Figures & data
Figure 1 Application of Fe-based MOFs nanomaterials in biomedicine. Adapted from these studies.36,80,89,104,115,116,138,139,162,166.
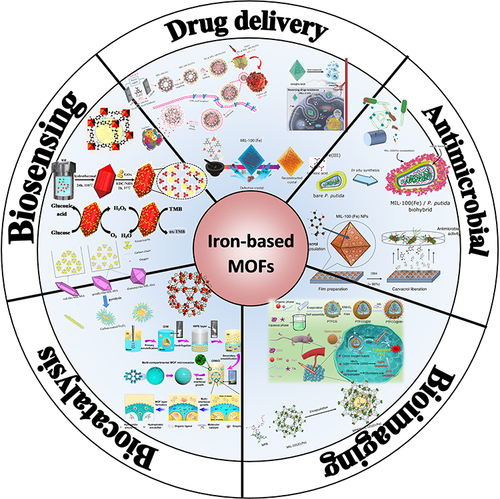
Figure 2 Application of Fe-MOF nanomaterials in biomedicine and particle size dependence relationship.
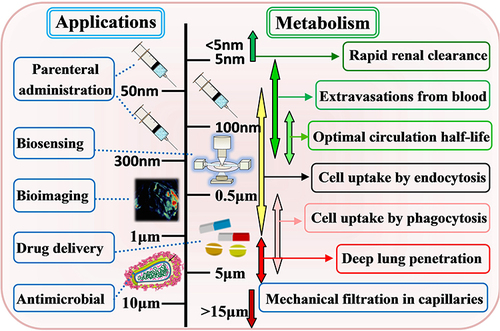
Table 1 Summarizes Achievements Related to Fe-MOFs, Solvents Used in Their Preparation, and Their Applications
Figure 4 Biocompatibility evaluation of Fe-MOFs nanomaterials: (A) Key players in iron metabolism. Reprinted from Vogt A-CS, Arsiwala T, Mohsen M, et al. On Iron Metabolism and Its Regulation. Int J Mol Sci. 2021;22:4591. Creative Commons.Citation45 (B) In vitro and in vivo models employed to assess nano materials' toxicity. Reprinted from Loret T, Rogerieux F, Trouiller B, et al. Predicting the in vivo pulmonary toxicity induced by acute exposure to poorly soluble nanomaterials by using advanced in vitro methods. Part Fibre Toxicol. 2018;15:25. Creative Commons.Citation46 (C) Evaluation of MIL-89(Fe) toxicity on embryonic zebrafish development. Reprinted from Al-Ansari DE, Al-Badr M, Zakaria ZZ, et al. Evaluation of Metal‐Organic Framework MIL-89 nanoparticles toxicity on embryonic zebrafish development. Toxicology Reports. 2022;9:951–960. Creative Common.Citation44 (D) Effects of MIL-100(Fe) on human normal liver cells (HL-7702) cells’ viability and morphology. Reprinted from Chen G, Leng X, Luo J, et al. In Vitro Toxicity Study of a Porous Iron(III) Metal‒Organic Framework. Molecules. 2019;24(7):1211. Creative Commons.Citation47
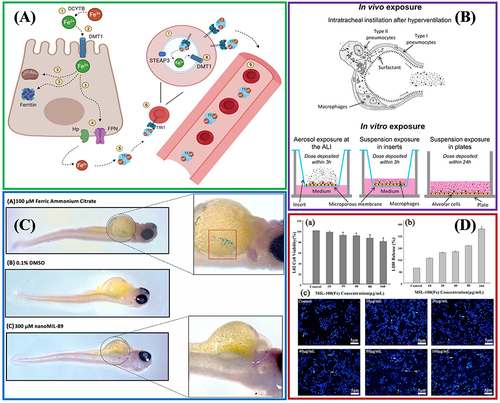
Table 2 Summary of the Drug, the MOF Type, Loading Capacity, and In Vitro Release Studies in This Review
Figure 5 Different strategies for incorporating biomedical related reagents into Fe-MOFs nanomaterials.
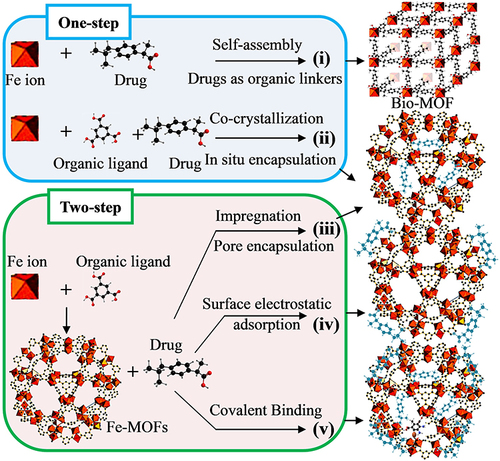
Figure 6 The practical application of Fe-MOFs in DDS: (A) Application of MIL-100 (Fe) in Guest Encapsulation. Reprinted from Souza BE, Möslein AF, Titov K, et al. Green reconstruction of MIL-100 (Fe) in water for high crystallinity and enhanced guest encapsulation. ACS Sustainable Chem Eng. 2020;8(22):8247–8255. Creative Commons.Citation36 (B) The combined formulation of the NH2-MIL-101(Fe)/d-pen and NH2-MIL-101(Fe)/CPT-11 and the anticancer mechanism. Reprinted from Ji HB, Kim CR, Min CH, et al. Fe-containing metal-organic framework with D-penicillamine for cancer-specific hydrogen peroxide generation and enhanced chemodynamic therapy. Bioeng Transl Med. 2023;8:e10477. Creative Commons.Citation77 (C) Green hydrothermal synthesis of iron based MOFs drug carriers,Citation74 (D) MOF-53 (Fe) nanoparticle embeds vancomycin drug. Lin S, Liu X, Tan L, et al. Porous Iron-Carboxylate Metal–Organic Framework: a Novel Bioplatform with Sustained Antibacterial Efficacy and Nontoxicity. ACS Appl Mater Interfaces. 2017;9(22):19248–19257. Copyright (2017), American Chemical Society.Citation82 (E) DOX@Fe-MOF nanocrystals for overcoming cancer resistance/metastasis. Reprinted from Yao XX, Chen DY, Zhao B, et al. Acid-Degradable Hydrogen-Generating Metal-Organic Framework for Overcoming Cancer Resistance/Metastasis and Off-Target Side Effects. Adv Sci. 2022;9:2101965. Creative Commons.Citation80
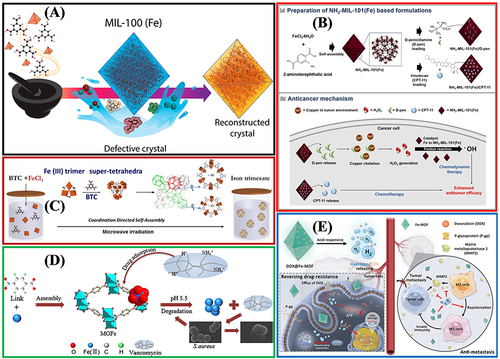
Figure 8 The practical application of Fe-MOFs in Biosensors: (A) Integrated with Glucose oxidase as a biomimetic Glucose biosensor. Reprinted from Weiqing X, Jiao L, Yan H, et al. Glucose oxidase-integrated metal–organic framework hybrids as biomimetic cascade nanozymes for ultrasensitive glucose biosensing. ACS Appl Mater Interfaces. 2019;11:25, 22096–22101. Copyright (2019) American Chemical Society.Citation89 (B) Efficient Biocatalytic System for Biosensing by Combining MIL-53(Fe) MOF-Based Nanozymes and G-Quadruplex (G4)-DNAzymes. Reprinted from Mao XX, He FN, Qiu D, et al. Efficient Biocatalytic System for Biosensing by Combining Metal- Organic Framework (MOF)-Based Nanozymes and G-Quadruplex (G4)-DNAzymes. Anal Chem. 2022;94(20):7295–7302. Copyright (2022) American Chemical Society.Citation90 (C) PCN-224(Fe) hybridized gold nanoparticles as a bifunctional nanozyme for glucose sensing. Tong PH, Wang JJ, Hu X-L, et al. Metal-organic framework (MOF) hybridized gold nanoparticles as a bifunctional nanozyme for glucose sensing. Chem Sci. 2023;14:7762–7769. Creative Commons.Citation91 (D) Fe-MOF-525 Enables Benchmark Electrochemical Biosensing. Reprinted from Zhou ZY, Wang J, Hou S, et al. Room Temperature Synthesis Mediated Porphyrinic NanoMOF Enables Benchmark Electrochemical Biosensing. Small. 2023. 2301933. Creative Commons.Citation93 (E) MIL-88A-Derived Fe3O4@C Hierarchical Nanocomposites for Electrochemical Sensing. Wang L, Zhang Y, Li X, et al. The MIL-88A-Derived Fe3O4-Carbon Hierarchical Nanocomposites for Electrochemical Sensing. Sci Rep. 2015;5:14341. Creative Commons.Citation104
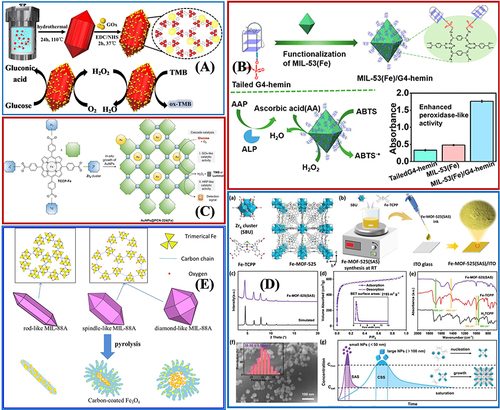
Table 3 Summary of Biosensing Applications of Fe-MOFs in This Review
Figure 10 The practical application of Fe-MOFs in biocatalysis: (A) Cu/Au/Pt TNP-modified TCPP-(Fe) nanozyme. Reprinted from Wu P, Gong F, Feng X, et al. Multimetallic nanoparticles decorated metal-organic framework for boosting peroxidase-like catalytic activity and its application in point-of-care testing. J Nanobiotechnol. 2023;21:185. Creative Commons.Citation112 (B) MIL-101(Cr/Fe) used as a platform for MP-8 enzyme immobilization. Reprinted from Kesse X, Sicard C, Steunou N, et al. Encapsulation of Microperoxidase-8 into MIL-101(Cr/Fe) Nanoparticles: a New Biocatalyst for the Epoxidation of Styrene. Eur J Inorg Chem. 2023;26:e202300040. Creative Commons.Citation115 (C) Multi-compartmental MOF microreactors for chemo-enzymatic cascade catalysis. Reprinted from Tian D, Hao R, Zhang X, et al. Multi-compartmental MOF microreactors derived from Pickering double emulsions for chemo-enzymatic cascade catalysis. Nat Commun. 2023;14:3226. Creative Commons.Citation116 (D) Fe-MOF based bio-/enzyme-mimics used for cancer treatment and anti-tumor principle. Reprinted from Xiang X, Pang H, Ma T, et al. Ultrasound targeted microbubble destruction combined with Fe-MOF based bio-/enzyme-mimics nanoparticles for treating of cancer. J Nanobiotechnol. 2021;19:92. Creative Commons.Citation117
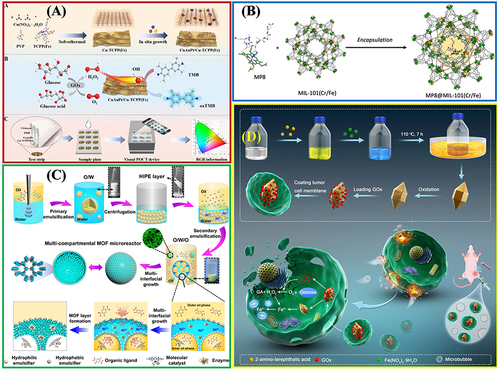
Figure 11 (A) Schematic illustration of imaging-guided therapy. (B) Photodynamic tumor therapy (PDT) by reversing multiple resistances. Reprinted from Liu P, Zhou Y, Shi X, et al. A cyclic nano-reactor achieving enhanced photodynamic tumor therapy by reversing multiple resistances. J Nanobiotechnol. 2021;19:149. Creative Commons.Citation138 (C) MRI imaging of orthotopic pancreatic murine tumors. Reprinted from Rojas JD, Joiner JB, Velasco B, et al. Validation of a combined ultrasound and bioluminescence imaging system with magnetic resonance imaging in orthotopic pancreatic murine tumors. Sci Rep. 2022;12:102. Creative Commons.Citation130 (D) PAI images and (E) Photothermal photographs of doxorubicin@MIL-100 compound. Reprinted from Zhiming H, Caina X, Liang Y, et al. Multifunctional drug delivery nanoparticles based on MIL-100 (Fe) for photoacoustic imaging-guided synergistic chemodynamic/chemo/photothermal breast cancer therapy. Mater Design. 2022;223:111132. Creative Commons..Citation131
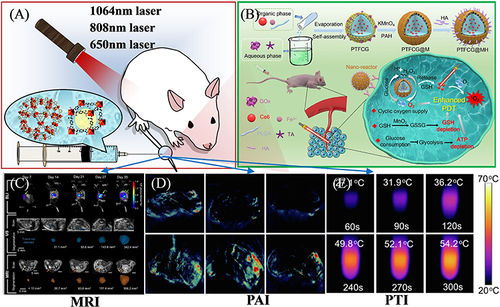
Figure 12 Active site and main applications of antibacterial Fe-MOFs nanomaterials and Their Composites.
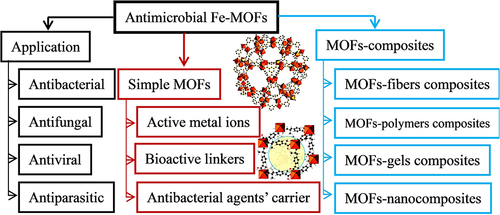
Figure 13 (A) Surface-Anchored MOFs-Cotton Material with antibacterial properties. Reprinted from Rubin HN, Neufeld BH, Reynolds MM. Surface-anchored metal–organic framework–cotton material for tunable antibacterial copper delivery. ACS Appl Mater Interfaces. 2018;10(17):15189–15199. Copyright (2018) American Chemical Society.Citation162 (B) The antibacterial mechanisms of Fe(III)-MOF towards different types of microorganisms, fungus, and yeast. Reprinted from Sheta SM, Salem SR, El‑Sheikh SM. A novel Iron (III)‑based MOF: synthesis, characterization, biological, and antimicrobial activity study. J Mater Res. 2022;37(14):2357–2367. Creative Commons.Citation163 (C) Synthesis of a mesoporous MIL-100(Fe) bacteria exoskeleton Reprinted from Permyakova A, Kakar A, Bachir J, et al. In Situ Synthesis of a Mesoporous MIL-100(Fe) Bacteria Exoskeleton. ACS Materials Lett. 2023;5(1):79–84. Copyright (2023) American Chemical Society.Citation139 (D) Smart MIL-88B(Fe) coating as a host matrix for the antibiofilm compound. Reprinted from Claes B, Boudewijns T, Muchez L, et al. Smart metal–organic framework coatings: triggered antibiofilm compound release. ACS Appl Mater Interfaces. 2017;9(5):4440–4449. Copyright (2017) American Chemical Society.Citation165 (E) Antibacterial properties of Carvacrol encapsulated in MIL-100 (Fe) nanoparticles. Reprinted from Caamaño K, Heras-Mozos R, Calbo J, et al. Exploiting the Redox Activity of MIL-100(Fe) Carrier Enables Prolonged Carvacrol Antimicrobial Activity. ACS Appl Mater Interfaces. 2022;14:10758–10768. Creative Commons.Citation166
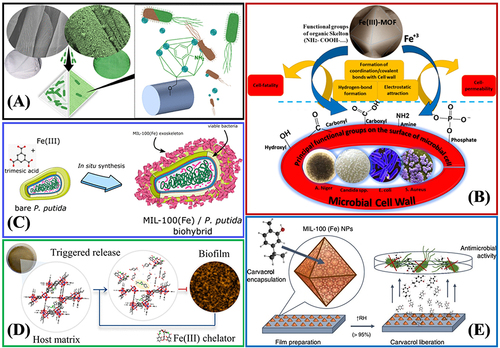