Figures & data
Table 1. Potential AbcR1 targets in A. tumefaciens identified by 2D proteomics
Figure 1. Identification of known AbcR1 targets by 2D-PAGE. Subsections of 2D gels showing Atu2422 (A) and Atu1879 (B) from A. tumefaciens WT (closed black circle) and ∆AbcR1 deletion mutant (dotted black circle) and northern blot analyses of atu2422 (B) and atu1879 (C) transcripts in different growth phases. The WT and the ∆AbcR1 deletion mutant (∆R1) were grown to exponential (OD600: 0.5) or stationary phase (OD600: 1.5) in YEB medium. Eight µg of total RNA were separated on 1.2% denaturing agarose gels. Ethidiumbromide-stained 16S rRNAs were used as loading control.
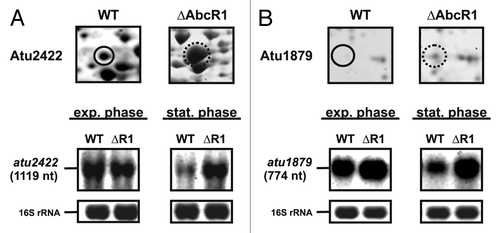
Figure 2. Validation of new AbcR1 targets. Subsections of 2D gels showing Atu4577 (A), MalE (B), Atu4046 (C), Atu4678 (D), DppA (E), Atu0857 (F), FrcB (G), and AtpH (H) from A. tumefaciens wild-type (closed black circles) and ∆AbcR1 mutant (dotted black circles) and corresponding northern blot analyses of target mRNAs in different growth phases. The wild-type (WT) and the ∆AbcR1 deletion mutant (∆R1) were grown and treated as in .
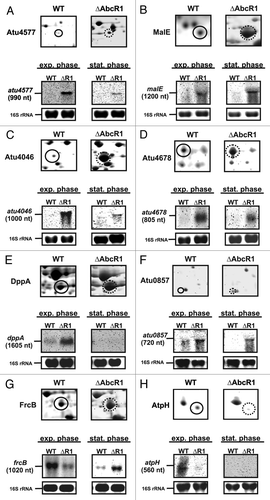
Figure 3. Altered protein synthesis in the ∆hfq mutant reveals new putative AbcR1 targets. (A) Total protein samples from stationary growth phase (OD600: 1.0) of A. tumefaciens WT and the hfq deletion mutant (∆hfq) were subjected to two-dimensional gel electrophoresis. Proteins overrepresented in WT or in ∆hfq preparations are shown in green or red, respectively. Proteins present in equal amounts in both preparations appear in yellow. Six (green spots) and 22 (red spots) proteins significantly accumulated equally in three replicates of WT (closed white circles) or in ∆hfq (dotted white circles) were analyzed via MALDI-TOF. Altered proteins that were not identified by MALDI-TOF are marked with numbers. #, proteins were identified in multiple spots. (B) Venn diagram comparing altered proteins from different proteomic approaches (∆hfq 1D-gel, ∆hfq 2D-gel, and ∆AbcR1 2D-gel) in A. tumefaciens. ABC transporter components are underlined. (+) or (-) indicate over- or underrepresentation of proteins in deletion mutants.
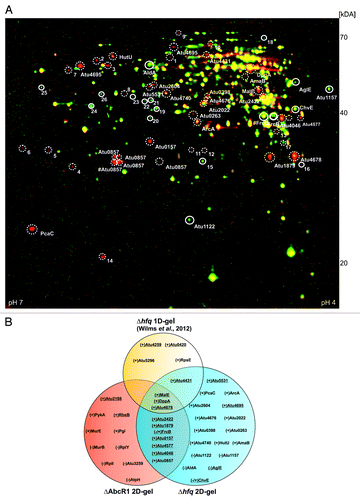
Figure 4. Verification of additional AbcR1 targets. Northern blot analyses of atu4413 (A), atu4259 (B), chvE (C), nocT (D), attC (E), and atu3114 (F) mRNAs in different growth phases. The wild-type and the ∆AbcR1 deletion mutant (∆R1) were grown to exponential (OD600: 0.5) or stationary phase (OD600: 1.5) in YEB medium. Eight µg of total RNA were separated on 1.2% denaturing agarose gels. Ethidiumbromide-stained 16S rRNAs were used as loading control.
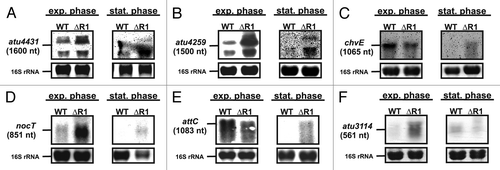
Figure 5. Comparative computational predictions suggest two functional AbcR1 modules. Sequence alignment (A) and consensus structure (B) of AbcR1 in A. tumefaciens C58 (A.t.), A. radiobacter K84 (A.r.), R. etli CFN42 (R.e.), R. leguminosarum bv. vicae (R.l.), R. etli 652 (R.e.652) S. meliloti 1021 (S.m.), and S. medicae WSM419 (S.md.). Sequence conservation is given in bold letters. Nucleotides highly conserved in structure are marked in red. The calculated structure is given in dot-bracket notation above the alignment. Grey shaded boxes (A) or gray marked nucleotides (B) represent regions M1 and M2. Visualization of the predicted binding regions in AbcR1 (C) and the experimentally verified targets of AbcR1 (D). The density plots at the top indicate the relative frequency of sRNA or mRNA nucleotide positions in the predicted AbcR1–target mRNA interactions. The density plots combine all optimal predictions for the 15 conserved verified targets in all included homologs of AbcR1 (C) or target mRNAs (D). Distinct interaction domains are indicated by local maxima marked with upright lines. Below the density plots, schematic alignments of the AbcR1 homologs (C) and the targets (D) are drawn to visualize the predicted optimal and suboptimal interactions for each organism. Each alignment contains eight lines, one for each organism included in the CopraRNA prediction. The order of the organisms in the alignment from top to bottom is: A. tumefaciens C58 (A.t.), A. radiobacter K84 (A.r.), R. etli CFN42 (R.e.), R. leguminosarum bv. vicae (R.l.), R. etli 652 (R.e.652) S. meliloti 1021 (S.m.), and S. medicae WSM419 (S.md.). The aligned regions are colored in gray and the optimal predicted interaction regions are given in different colors (for contrast only). The respective best suboptimal interaction site predictions are additionally shown by gray lines. White regions indicate gaps inside the AbcR1 alignment. Locus tag and gene name (if available) of target mRNAs are given on the right. A vertical gray line indicates the start codon. Numbering of bases in mRNA alignments is given relative to the start codon (D). For detailed visualization of optimal and suboptimal interactions for AbcR1 and its verified target mRNAs in A. tumefaciens, see Table S3.
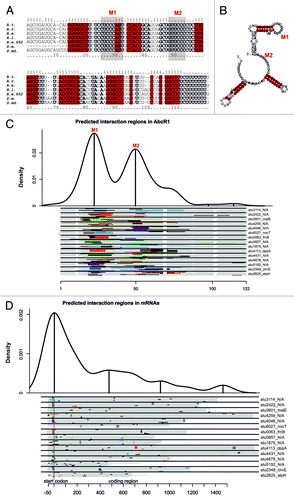
Figure 6. Binding of target mRNAs at the translation initiation region by two distinct functional modules. (A) Secondary structures of WT AbcR1 and the variants Mut1, Mut2, and Mut1+2. Band shift experiments with AbcR1 variants and atu2422 (B), frcB (B), atu4678 (C), chvE (C), and atpH (D) mRNA fragments (~-50/+100 nt relative to the AUG start codon). Predicted IntaRNA duplexes formed by AbcR1 and target mRNAs are shown to the left. Numbering of mRNA nucleotides is given relative to the AUG/GUG start codon. 32P-labeled AbcR1 variants (< 0.05 pmol) were incubated with increasing concentrations of unlabeled target RNAs at 30 °C for 20 min. Final concentrations of unlabeled RNA were added in 100 (lanes 2), 200 (lanes 3), and 400 (lanes 4) fold excess. Samples shown in lanes 1 were incubated with water (control).
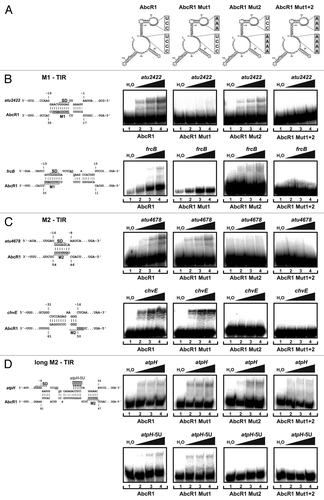
Figure 7. Binding of target mRNAs in the coding sequence by AbcR1. (Top) Secondary structures of AbcR1 wild-type, the variants Mut1, Mut2, and Mut1+2. Band shift experiments with AbcR1 variants and atu1879 (A), atu3114 (A), and malE (B) mRNA fragments (~150 nt). Predicted IntaRNA duplexes formed by AbcR1 and target mRNAs are shown to the left. Numbering of mRNA nucleotides is given relative to the start codon. 32P-labeled AbcR1 variants (< 0.05 pmol) were incubated with increasing concentrations of unlabeled target RNAs at 30 °C for 20 min. Final concentrations of unlabeled RNA were added in 100 (lanes 2), 200 (lanes 3) and 400 (lanes 4) fold excess. Samples from lanes 1 were incubated with water (control).
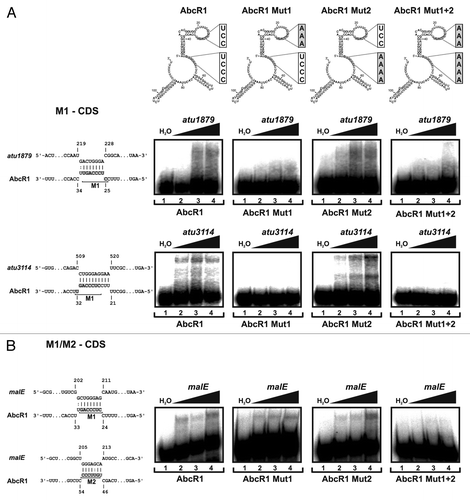
Figure 8. In vivo validation of AbcR1 modules 1 and 2. Northern blot analyses of AbcR1 (A), atu2422 (B), atu4678 (C), atu3114 (D), and atu4431 (E) transcripts from cultures of the A. tumefaciens wild-type (WT) or the ΔAbcR1/2 deletion mutant (ΔR1/2) complemented with a plasmid expressing different AbcR1 variants (+R1, +Mut1, +Mut2, +Mut1+2). The strains were grown in YEB medium. +v: control strains harboring the empty vector. Eight μg of total RNA were separated on 1.2% denaturing agarose gels. Ethidiumbromide-stained tRNAs or 16S rRNAs were used as loading control.
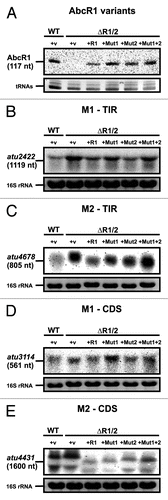
Figure 9. Precise mapping of AbcR1 binding sites in target mRNAs. (A) Principle of AbcR1 binding-site mapping by toeprinting analysis. -AbcR1, without AbcR1; reverse transcription (RT) starting from a primer complementary to the target mRNA sequence transcribes a full-length product. +AbcR1, pairing of AbcR1 with the target sequence terminates reverse transcription (truncated product). AbcR1 binding-site mapping on atu2422 (B), atu3114 (C), and malE (D) RNA fragments was performed as described in Experimental procedures. The position of truncated products is indicated to the right. mRNA nucleotides involved in M1 binding are shown to the left. Concentrations of AbcR1 RNAs were 1.5 pmol µl−1 (lane 2) and 2.5 pmol µl−1 (lane 3).
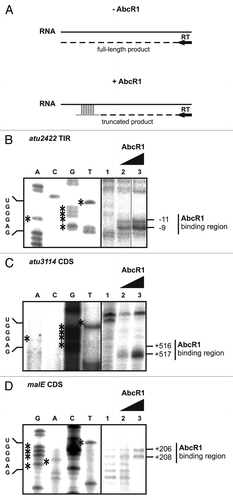
Figure 10. AbcR1 M1 and M2 target mRNAs in the TIR and the CDS for degradation. Northern blot analyses of frcB (A), atu4678 (B), atu3114 (C), atu4431 (D), malE (E), and atpH (F) transcripts from cultures treated with rifampicin. Cultures of the A. tumefaciens wild-type (WT) or the ΔAbcR1 deletion mutant (ΔR1) were grown to exponential or stationary (in case of frcB) growth phase in YEB medium and treated with rifampicin (250 mg ml-1). Total RNA fractions were collected at the indicated time points. Eight μg of total RNA were separated on 1.2% denaturing agarose gels. Ethidiumbromide-stained 16S rRNAs were used as loading control. Quantification of transcript stabilities and their calculated half-lives are given to the right.
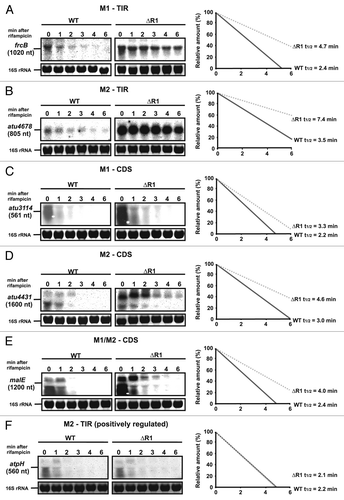
Figure 11. The AbcR1 regulon of A. tumefaciens. AbcR1 controls mRNAs of periplasmic substrate-binding proteins of 14 ABC transporters (sugars and amino acids to the left and right, respectively), an annotated oxidoreductase (atu0857) and AtpH. Module 1 (red) and module 2 (blue) dependent genes are sorted toward the top and bottom of the schematic cell, respectively. The interaction region (TIR or CDS) and the mode of action (repression or activation) are indicated. Dashed lines refer to computationally predicted interactions.
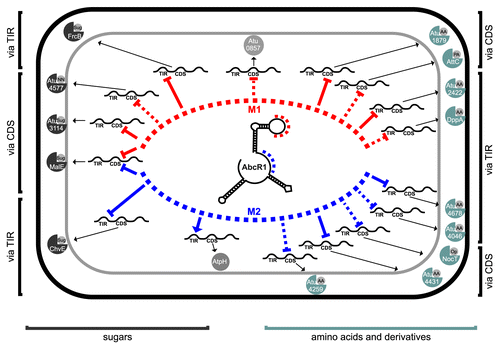