Abstract
Cancer cell-derived micro-particles (MPs) play important regulatory roles on cellular and system levels. These activities are attributed in part to protein factors carried by MPs. However, recruitment strategies for sequestering certain protein factors in MPs are poorly understood. In the current study, using exogenous and endogenously expressed phospholipid-binding probes, we investigated the distribution of membrane phospholipids in MPs as a potential mechanism for electrostatically enriching cationic protein factors in MPs. We detected a significant level of externalised phosphatidylethanolamine (PE) at the outer surface of MPs. This was accompanied, in the inner leaflet of the MP membrane, by a greater density of negatively charged phospholipids, particularly phosphatidylserine (PS). The local enrichment of PS in the inner surface of MPs was correlated with an elevated presence of small GTPases in a polybasic region (PBR)-dependent fashion. By employing a series of RhoA derivatives, including constitutively active and RhoA derivatives lacking a PBR, we could demonstrate that the congregation of RhoA in MPs was dependent on the presence of the PBR. A chimer with the fusion of PBR sequence alone to GFP significantly enhanced GFP localisation in MPs, indicative of a positive contribution of electrostatic interactions in RhoA recruitment to MPs. Using in silico thermodynamic simulations, we characterised the electrostatic interactions between PBR and anionic lipid membrane surface. In summary, the redistribution of membrane phospholipids in MPs has an impact on the local ionic density, and is likely a contributing factor in the electrostatic recruitment of membrane-associated proteins to MPs in a PBR-dependent fashion.
To access the supplementary material to this article, please see Supplementary files under Article Tools online.
Cancer cell-derived micro-particles (MPs) are an important means of cell-cell communication (Citation1, Citation2). The MPs are membrane-bound vesicles shed from viable cells; therefore, they are distinct from apoptotic bodies. They also differ from exosomes that are generated as multivesicular bodies from the endosomal pathway. With a typical diameter in the micrometre range, the MPs are delivery vehicles for signalling factors and play important regulatory roles in cellular survival and neoplastic transformation.
Membrane reorganisation is an integral part of MP formation. How this process contributes to the structure and function of MPs is of interest. In resting conditions, the phospholipids in the plasma membrane (PM) of a eukaryotic cell are asymmetrically distributed across the bilayer. Phosphatidylcholine (PC) and sphingomyelin (SM) are predominantly in the outer layer, whereas phosphatidylethanolamine (PE), phosphatidylserine (PS) and phosphatidylinositides (PIs) are constituents of the inner leaflet (Citation3). While PE has a neutral net charge, PS and PIs are anionic.
The lateral and transbilayer redistribution of phospholipids have recently been shown to have regulatory roles. Local enrichment of PS at the inner membrane surface is essential for the maintenance of cell polarity in budding yeast (Citation4, Citation5). This alters the electrostatic potential which, in turn, can influence the localisation of cationic membrane-interacting proteins. Processes regulated in this fashion include the polybasic region (PBR)-dependent membrane association of small GTPases (Citation4–Citation6). This process is accompanied by the transbilayer redistribution of PE to the outer leaflet of the PM, where the departure of PE from the inner leaflet is thought to leave space for a greater density of anionic phospholipids. In mammalian cells, local enrichment of PS/PIs at the inner leaflet of the PM mediates the recruitment of RhoA at the cleavage furrow during cytokinesis, where PE externalisation is essential for the completion of cell division (Citation6). TAT-5, which is a PE-specific P4 ATPase in Caenorhabditis elegans, suppresses the budding of extracellular vesicles presumably by preventing the externalisation of PE (Citation7). These pieces of evidence are implicative of a key role of phospholipid redistribution as a signalling strategy in vesicle formation by modulating membrane curvature and electrostatic density.
The goal of the current investigation was to characterise the distribution of phospholipids in the membrane of MPs using exogenous and endogenously expressed probes, and to explore potential roles of membrane electrostatic potential in recruiting protein factors. Here, we report a presence of PE on the outer surface of MPs derived from breast cancer cells. This was concomitant with an enrichment of PS in the inner surface of vesicular membrane, and the colocalisation of RhoA in a PBR-dependent manner. We applied in silico thermodynamic calculations to quantitatively characterise the nature and molecular mechanisms of PBR-membrane interactions. These findings shed light on an active role of phospholipid rearrangement in modulating membrane ionic density and contributing to the recruitment of membrane-associated protein factors.
Materials and methods
Cell, reagents and plasmids
Human breast cancer cell line MDA-MB-231 was purchased from ATCC. RhoA antibody (sc-418) and donkey anti-mouse IgG-FITC (sc-2099) were purchased from Santa Cruz. Duramycin, biotin and phalloidin tetramethylrhodamine (Cat#P1951) were from Sigma; biotinylated duramycin was synthesised as described earlier (Citation8); avidin-Texas red conjugate (A820) and avidin-FITC (Cat#43-4411) were from Life Technologies; Zeba desalt spin column (Cat#89890) was from Thermo Scientific. GFP-tagged wild-type RhoA (GFP-RhoA), constitutively active RhoA (GFP-CA-RhoA), wild-type RhoA with positive charges in the PBR mutated to Q (GFP-RhoA-PBRQ) and constitutively active RhoA with positive charges in the PBR mutated to Q (GFP-CA-RhoA-PBRQ) were prepared and used as previously described (Citation9). Lact-C2-GFP (Cat#22852), 2PH-PLCdelta-GFP (Cat#35142) and PM-GFP (Cat#21213) were purchased from Addgene. LifeAct–TagGFP2 (Cat#60101) was purchased from Ibidi.
Plasmid construction
RhoA C-terminal 14 aa was added to EGFP C-terminal to form a fusion protein GFP-PBR by inserting a polymerase chain reaction (PCR) product using GFP-WT-RhoA as template and digested with BglII and XhoI into pEGFP-C1 BglII and XhoI sites. Primers used for EGFP-PBR were: ctgacAGATCTcaagctagacgtgggaagaaa and ctgacGTCGACtcacaagacaaggcaaccaga. Its PBRQ mutant form was constructed by site-directed mutagenesis using the following primers: ggactcagatctcaagctcaacaggggcagcaacaatctggttgccttgtcttg and caagacaaggcaaccagattgttgctgcccctgttgagcttgagatctgagtcc. The plasmids were sequenced to confirm having correct sequences.
Cells were treated with 2.5 µg/ml of biotin-conjugated Duramycin for 10 min in DMEM containing 10% FBS. Cells were washed with media 3 times and Texas Red or FITC-conjugated avidin was added to a final concentration of 2.5 µg/ml for another 10 min. After washing for 3 times in culture media, cells were used for microscopy studies.
Confocal microscopy
Micrographs were acquired using Zeiss LSM510 confocal microscope or spinning disc confocal microscope. For Zeiss LSM510, the confocal system is based on a microscope with 40× or 63×water immersion objective lenses, equipped with an Argon laser or HeNe laser. For the spinning disc, the system is based on an Axiovert 200 M microscope with 63× or 100×oil immersion objective lenses, equipped with diode-pumped solid-state lasers (440, 491, 561, 638 and 655 nm) and a motorised XY stage. For live cell imaging, cells were seeded in a 35-mm coverslip glass plate with HEPES-buffered DMEM and the plate was transferred to a chamber that was placed in a microscope stage heater set to 37°C.
For microscopy data processing, the following procedures were followed using the Nikon NIS-Elements software. To determine the regional signal intensity along a segment of membrane, a line is drawn perpendicular to the membrane to generate a linear plot of signal intensity values as a function of space. The value at the membrane interception was noted. This process was repeated at 5 random locations within the membrane segment for calculating the average signal intensity. This method was used to determine the membrane signal intensity at the MPs and PM. To determine the rate of colocalisation between 2 fluorescent colours, the signal intensity of each colour was determined using the above-mentioned method. A positive localisation for each colour at the MPs is a value where the mean signal intensity is at least 2 standard deviations greater than that of the PM. Signal intensity values of each colour were determined in random MPs, and a colocalisation is considered positive when the signal intensity of both colours was at least 2 standard deviations greater than the PM.
Immunostaining for RhoA
MDA-MB-231 cells grown on coverslips were washed twice with PBS. Cold methanol was added to fix the cells for 10 min at −20°C. Cells were washed with PBS twice again for a total of 20 min. One millilitre of blocking buffer, which consisted of 5% normal donkey serum (Santa Cruz, Cat#sc-2044) and 0.3% Triton X-100 (Sigma, Cat#T-9284) in DPBS, was directly added into the 35-mm plate to cover the cells, the plate was left at room temperature for 1 hour. Then, 30 µl of RhoA antibody (diluted 1:50 with the blocking buffer) was added and the chamber was left at 4°C overnight. On the following day, the coverslip was washed with PBS 3 times, 10 min each time. Then, 30 µl of secondary antibody or phalloidin (diluted with the blocking buffer) was added and incubated for 1 hour. After rinsing and washing the coverslip 4 times with PBS and with deionised water once, the coverslip was mounted with an anti-fade media containing DAPI for microscopy.
Western blot
Proteins from total cell lysates were resolved by SDS-PAGE, and transferred to nitrocellulose membrane, blocked in 5% non-fat milk and blotted with the Anti-RhoA antibody.
Thermodynamic calculations
To quantitatively characterise the interactions between RhoA and the MP inner membrane leaflet, we applied a molecular theory approach to examine the adsorption of the C-terminal PBR onto a multicomponent phospholipid bilayer. This theoretical approach has previously been successfully applied to lipid bilayers as well as other biological relevant systems (Citation10–Citation17). The molecular theory considered a very large set of conformations of each of the different molecular species in the system and the probability of each conformation was obtained through a free energy minimisation that includes all energetic and entropic contributions. Two forms of RhoA PBR were studied: one where the original amino acid sequence of the RhoA protein was maintained (RhoA-PBR) and the other where the cationic amino acid residues in the PBR were substituted with glutamines, which had no net charge (RhoA-PBRQ). Each amino acid was represented as 1 bead with the amino acid's characteristic charge and an effective diameter of 0.376 nm. To account for the geranylgeranyl moiety, a hydrocarbon anchoring chain consisting of 20 segments was attached to the C-terminal cysteine prenylated amino acid residue. The model phospholipid membrane composed of 2 leaflets with the 3 most common types of phospholipids: dipalmitoylphosphatidylcholine (DPPC), dipalmitoylphosphatidylethanolamine (DPPE) and dipalmitoylphosphatidylserine (DPPS). To simulate mammalian membranes with a range of phospholipid compositions, the lipid molar fraction compositions of different lipids at the inner membrane surface are summarised in Table . The total number density of the negatively charged DPPS could be varied while the molar ratio between DPPC and DPPE was kept constant in order to evaluate how DPPS was involved in the RhoA-PBR and RhoA-PBRQ adsorption. The average area per lipid was set to 0.645 nm2.
Table I. Homogeneous bare membrane compositions for membranes A, B, C and D
The molecular theory was formulated by writing the total free energy of the system as a function of the 3-dimensional spatial distribution of molecules making up the system and the probabilities for different lipid and protein conformations. The relevant contribution to the total free energy of the system included the following terms:1
where T was the system temperature, S conf,lip was the conformational entropy of the different lipid molecules, S mix,lip was the translational (mixing) entropy of the lipid molecules, S mix was the translational (mixing) entropy of the free species (water molecules, anions and cations), U int,lip accounted for the internal energy of the lipid chain conformation, U elec was the total electrostatic energy that arises from the charged species, U vdW was the intermolecular total van der Waals attraction energy, U B was the self-energy of the free ions and S conf,PBR represented the conformational entropy of the protein being adsorbed. The input required to solve the set of equations were the set of conformations for each lipid type and protein molecules, the salt concentration of the solution in contact with the membrane and the lipid composition of the bilayer.
Results
Phospholipid reorganisation in MP membrane
Cultured human breast cancer cells continuously generated MPs. Individual MPs were identified as vesicular structures on the surface of viable cells. They differed from other cellular structures such as the lamellipodia and blebs. The MPs stained strongly using Duramycin-based fluorescent probes, which bound PE with high affinity and specificity. In confocal micrographs, the vesicular structure was apparent, where the staining was along the membrane surface of MPs and was not internalised (A–C). This staining profile was indicative of an accessible source of PE, presumably exposed on the MP surface. In contrast, neither the leading edge, where there was active lamellipodia formation (arrow heads), nor the retracting end of the cell had a general staining for PE. The difference suggested that although there were active cytoskeletal and membrane reorganisation in these regions, PE externalisation took place only under well-defined conditions. With time, the MPs were shed into the culture media, where they retained surface PE and were stained positive by Duramycin (D–F).
Fig. 1. PE staining at the surface of MPs. A–C, a cell-associated MP is stained positive for surface PE using Duramycin. Scale bars represent 5 µm. D–F, isolated MPs are consistently stained positive for surface PE. Scale bars represent 3 µm.
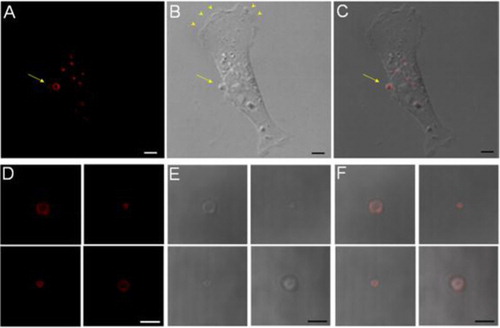
We used a series of endogenously expressed probes to investigate the distribution of various phospholipids at the inner surface of MP and PM. The endogenous PS probe, consisting of the C2 domain of lactadherin and GFP (Lact-C2-GFP), strongly stained the inner surface of MP membrane (A). The signal intensity from Lact-C2-GFP in MPs was greater than that at the PM with a ratio of 4.43±3.16 (n=35). Mean fluorescent intensity data from individual vesicles versus the PM were collected and are shown in Supplementary file. This was indicative of a greater density of PS, accessible at a higher abundance as binding targets for Lact-C2-GFP. Staining of PS at the inner membrane of MPs by Lact-C2-GFP consistently colocalised with PE staining by Duramycin from the outer surface (A–C). Quantitatively, Lact-C2-GFP and Duramycin co-registered at a rate of 92.11% (35 out of 38 MPs). In contrast, endogenously expressed probes for PIP2 and PM had only partial colocalisation with Duramycin staining in MPs, at 38.64% (17 out of 44 MPs) and 44.23% (23 out of 52 MPs), respectively (D–I). The rates of colocalisation between surface PE and PS, PIP2 or PM are summarised in J. These differences suggested that PS had a greater presence in the inner membrane of MPs in a more selective fashion, whereas the presence of other phospholipids was less consistent.
Fig. 2. Colocalisation of surface PE with endogenously expressed probes. A–C, a greater presence of an endogenous PS-binding probe, Lact-C2-GFP, at the inner leaflet of MP membrane is colocalised with PE staining from the outer surface (arrows). D–F, an endogenous PIP2 probe has a partial colocalisation with PE staining (arrows); but with many mismatches (arrow heads). G–I, a general probe for the PM also had a partial colocalisation in MPs, with mismatches (arrow heads). Scale bars represent 5 µm. J, a summary for rates of colocalisation between surface PE and PS, PIP2 or PM.
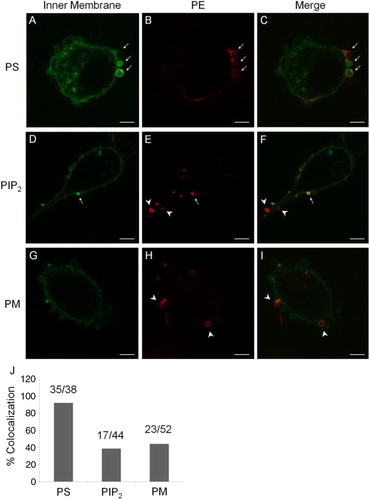
Colocalisation of small GTPases in MPs
To characterise the localisation of RhoA in MPs, we used an endogenously expressed fusion protein between the wild-type RhoA and GFP (GFP-RhoA). As shown in A–C, GFP-RhoA colocalised consistently in MPs that were stained positive for surface PE. Quantitatively, the rate of MP colocalisation for GFP-RhoA was 74.73% (68 out of 91 MPs). Based on the GFP fluorescence intensity, there was a stronger presence of GFP-RhoA along the membrane of MPs than the PM elsewhere with a ratio of 3.31±2.36 (n=68). This was indicative of a process that selectively sequestered the probe in MPs. The endogenous expression of a fusion protein between constitutively active RhoA and GFP (GFP-CA-RhoA) exhibited a similar rate of colocalisation in MPs, at 76.67% (46 out of 60 MPs) (D–F). In contrast, the substitution of positive charges in the PBR of RhoA with glutamine diminished the rates of colocalisation to 13.43% (9 out of 67 MPs) and 15.15% (10 out of 66 MPs), for GFP-RhoA-PBRQ and GFP-CA-RhoA-PBRQ, respectively (G–L). The rates of colocalisation between PE-positive vesicles and different RhoA constructs are summarised in M.
Fig. 3. Colocalisation of RhoA probes in MPs. A–C, an intense presence of GFP-RhoA in PE-positive MPs (arrows). D–F, colocalisation of constitutively active GFP-RhoA in PE-positive MPs (arrows). G–I, substitution of cationic residues in the PBR of GFP-RhoA with Gln (PBRQ) abolished MP localisation and resulted in a mismatch with MPs (arrow heads). J–L, the PBRQ mutant of GFP-CA-RhoA has poor colocalisation in MPs. Scale bars represent 5 µm. M, a summary for rates of colocalisation between surface PE and RhoA derivatives.
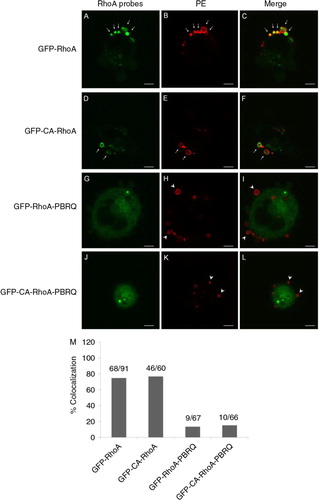
The above findings on RhoA were validated using immunocytochemisty that probed directly for native RhoA. Cells incubated with biotinylated Duramycin for MP binding were fixed and permeabilised, and were stained using an anti-human RhoA antibody. The presence of native RhoA in MPs was colocalised with MPs that were positive for membrane PE at a rate of 80.04% (33 out of 41 MPs). The signal intensity for RhoA staining was greater in MPs than along the PM, with a ratio of 1.85±0.70 (n=33). A typical example of RhoA colocalisation in a PE-positive MP is shown in A–C. As a second line of validation, MPs were harvested by centrifugation and their protein content, versus that of the total cell lysate, was probed for the presence of RhoA by Western blotting. As demonstrated in D, there was a significantly elevated RhoA level in MPs compared with cell lysate. This result further supported the selective sequestration of RhoA in MPs.
Fig. 4. Colocalisation of native RhoA in MPs. A–C, the presence of native RhoA in MPs, as detected by immunocytochemistry, is colocalised with PE staining from the outer surface. D, MPs harvested from the culture media contained relatively high levels of native RhoA compared to total cell lysate, as detected using Western blotting. Scale bars represent 5 µm.
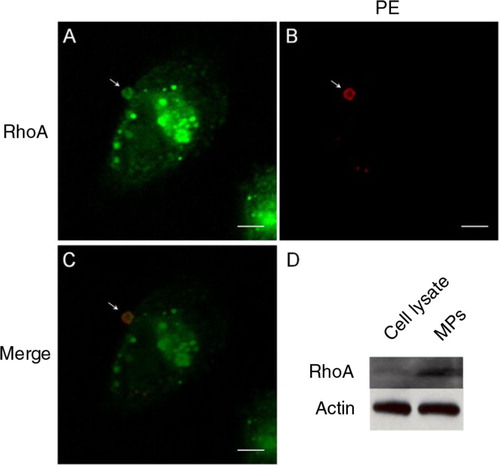
Electrostatic-dependent protein localisation in MPs
To test the hypothesis that an electrostatic-dependent mechanism contributes to the selective packaging of positively charged protein factors in MPs, we constructed a chimeric probe that consisted of a GFP fused with the C-terminal of RhoA which contains the PBR (GFP-PBR). A control was constructed with the cationic residues in the PBR substituted with glutamines (GFP-PBRQ). When GFP-PBR was endogenously expressed, it had a substantially greater rate of colocalisation with PE-positive MPs at 41.42% (70 out of 169 MPs) (A–C) compared to the chimer with mutated PBR, GFP-PBRQ, which had few incidences of colocalisation in MPs at 12.71% (15 out of 118 MPs; D–F). The rates of colocalisation between PE surface staining and GFP-PBR or GFP-BPRQ are summarised in G.
Fig. 5. Electrostatic-dependent localisation of protein factors in MPs. A–C, the colocalisation of a general probe consisting of EGFP and the C-terminal PBR of RhoA (EGFP-PBR) in MPs (arrows). D–F, Gln substitution of basic residues abolished the probe localisation in MPs (arrow heads). Scale bars represent 5 µm. G, a summary for rates of colocalisation between surface PE and GFP chimers carrying the PBR or PBRQ.
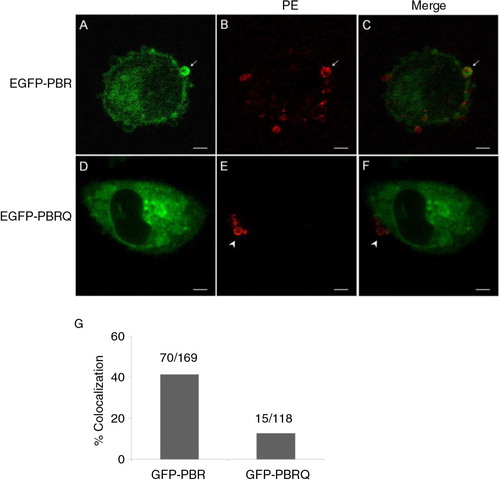
Theoretical calculations of PBR-membrane interactions
In silico thermodynamic calculations supported, in a quantitative fashion, a positive enhancement of PBR-dependent protein binding to PS-rich membrane surface. For the positively charged RhoA-PBR, the relative free energy of adsorption is greater (more negative) as the local density of PS increases (Table ), whereas this trend does not hold true for the negative control, PBRQ (Table ). We examined thermodynamic contributions from both electrostatic and non-electrostatic components. As the concentration of PS increases in the membrane composition, there is a stronger electrostatic component to the adsorption free energy for the positively charged PBR. It is important to emphasise that the electrostatic contribution includes charge–charge interactions, counter ion release and the recruitment of an optimal phospholipid rearrangement underneath the adsorbed protein (see below). At the same time, the non-electrostatic free energy becomes more negative showing the coupling that exists among all degrees of freedom. The main gain in non-electrostatic free energy is in the recruitment of lipids with smaller head-groups in order to optimise the interactions between the PS lipids and the charged protein. This behaviour is a result from the release of steric repulsions in the packing of lipid head groups near the region where the protein is located. For the non-charged protein, the (slightly) positive values for the relative adsorption free energies do not show a significant dependence on the amount of negatively charged PS in the underlying membrane. Namely, there is no net gain in the relative free energy when RhoA-PBRQ is adsorbed to a lipid membrane that contains negatively charged PS.
Table II. Calculated relative free energy of adsorption of PBR on membranes A, B, C and D
Table III. Calculated relative free energy of adsorption of PBRQ membranes A, B, C and D
The molecular theory output contains both thermodynamic information and changes in the structure of lipid layer upon protein adsorption. The predictions indicate that the adsorption of PBR on membrane surface resulted in a lateral reorganisation of phospholipids at the vicinity of the adsorbed protein. displays the maps of net changes in phospholipid density when the positively charged RhoA-PBR is adsorbed onto the bilayer. For the adsorption of RhoA-PBR on PS-containing membrane, there is a lateral rearrangement of local PS molecules near the positively charged protein (A). The gain in the overall free energy, by bringing into close proximity the positively charged protein and negatively charged lipids, is substantially greater than the loss in entropy that results from the local congregation of PS. The lateral reorganisation of PS was accompanied with a depletion of PC and PE near the adsorbed Rho-A-PBR (A). The reduction of PC and PE molecules allows for more space for PS molecules. In contrast, B shows that there is no effective attraction between the uncharged RhoA-PBRQ protein segment and the lipid membrane. This is consistent with the weak dependence of the RhoA-PBRQ free energy of adsorption on the PS membrane composition.
Fig. 6. Molecular theory predictions of PBR-membrane interactions. A, the presence of DPPS in the membrane substantially enhanced PBR adsorption. The PBR is represented as a string of beads, where basic residues are purple, neutral green and Cysteine yellow. The adsorption of PBR induced a lateral rearrangement of DPPS to congregate in the vicinity of the peptide, which was accompanied with a reduction of local PE and PC levels. The colour bar on the right side represents the fold change, where blue indicates a 2 fold enhancement and red approaches an absence of enhancement. B, the mutant PBRQ resulted in minimal re-organisation in all phospholipids.
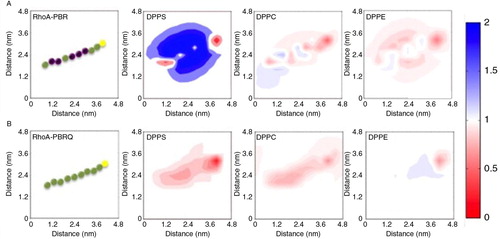
Discussion
Cancer cell-derived MPs constitute a form of cell-cell communication that is distinct from soluble secreted factors. The MPs contain signalling factors, and are known to modulate cell survival, differentiation, cancer invasion, metastasis and evasion of immune surveillance. A higher abundance of biological factors in MPs is likely to contribute to a greater signalling capacity. Emerging evidence indicates that cargo contents of MPs are recruited in a selective fashion as opposed to a passive replica of host cell components (Citation18). However, the recruiting mechanism for selected signalling factors to MPs remains not fully characterised.
The PM is centrally positioned in many important signalling pathways; MP cargos of membrane-interacting factors are instrumental in eliciting responses in target cells. A membrane-based recruitment process involving electrostatic attraction offers an efficient mechanism in that it can afford to attract a wide range of factors without necessitating cognate binding or transport interactions. Small GTPase RhoA has been shown to localise in cancer cell-derived MPs (Citation19, Citation20). The presence of RhoA is essential for triggering signalling pathways that lead to the formation of MPs. Rho GTPases play important roles in a broad range of cellular activities from cytoskeletal rearrangement to migration. Recent evidence indicates Rho GTPase activities are linked to mitochondrial glutaminase activation, thus energy metabolism in cancer cell (Citation21). It is conceivable that the horizontal transduction of Rho GTPases by way of extracellular microvesicles will have an effect on tumour cell transformation and microenvironment. An enrichment of Rho GTPases in cancer cell-derived MPs therefore will not only facilitate microvesicle biogenesis but also potentiate the oncogenic impact of MPs on recipient cells.
Data from the current study indicated that the recruitment of Rho GTPases to the inner membrane of MPs was critically dependent on the presence of PBRs. The MP localisation of a GFP-PBR probe substantiated this finding, and implicated a more general recruitment mechanism which helped attract protein factors to MPs via electrostatic interactions. This may shed light on the presence of signalling factors beyond the Rho GTPases. Other factors that are known to colocalise in MPs include the morphogen Sonic Hedgehog (Shh) (Citation22), chemokine (C-C motif) ligand 5 (CCL5) (Citation23) and EGFR (Citation24). A common feature for these signalling factors is that they are membrane-interacting proteins with PBRs. The presence of cationic features in these proteins can make them more responsive towards anionic surfaces electrostatically.
Both experimental data and theoretical predictions indicate that the localisation of Rho GTPases in MPs is attributed at least in part to changes in electrostatic potential along the inner membrane surface. The colocalisation of molecular probes for different phospholipid species suggests that the recruitment of PS is the predominant contributing factor but not the PIs, even though the latter are more strongly anionic. This difference may be explained by the regulatory processes that govern local concentrations of PIs by kinases, phosphatases and lipases; whereas, the regulation of PS is attainable via lateral diffusion but to a less degree chemical modulation. According to the theoretical predictions, the electrostatic attraction of positively charged protein factors to local membrane surface helps recruit a greater presence of PS through lateral diffusion. As such, by combining experimental and theoretic data, the current findings are consistent with a mutual attraction among anionic membrane phospholipids and cationic protein factors at the membrane interface. However, it remains to be established whether this type of interactions are sufficient to cause a greater scale of PS enrichment as detected by endogenous probes. The mutual attraction is likely to have contributed to a self-sustained recruitment force that helps package protein factors in a PBR-dependent fashion.
Accumulating evidence suggests a regulatory role of membrane phospholipid redistribution-medicated electrostatic switch. Congregated PS distribution was observed in Saccharomyces cerevisiae, where it mediates cell polarity by maintaining Cdc42 localisation (Citation4). This process was not associated with a lateral redistribution of PIs (Citation4, Citation5). The cyclic on-and-off association of Cdc42 with PM is regulated by the inward translocation of PE, carried out by P4 ATPases Dnf1/Dnf2 with Cdc50 homologue Lem3 as a cofactor (Citation5). It was proposed that the concentration of PE confers a buffering capacity that controls the local density of PS, thus anionic strength. The membrane association of yeast Cdc42 was further demonstrated using artificial membrane containing a high density of PS, whereas this association was diminished when PE concentration was elevated as a dilution factor for PS (Citation5). The electrostatic-dependent localisation of cytosolic proteins was demonstrated using a series of endogenously expressed probes with variable cationic surface charges (Citation25). The study provides yet another layer of evidence on the modulation of protein–membrane interactions via electrostatic density. In the mammalian system, PE externalisation was observed at the cleavage furrow and is essential for the completion of cytokinesis (Citation6).
In cancer cell-derived MPs, there has been no direct evidence that captures the process of trans-bilayer movement of phospholipids, such as PE, for mediating local membrane electrostatic density. The interpretation of current findings was based on correlative and partially causal data which offered a plausible explanation on PS congregation in the inner leaflet and its association with PE externalisation. This interpretation was also derived in light of similar phenomena observed in the yeast. Nonetheless, the underlying mechanism that mediates local phospholipid flip-flop activities remains elusive. Known factors that can mediate membrane phospholipid asymmetry include P4 ATPases, which catalyse the inward translocation of phospholipids; floppases, which transport phospholipids towards the outer leaflet; and scramblases, which randomise phospholipid distribution across the 2 leaflets. Among these mediators, MDR1 was thought to be an attractive candidate initially because it is known to localise in MP membrane and that PE analogues had been shown to be translocated across the membrane bilayer as substrates for MDR1 (Citation26). However, ABC transporters act as exporters instead of translocases (Citation27). A second possibility is the local down-regulation of P4 ATPase(s) which may be responsible for maintaining the asymmetric distribution of aminophospholipids, in particular PE. The inactivation of Tat-5, a PE-specific P4 ATPase in C. elegans, resulted in elevated formation of extracellular vesicles (Citation7). However, among the 14 known P4 ATPases in humans, PE specificity has not been reported to this day. A third scenario is the local activation of scramblases. However, a non-discriminative redistribution of phospholipids will result in the equilibrium of both PE and PS. This scenario is incompatible with our data that PE is consistently present at MP outer surface but not PS. More than 40% of MPs derived from breast cancer cells were PS-negative, whereas they are uniformly PE-positive (Citation8).
In conclusion, we present data on the redistribution of membrane phospholipids in MPs, which alludes to a potential recruitment mechanism where electrostatic interactions may contribute to the selective packaging of signalling protein factors in cancer cell-derived MPs.
Conflict of interest and funding
The authors declare there are no competing financial interests in relation to the work described.
Supplementary material
Download PDF (196.3 KB)Acknowledgement
Financial support from the National Institute of Health (5R01HL102085) and the National Science Foundation (CBET-1264696) is gratefully appreciated.
Notes
To access the supplementary material to this article, please see Supplementary files under Article Tools online.
References
- Muralidharan-Chari V, Clancy JW, Sedgwick A, D'Souza-Schorey C. Microvesicles: mediators of extracellular communication during cancer progression. J Cell Sci. 2010; 123: 1603–11.
- D'Souza-Schorey C, Clancy JW. Tumor-derived microvesicles: shedding light on novel microenvironment modulators and prospective cancer biomarkers. Genes Dev. 2012; 26: 1287–99.
- van Meer G, Voelker DR, Feigenson GW. Membrane lipids: where they are and how they behave. Nat Rev Mol Cell Biol. 2008; 9: 112–24.
- Fairn GD, Hermansson M, Somerharju P, Grinstein S. Phosphatidylserine is polarized and required for proper Cdc42 localization and for development of cell polarity. Nat Cell Biol. 2011; 13: 1424–30.
- Das A, Slaughter BD, Unruh JR, Bradford WD, Alexander R, Rubinstein B et al. Flippase-mediated phospholipid asymmetry promotes fast Cdc42 recycling in dynamic maintenance of cell polarity. Nat Cell Biol. 2012; 14: 304–10.
- Emoto K, Inadome H, Kanaho Y, Narumiya S, Umeda M. Local change in phospholipid composition at the cleavage furrow is essential for completion of cytokinesis. J Biol Chem. 2005; 280: 37901–7.
- Wehman AM, Poggioli C, Schweinsberg P, Grant BD, Nance J. The P4-ATPase TAT-5 inhibits the budding of extracellular vesicles in C. elegans embryos. Curr Biol. 2011; 21: 1951–9.
- Larson MC, Woodliff JE, Hillery CA, Kearl TJ, Zhao M. Phosphatidylethanolamine is externalized at the surface of microparticles. Biochim Biophys Acta. 2012; 1821: 1501–7.
- Berg TJ, Gastonguay AJ, Lorimer EL, Kuhnmuench JR, Li R, Fields AP et al. Splice variants of SmgGDS control small GTPase prenylation and membrane localization. J Biol Chem. 2010; 285: 35255–66.
- Elliott R, Katsov K, Schick M, Szleifer I. Phase separation of saturated and mono-unsaturated lipids as determined from a microscopic model. J Chem Phys. 2005; 122: 44904.
- Grillo DM, Szleifer I, de la Cruz MO. Molecular modeling of domain formation upon protein adsorption in lipid bilayers. Biophys J. 2011; 100: 333.
- Uline MJ, Longo GS, Schick M, Szleifer I. Calculating partition coefficients of chain anchors in liquid-ordered and liquid-disordered phases. Biophys J. 2010; 98: 1883–92.
- Pogodin S, Baulin VA. Coarse-grained models of phospholipid membranes within the single chain mean field theory. Soft Matter. 2010; 6: 2216–26.
- Khelashvili G, Harries D, Weinstein H. Modeling membrane deformations and lipid demixing upon protein-membrane interaction: the BAR dimer adsorption. Biophys J. 2009; 97: 1626–35.
- Tagliazucchi M, Peleg O, Kroger M, Rabin Y, Szleifer I. Effect of charge, hydrophobicity, and sequence of nucleoporins on the translocation of model particles through the nuclear pore complex. Proc Natl Acad Sci U S A. 2013; 110: 3363–8.
- Tagliazucchi M, de la Cruz MO, Szleifer I. Self-organization of grafted polyelectrolyte layers via the coupling of chemical equilibrium and physical interactions. Proc Natl Acad Sci U S A. 2010; 107: 5300–5.
- Nap R, Gong P, Szleifer I. Weak polyelectrolytes tethered to surfaces: effect of geometry, acid-base equilibrium and electrical permittivity. J Polym Sci Pol Phys. 2006; 44: 2638–62.
- Lee TH, D'Asti E, Magnus N, Al-Nedawi K, Meehan B, Rak J. Microvesicles as mediators of intercellular communication in cancer – the emerging science of cellular ‘debris’. Semin Immunopathol. 2011; 33: 455–67.
- Antonyak MA, Wilson KF, Cerione RA. R(h)oads to microvesicles. Small GTPases. 2012; 3: 219–24.
- Li B, Antonyak MA, Zhang J, Cerione RA. RhoA triggers a specific signaling pathway that generates transforming microvesicles in cancer cells. Oncogene. 2012; 31: 4740–9.
- Wilson KF, Erickson JW, Antonyak MA, Cerione RA. Rho GTPases and their roles in cancer metabolism. Trends Mol Med. 2013; 19: 74–82.
- Soleti R, Benameur T, Porro C, Panaro MA, Andriantsitohaina R, Martínez MC. Microparticles harboring Sonic Hedgehog promote angiogenesis through the upregulation of adhesion proteins and proangiogenic factors. Carcinogenesis. 2009; 30: 580–8.
- Mack M, Kleinschmidt A, Brühl H, Klier C, Nelson PJ, Cihak J et al. Transfer of the chemokine receptor CCR5 between cells by membrane-derived microparticles: a mechanism for cellular human immunodeficiency virus 1 infection. Nat Med. 2000; 6: 769–75.
- Al-Nedawi K, Meehan B, Micallef J, Lhotak V, May L, Guha A et al. Intercellular transfer of the oncogenic receptor EGFRvIII by microvesicles derived from tumour cells. Nat Cell Biol. 2008; 10: 619–24.
- Yeung T, Gilbert GE, Shi J, Silvius J, Kapus A, Grinstein S. Membrane phosphatidylserine regulates surface charge and protein localization. Science. 2008; 319: 210–13.
- Bosch I, Dunussi-Joannopoulos K, Wu RL, Furlong ST, Croop J. Phosphatidylcholine and phosphatidylethanolamine behave as substrates of the human MDR1 P-glycoprotein. Biochemistry. 1997; 36: 5685–94.
- van Meer G. Dynamic transbilayer lipid asymmetry. Cold Spring Harb Perspect Biol. 2011; 3: 1–11.