | Upon addition of V to NMO, catalytic activity was reduced through the limitation of available lattice oxygen on the sample bed and the adsorption of NH3 at L acid sites that also blocks the active site. However, in the case of V/NMO without any OA added, relatively excellent activity was observed even in the low-temperature range. Therefore, V/NMO can potentially be used for the development of a catalyst functional over a wide temperature range. |
REFERENCES
- Forzatti , P. 2000 . Environmental Catalysis for Stationary Applications . Catal. Today , 62 : 51 – 65 .
- Kim , S.S. and Hong , S.C. 2007 . The Emission of NO2 and NH3 in Selective Catalytic Reduction over Manganese Oxide with NH3 at Low Temperature . J. Korean Ind. Eng. Chem. , 18 : 255 – 261 .
- Bosch , H. and Janssen , F. 1988 . Formation and Control of Nitrogen Oxides . Catal. Today , 2 : 369 – 379 .
- Markvart , M. and Pout , V. 1975 . Selective Reduction of Nitrogen Oxides by Ammonia Using Non-platinum Catalysts . Ind. Chem. Eng. , 15 : 546 – 551 .
- Bauerle , G.L. , Wu , S.C. and Ken , Nobe . 1978 . Parametric and Durability Studies on NOx Reduction with NH3 on Fe-Cr Oxide Catalysts . Ind. Eng. Chem. Prod. Res. Dev. , 17 : 123 – 128 .
- Shikada , T. , Fudimoto , K. , Kunugi , T. and Tominaga , H. 1983 . Reduction of Nitric Oxide with Ammonia on Silica-Supported Vanadium Oxide Catalysts . J. Chem. Tech. Biotechnol. , 33 : 446 – 447 .
- Pearson , I.M. , Ryu , H. , Wong , W.C. and Nobe , K. 1983 . “Chemical Mixed” Catalysts . Ind. Eng. Chem. Prod. Res. Dev. , 22 : 381 – 382 .
- Singoredjo , L. , Kover , R. , Kapteijn , F. and Moulijn , J.A. 1992 . Alumina Supported Manganese Oxides for the Low-Temperature Selective Catalytic Reduction of Nitric Oxide with Ammonia . Appl. Catal. B , 1 : 297 – 316 .
- Choi , S.H. , Lee , D.K. , Hong , S.I. and Hong , S.C. 2009 . Characterization of Mixtures of Natural Manganese Ore with V/TiO2 in Selective Catalytic Reduction of NO with NH3 . Environ. Eng. Sci. , 26 : 1607 – 1613 .
- Amiridis , M.D. , Wacks , I.E. , Deo , G. , Jehng , J.M. and Kim , D.S. 1996 . Reactivity of V2O5 Catalysts for the Selective Catalytic Reduction of NO by NH3: Influence of Vanadia Loading, H2O, and SO2 . J. Catal. , 161 : 247 – 253 .
- Tronconi , E. , Nova , I. , Ciardelli , C. , Chatterjee , D. and Weibel , M. 2007 . Redox Features in the Catalytic Mechanism of the “Standard” and “Fast” NH3-SCR of NOx over a V-Based Catalyst Investigated by Dynamic Methods . J. Catal. , 245 : 1 – 10 .
- Lietti , L. , Alemany , J.L. , Forzatti , P. , Busca , G. , Ramis , G. , Giamello , E. and Bregani , F. 1996 . Reactivity of V2O5-WO3/TiO2 Catalysts in the Selective Catalytic Reduction of Nitric Oxide by Ammonia . Catal. Today , 29 : 143 – 148 .
- Busca , G. , Lietti , L. , Ramis , G. and Berti , F. 1998 . Chemical and Mechanistic Aspects of the Selective Catalytic Reduction of NOx by Ammonia over Oxide Catalysts: a Review . Appl. Catal. B , 18 : 1 – 36 .
- Gutierrez-Aliejandre , A. , Ramirez , J. and Busca , G. A . 1998 . Vibrational and Spectroscopic Study of WO3/TiO2-Al2O3 Catalyst Precursors . Langmuir , 14 : 630 – 639 .
- Qingya , L. , Zhenyu , L. and Chengyue , L. 2009 . Adsorption and Activation of NH3 during Selective Catalytic Reduction of NO by NH3 . Chin. J. Catal. , 27 : 636 – 646 .
- Pinaeva , L.G. , Suknev , A.P. , Budneva , A.A. , Paukshtis , E.A. and Bal'Zhinimaev , B.S. 1996 . On the Role of Oxygen in the Reaction of NO Reduction by NH3 over Monolayer V2O5-TiO2 Catalyst . J. Mol. Catal. A , 112 : 115 – 124 .
- Lietti , L. , Ramis , G. , Berti , F. , Toledo , G. , Robba , D. , Busca , G. and Forzatti , P. 1998 . Chemical, Structural and Mechanistic Aspects on NOx SCR over Commercial and Model Oxide Catalysts . Catal. Today , 42 : 101 – 116 .
- Lietti , L. , Forzatti , P. and Bregani , F. 1996 . Steady-State and Transient Reactivity Study of TiO2 Supported V2O5-WO3 De-NOx Catalysts: Relevance of the Vanadium-Tungsten Interaction on the Catalytic Activity . Ind. Eng. Chem. Res. , 35 : 3884 – 3892 .
- Seo , P.W. , Lee , J.Y. , Shim , K.S. , Hong , S.H. , Hong , S.C. and Hong , S.I. 2009 . The Control of Valence State: How V/TiO2 Catalyst Is Hindering the Deactivation Using the Mechanochemical Method . J. Hazard. Mater. , 165 : 39 – 47 .