Abstract
Hydrophobic interaction chromatography (HIC) is commonly used as a polishing step in monoclonal antibody purification processes. HIC offers an orthogonal selectivity to ion exchange chromatography and can be an effective step for aggregate clearance and host cell protein reduction. HIC, however, suffers from the limitation of use of high concentrations of kosmotropic salts to achieve the desired separation. These salts often pose a disposal concern in manufacturing facilities and at times can cause precipitation of the product. Here, we report an unconventional way of operating HIC in the flowthrough (FT) mode with no kosmotropic salt in the mobile phase. A very hydrophobic resin is selected as the stationary phase and the pH of the mobile phase is modulated to achieve the required selectivity. Under the pH conditions tested (pH 6.0 and below), antibodies typically become positively charged, which has an effect on its polarity and overall surface hydrophobicity. Optimum pH conditions were chosen under which the antibody product of interest flowed through while impurities such as aggregates and host cell proteins bound to the column. This strategy was tested with a panel of antibodies with varying pI and surface hydrophobicity. Performance was comparable to that observed using conventional HIC conditions with high salt.
Introduction
Hydrophobic interaction chromatography (HIC) occupies a unique niche as a polishing step in many monoclonal antibody (mAb) purification processes.Citation1,Citation2 This mode of chromatography is particularly useful for aggregate removal, and it provides good clearance of other process-related impurities such as host cell protein (HCP), leached Protein A and endogenous viruses.Citation3-Citation6 HIC is based on interactions between hydrophobic (aliphatic or aromatic) ligands on the stationary phase with hydrophobic patches on the surface of proteins.Citation7 Interactions of proteins on HIC are usually promoted by kosmotropic salts, e.g., ammonium sulfate, sodium citrate, potassium phosphate.Citation8 Kosmotropic salts interact with water molecules to reduce solvation of protein molecules in solution and expose their hydrophobic patches to promote binding.Citation9 Elution is usually facilitated by decreasing salt concentration or by use of organic mobile phase modifiers.
Despite its orthogonal selectivity, the use of HIC in any purification process presents two primary challenges. In general, binding capacity has been traditionally limited on HIC, especially in comparison to ion exchange chromatography (IEX).Citation10,Citation11 Resin vendors have lately tried to optimize the pore size and ligand density in an effort to maximize capacity;Citation12 however, 10% breakthrough capacities of > 40 mg/mL of resin have not yet been reported.Citation13 To circumvent this issue, HIC is sometimes used in the flowthrough mode in which the product of interest flows while the more hydrophobic impurities remain bound to the column. This strategy has been particularly popular as a polishing step in antibody processes since aggregates are usually more highly retained on HIC.Citation14 Second, the use of high concentrations of salts is highly undesirable in any manufacturing process because it can cause corrosion of stainless steel tanks. Due to municipal waste water concerns, it is very expensive to dispose of ammonium sulfate, the most commonly used kosmotropic salt.Citation15 In addition, the presence of salt in the load material, elution pool or the FT pool from the HIC step also complicates sample manipulation and requires significant dilution, or an ultrafiltration/ diafiltration unit operation, between processing steps.Citation13
Efforts to operate HIC under reduced or no-salt conditions have been reported. Arakawa and researchersCitation16,Citation17 tried to use arginine to promote binding and facilitate elution in HIC systems. Recently, GagnonCitation18 reported the use of glycine in HIC systems to keep conductivities low. Kato et al.Citation19 used HIC at low salt concentration for capture of mAbs using a critical hydrophobicity approach, but with limited success.
Here, we report a novel use of HIC in the flowthrough mode with no kosmotropic salt in the mobile phase. Instead of the addition of salt, the pH of the mobile phase was modulated to alter the surface charge of the protein, and thereby influence selectivity. The effect of pH on retention in HIC is usually unpredictable and thus pH is not frequently studied as a parameter during HIC optimization. In practice, however, it can influence protein retention by titrating charged patches close to the hydrophobic patches on the protein surface.Citation20 For our examination of the effects of pH adjustment, we selected a very hydrophobic resin to promote maximum interaction with the stationary phase under no-salt conditions.
Results
Four mAbs (mAbs A-D) with varying pIs (~6.5−8.7) and surface hydrophobicity were used in this study. The antibodies had a HIC FT step in their manufacturing process that primarily served to reduce aggregates and HCPs. Ammonium sulfate was used as the kosmotropic salt to achieve the desired selectivity; the concentration selected in the process was dependent on the hydrophobicity of the molecule and the separation desired. The ammonium sulfate concentration needed for each molecule and the dilution that was required to prepare the load sample for its respective HIC (Phenyl Sepharose Fast Flow [FF] High Substitution [HS]) FT step are shown in . The aim of this study was to devise an alternative HIC FT step using no-salt conditions that would be comparable in process performance to the existing HIC FT step, which served as the control.
Table 1. Ammonium sulfate concentrations used in the control HIC (Phenyl Sepharose) FT processes and corresponding dilutions with concentrated salt solution required to achieve the required ammonium sulfate concentration
Resin selection
The first step in the optimization process was to select a resin that was more hydrophobic than the Phenyl Sepharose FF HS resin used in the existing process. In the FT mode, only a more hydrophobic resin than the control resin has the potential of achieving the same separation under reduced salt conditions. A lesser hydrophobic resin would require even higher salt concentration to provide the same selectivity. To compare the hydrophobicity of various resins on an even basis, linear retention of lysozyme in a decreasing salt (ammonium sulfate) gradient was determined on commonly used commercial HIC resins. More hydrophobic ligands, e.g., phenyl, butyl, hexyl, octyl, were selected for this experiment, and less hydrophobic ligands such as ether and PPG were excluded. The resins chosen for screening were Phenyl Sepharose FF HS (control resin), Capto Phenyl HS, Butyl Sepharose 4FF and Octyl Sepharose 4FF from GE Healthcare, and Phenyl Toyopearl, Butyl Toyopearl and Hexyl Toyopearl from Tosoh.
The linear retention data on all of these resins is shown in . Phenyl Sepharose FF HS was actually more hydrophobic than most other resins. The only resin that was more hydrophobic than the control resin was Hexyl Toyopearl, and hence this resin was selected for further optimization. Hexyl Toyopearl also offers the advantage of a rigid polymeric backbone and allows faster flow rate and ease of packing at larger scale. Interestingly, Hexyl Toyopearl has traditionally not been selected for bind and elute applications due to overly strong antibody-resin interactions leading to low product recovery.Citation13
Process optimization
To determine the pH of the mobile phase needed for the FT step, pH gradients were run initially under analytical conditions with all four antibodies on the Hexyl Toyopearl resin. A pH range of 6.0−3.5 was chosen for the gradient because most of the antibodies used in the study were not very stable beyond this range. The pH at which each mAb eluted in the gradient is shown in and the exact values are listed in . MAbs B and D were practically unretained and hence eluted at pH 6.0, the starting point of the gradient ().
Table 2. Elution pH at peak maxima in a decreasing pH gradient on Hexyl Toyopearl data
The pH values listed in was used as the starting point for further optimization of the preparative flowthrough conditions. The amount of protein loaded during the preparative experiments was kept the same as the control process for an unbiased comparison. Higher pHs caused the antibody monomer to bind more strongly, resulting in lower step yields, while lower pHs caused the high molecular weight (HMW) species to flow through along with the monomer. The goal was to find the optimum pH that gave the best compromise between recovery and HMW clearance. The mobile phase pH was optimized for each molecule to give comparable performance as its respective control step in terms of step yield and impurity (HMW and HCP) clearance (detailed optimization data not shown). shows a representative chromatogram for mAb B from the no-salt HIC flowthrough step. The final conditions developed for the new HIC FT step for each antibody are listed in . A comparison of the data in and , indicates that the final optimum pH conditions were fairly close to those obtained from the analytical pH gradient experiments. Hence, this can be used as quick method development tool for this process step. It is also interesting to note that mAbs B and D had the same optimum pH (pH 6.0) despite having pIs at the two ends of the range (8.7 vs. 6.5). This was probably due to the fact that the two mAbs were significantly different in their surface hydrophobicity as determined by linear retention on the control HIC resin (). mAb B is less hydrophobic than mAb D (), which likely counteracted the effect of higher pI. Thus, it can be said that the optimum pH needed by each molecule was influenced by both its pI and surface hydrophobicity. As shown in , the process data (step recovery and impurity clearance) from the two HIC steps (no-salt and high salt control process) indicates that performance comparable to the control was seen in all cases.
Table 3. Process performance comparison between high-salt and no-salt HIC FT step for each antibody
Figure 4. Elution salt concentration of mAb B and D on a decreasing ammonium sulfate gradient using Phenyl Toyopearl resin (Lower elution salt concentration implies greater hydrophobicity).
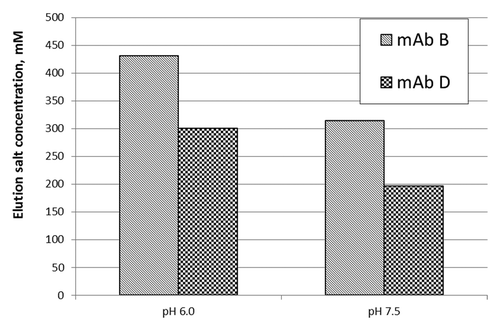
Further optimization studies were conducted with mAb B to evaluate the effect of column loading on step performance. plots step yield and HMW level of the FT pool as a function of column loading on the Hexyl resin. Only HMW was monitored because it was the critical impurity that needed to be removed by this step. Protein A eluate with a higher HMW % was used for this study to test the worst-case scenario; hence, the HMW levels here are slightly higher than that reported in . As seen in , both yield and HMW levels increased as a function of column loading. This is typical for any flow-through step where the optimum column loading is selected based on best compromise between yield and desired HMW level. The rate of increase in this case was found to be similar to what had been seen with the historic high salt HIC step. An average loading of ~100 g/L was chosen for this process to consistently meet target HMW level of < 1%.
After finalizing the mobile phase conditions and column loading, a resin lot-to-lot variability study was also completed to ensure process robustness at manufacturing scale (). This was considered important because resin hydrophobicity was a major contributor to the selectivity of this step. Three lots of Hexyl resin spanning the manufacturer’s specification range was chosen for this study. Since the HIC step was designed to be used as the 2nd polishing step, eluate from the 1st polishing step was used as load for this study. All experiments were performed at 100 mg/ ml resin loading. summarizes the yield and product quality data and shows the consistent performance across all three resin lots.
Table 4. Resin lot-to-lot variability study
Discussion
The results shown here demonstrate a new way of utilizing the selective power of a HIC step without using high salt solutions. Operating an HIC step in the absence of kosmotropic salts in the mobile phase can have substantial implications for large scale protein purification processes. For example, the method eliminates the need for the addition of relatively high concentrations of ammonium sulfate or other kosmotropic salts to the mobile phase prior to the HIC step and avoids the associated dilution of the feed stream. In our case, this enabled the scale up of a highly productive (high titer) mAb production process in an existing facility by overcoming tank volume limitations. Minimizing pool volumes also had an economic impact as it helped to significantly reduce the size of the costly viral filter that followed the HIC step. Furthermore, removing ammonium sulfate from the manufacturing process helped reduce disposal costs and was considered more compatible with environmental considerations. While the proof-of-concept described here was demonstrated with mAbs and Hexyl Toyopearl resin and is particularly useful for high titer antibody processes, in theory the concept can be extended to any other protein and resin of similar hydrophobicity.
Materials and Methods
Materials
All mAbs used in this study were produced internally at Biogen Idec in a CHO cell line. MAbs A-D were IgG1s with isoelectric points of ~7.2, 8.7, 7.4, and 6.5, respectively. Model protein lysozyme was purchased from Sigma. Agarose-based resins such as Phenyl Sepharose HS, Capto Phenyl HS, Butyl Sepharose 4FF and Octyl Sepharose 4FF were obtained from GE Healthcare. Methacrylate-based HIC resins such as Phenyl Toyopearl 650M, Butyl Toyopearl 650M, and Hexyl Toyopearl 650C were obtained from Tosoh Bioscience. TSK gel G3000 SWXL column (7.8 mm × 300 mm) used for SEC analysis was purchased from Tosoh Bioscience. All chemicals and salts were purchased from JT Baker.
Equipment
All chromatographic experiments were performed on AKTA Explorer chromatographic systems from GE Healthcare. HPLC analysis was performed in a Waters HPLC e2695 Separation Module. Absorbance of protein samples was measured using a Lambda 25 UV/VIS spectrophotometer from Perkin Elmer.
Protein retention experiments
Linear retention data of lysozyme on the various HIC resins was obtained from linear gradient experiments using pulse injection (0.1 mL of protein at ~5 mg/ml concentration) using a 0.66 cm D × 10 cm L column. A decreasing gradient of salt (ammonium sulfate) was run from 1.5 M to 0 M over 15 column volumes in a phosphate buffer system at pH 7.0.
The elution pH of the various antibodies on Hexyl Toyopearl was obtained from linear gradient experiments using pulse injection (0.5 mL of protein at ~5 mg/ml concentration) using a 0.66 cm D × 10 cm L column. A decreasing gradient of pH was run from pH 6.0 to 3.5 over 15 column volumes in a 10 mM citrate (conductivity ~2−3 ms/cm) buffer system. The elution pH at peak maxima was calculated from the gradient and further verified from the effluent pH trace obtained from the online Monitor pH/C-900 unit that is part of the AKTA system.
Salt gradient experiments with mAbs B and D were also performed in a similar manner on the Phenyl Sepharose resin. A decreasing gradient of ammonium sulfate was run from 1.5 to 0 M ammonium sulfate at pHs 6 and 7 over 10 column volumes. The elution salt concentration at peak maxima was calculated from the gradient.
Preparative purification experiments
The HIC preparative experiments were performed in the flowthrough mode. A 1 cm D × 20 cm L column was used for each experiment. The column was first equilibrated with 3 column volumes of the equilibration buffer. The mobile phase salt concentration and pH of that buffer was specific to the protein and resin combination, as explained in the Results section. The column was then loaded with a specific amount of protein as mentioned above. The flowthrough peak collection was started as the UV started to rise and the product was chased with the equilibration buffer. The column was cleaned with 3−5 column volumes of water and sanitized with 0.5N NaOH. A residence time of 6 min was used throughout the process.
Analytical techniques
HMW levels in samples were measured by analytical Size Exclusion Chromatography (SEC) using TSK gel G3000 SWXL column. A mobile phase of 100 mM NaPO4, 200 mM NaCl, pH 6.8 and a flow rate of 1 mL/min was used. Elution peaks were detected by UV absorbance at 280 nm.
HCP levels in the samples from the preparative experiments were determined using an in-house generic HCP assay comprising an ELISA-based immunoassay using electrochemiluminescent detection on the Meso Scale Discovery platform.
Acknowledgments
The authors would like to acknowledge Rae Chavez, Process Biochemistry for some experiments and the Analytical Development team within Bioprocess Development, Biogen Idec for timely analysis of all samples.
Disclosure of Potential Conflicts of Interest
No potential conflict of interest was disclosed.
References
- Shukla AA, Thömmes J. Recent advances in large-scale production of monoclonal antibodies and related proteins. Trends Biotechnol 2010; 28:253 - 61; http://dx.doi.org/10.1016/j.tibtech.2010.02.001; PMID: 20304511
- Cramer SM, Holstein MA. Downstream bioprocessing: recent advances and future promise. Curr Opin Chem Eng 2011; 1:27 - 37; http://dx.doi.org/10.1016/j.coche.2011.08.008
- McCue JT, Engel P, Ng A, Macniven R, Thömmes J. Modeling of protein monomer/aggregate purification and separation using hydrophobic interaction chromatography. Bioprocess Biosyst Eng 2008; 31:261 - 75; http://dx.doi.org/10.1007/s00449-008-0200-1; PMID: 18205016
- Shukla AA, Hubbard B, Tressel T, Guhan S, Low D. Downstream processing of monoclonal antibodies--application of platform approaches. J Chromatogr B Analyt Technol Biomed Life Sci 2007; 848:28 - 39; http://dx.doi.org/10.1016/j.jchromb.2006.09.026; PMID: 17046339
- Jiang C, Flansburg L, Ghose S, Jorjorian P, Shukla AA. Defining process design space for a hydrophobic interaction chromatography (HIC) purification step: application of quality by design (QbD) principles. Biotechnol Bioeng 2010; 107:985 - 97; http://dx.doi.org/10.1002/bit.22894; PMID: 20683852
- Lu Y, Williamson B, Gillespie R. Recent advancement in application of hydrophobic interaction chromatography for aggregate removal in industrial purification process. Curr Pharm Biotechnol 2009; 10:427 - 33; http://dx.doi.org/10.2174/138920109788488897; PMID: 19519419
- Nagrath D, Xia F, Cramer SM. Characterization and modeling of nonlinear hydrophobic interaction chromatographic systems. J Chromatogr A 2011; 1218:1219 - 26; http://dx.doi.org/10.1016/j.chroma.2010.12.111; PMID: 21255785
- Melander WR, Corradini D, Horváth CS. Salt-mediated retention of proteins in hydrophobic-interaction chromatography. Application of solvophobic theory. J Chromatogr 1984; 317:67 - 85; http://dx.doi.org/10.1016/S0021-9673(01)91648-6; PMID: 6530455
- Liu HF, Ma J, Winter C, Bayer R. Recovery and purification process development for monoclonal antibody production. MAbs 2010; 2:480 - 99; http://dx.doi.org/10.4161/mabs.2.5.12645; PMID: 20647768
- Senczuk AM, Klinke R, Arakawa T, Vedantham G, Yigzaw Y. Hydrophobic interaction chromatography in dual salt system increases protein binding capacity. Biotechnol Bioeng 2009; 103:930 - 5; http://dx.doi.org/10.1002/bit.22313; PMID: 19382248
- Urmann M, Graalfs H, Joehnck M, Jacob LR, Frech C. Cation-exchange chromatography of monoclonal antibodies: Characterization of a novel stationary phase designed for production-scale purification. MAbs 2010; 2:395 - 404; PMID: 20559022
- Roemling R, Jungbauer A. Meeting report: 8th HIC/RPC bioseparation conference. Biotechnol J 2013; Apr 26. doi: http://dx.doi.org/10.1002/biot.201300123. [Epub ahead of print].
- Chen J, Tetrault J, Ley A. Comparison of standard and new generation hydrophobic interaction chromatography resins in the monoclonal antibody purification process. J Chromatogr A 2008; 1177:272 - 81; http://dx.doi.org/10.1016/j.chroma.2007.07.083; PMID: 17709111
- Ghose S, Jin M, Liu J, Hickey J. Integrated polishing steps for monoclonal antibody purification. In: Gottschalk U, ed. Process Scale Purification of Antibodies. New York: John Wiley & Sons, 2009:145-167.
- Gagnon P. Polishing methods for monoclonal IgG purification. In: Shukla AA, Etzel MR, Gadam S, ed. Process Scale Bioseparations for the Biopharmaceutical Industry. New York: Taylor & Francis, 2006: 491-505.
- Arakawa T, Tsumoto K, Nagase K, Ejima D. The effects of arginine on protein binding and elution in hydrophobic interaction and ion-exchange chromatography. Protein Expr Purif 2007; 54:110 - 6; http://dx.doi.org/10.1016/j.pep.2007.02.010; PMID: 17408966
- Tsumoto K, Ejima D, Nagase K, Arakawa T. Arginine improves protein elution in hydrophobic interaction chromatography. The cases of human interleukin-6 and activin-A. J Chromatogr A 2007; 1154:81 - 6; http://dx.doi.org/10.1016/j.chroma.2007.02.061; PMID: 17449045
- Gagnon P. Technology trends in antibody purification. J Chromatogr A 2012; 1221:57 - 70; http://dx.doi.org/10.1016/j.chroma.2011.10.034; PMID: 22071423
- Kato Y, Nakamura K, Kitamura T, Hasegawa M, Sasaki H. Hydrophobic interaction chromatography at low salt concentration for the capture of monoclonal antibodies. J Chromatogr A 2004; 1036:45 - 50; http://dx.doi.org/10.1016/j.chroma.2004.02.009; PMID: 15139412
- Shukla AA, Yigzaw Y. Modes of Preparative Chromatography. In: Shukla AA, Etzel MR, Gadam S, ed. Process Scale Bioseparations for the Biopharmaceutical Industry. New York: Taylor & Francis, 2006: 491-505.