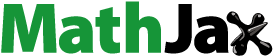
ABSTRACT
Chemical energy release from high octane number fuels via low temperature heat release (LTHR) can help develop high-efficiency gasoline engines by promoting ultra-lean combustion in spark ignition engines and improving combustion control in gasoline compression ignition engines. A recently developed experimental technique that permits isolated LTHR investigations in motored engines was used to characterize the LTHR behavior of iso-octane/air mixtures ranging in strength from to
at multiple inlet temperature conditions (
to
°C). LTHR changes were studied by observing variations in exhaust CO emissions and exhaust temperature increase. Observed heat release results were explained using cylinder mixture pressure-temperature histories alongside supporting chemical kinetics modeling estimates of mixture reactivity in the form of chemical ignition delay (ID) time. The effects of fuel enrichment on iso-octane/air mixture reactivity were found to be non-uniform and dependent on mixtures’ thermal state trajectories in the LTHR ID peninsula. LTHR intensity measurements were used to discuss changes in mixture
sensitivity at different engine inlet conditions. It was shown that by appropriately adjusting mixture thermal conditions via charge cooling from direct fuel injection and intake air heating, reactivity enhancements could be exploited maximally; and strong, positive, linear
sensitivity of around 10 J per 0.1 increase in
could be realized across a wide range of equivalence ratios from 0.05–1.2. It was also found that dominance of charge cooling effects at rich conditions resulted in negative and zero
sensitivity regions.
Introduction
Certain fuels exhibit two-stage auto-ignition (AI) chemistry, where mixture temperature and ignition delay (ID) are non-monotonically related due to the existence of a negative temperature coefficient (NTC) thermal band (Leppard Citation1990). In internal combustion engines, such fuels release energy through low (<850 K), intermediate (850–1200 K) and high temperature (>1200 K) reactions (Waqas et al. Citation2019). Heat released via low temperature reactions is known as low-temperature heat release (LTHR) and depends on the reacting mixture’s NTC characteristics. The two-stage chemistry of straight chain, diesel-like, low octane number fuels such as n-heptane (Pan et al. Citation2016; Saisirirat et al. Citation2009) is well established and LTHR has been demonstrated to improve emissions and performance of controlled auto-ignition (CAI) engines fueled by such fuels (Bajwa, Leach, and Davy Citation2023), for example by serving as an ignition trigger and promoting main heat release (Borgqvist, Tunestal, and Johansson Citation2013), making combustion more resilient to changes in charge state (Sjöberg and Dec Citation2006), or smoothing heat release and reducing NOx emissions via staged AI (Sjöberg and Dec Citation2006; Yang et al. Citation2011).
Similar benefits can be extended to engines fueled by high octane number, gasoline-like fuels if LTHR can be reliably realized and controlled. This is, however, difficult to achieve as such fuels generally exhibit weak two-stage AI chemistry at typical engine inlet conditions (Saisirirat et al. Citation2009). If realized, the benefits of LTHR can be exploited in various gasoline-based engines ranging from conventional spark ignition (SI) engines to different gasoline fueled CAI embodiments like partially premixed compression ignition (Kalghatgi, Risberg, and Ångström Citation2007) and spark-assisted compression ignition engines (Olesky et al. Citation2013). Gasoline LTHR can help improve combustion control and extend operating envelopes of CAI engines, and enable thermally efficient ultra-lean operation in SI engines because of its low temperature and spatially distributed (“volumetric”) nature (Collin et al. Citation2003). Approximately 80% of the global light-duty vehicle fleet is powered by gasoline engines (Senecal and Leach Citation2021, Ch. 9), therefore, the potential for reducing road transport CO2 and pollutant emissions is significant. Some benefits of gasoline LTHR have already been demonstrated for SI engines by pre-spark heat release studies as improved knock resistance (Splitter et al. Citation2019; Szybist and Splitter Citation2017), and the promise of improved combustion control for gasoline CAI engines has been explored through -sensitivity studies (Alemahdi et al. Citation2022; Dec, Yang, and Dronniou Citation2011; Pintor, Dec, and Gentz Citation2019).
This paper investigates the LTHR characteristics of iso-octane, used often as a gasoline surrogate (DelVescovo et al. Citation2020; Pintor, Dec, and Gentz Citation2019), to help advance the understanding of low-temperature chemistry of such fuels under engine-like conditions. The LTHR potential for different gasoline fuels can vary depending on the particular fuel’s octane sensitivity (Alemahdi et al. Citation2022; Szybist and Splitter Citation2017), which indirectly captures the extent of its underlying two-stage chemistry (NTC) characteristics. Iso-octane, being a primary reference fuel (PRF), has zero octane sensitivity by definition, and possesses relatively pronounced, for a high octane number fuel, NTC characteristics. Its NTC and reactivity (ID) attributes are similar to those of conventional gasoline fuels (Pintor, Dec, and Gentz Citation2019). Other reference fuels like ethanol or toluene-ethanol blended reference fuels have non-zero octane sensitivities and exhibit weaker LTHR (Alemahdi et al. Citation2022; Shahanaghi et al. Citation2022).
Previous engine studies using iso-octane have identified the following effects of changes in compressed mixture thermal and chemical states on LTHR behavior:
Increasing compression pressure generally supports LTHR onset and increases its intensity by making the mixture’s thermal state traverse low ID regions (Szybist and Splitter Citation2017; White et al. Citation2023).
Depending on the operating pressure and equivalence ratio, which determines the mixture’s thermal state relative to the NTC region, increasing compression temperature can weaken (White et al. Citation2023) or strengthen LTHR (Szybist and Splitter Citation2017) even though the effect of solely changing mixture temperature is to increase reactivity (Pintor, Dec, and Gentz Citation2019).
Increasing compression time by reducing engine speed strengthens LTHR by allowing more time for chain-branching exothermic reactions to occur, and can even lead to high temperature heat release if the mixture state resides in low ID regions for a prolonged period (Shahanaghi et al. Citation2022; Splitter et al. Citation2019; White et al. Citation2023).
Under suitable thermal conditions, increasing the fuel-air equivalence ratio (
) enhances mixture kinetics (i.e. shortens ID) and increases LTHR (Dec and Sjöberg Citation2004; Pintor, Dec, and Gentz Citation2019; Sjöberg and Dec Citation2006).
This enhancement is weaker than for lower octane number fuels. Another factor that can influence LTHR is the presence of residual gases, which can have varied net effects depending on the interplay between their diluting, heat capacity, chemical, and charge heating influences. DelVescovo et al. (Citation2020) predicted that the presence of NO in residual gases can significantly strengthen iso-octane LTHR.
A mixture property which has been used to study the effects of changing on reactivity is
-sensitivity, which can be expressed in general terms as:
The Reactivity Index can be any quantifier of a mixture’s propensity to combust in an engine, e.g. ignition delay computed from chemical kinetics models (Pintor, Dec, and Gentz Citation2019), or empirically determined auto-ignition or combustion indices like crank angle location of 10% (Dec, Yang, and Dronniou Citation2011; Yang et al. Citation2011) or 50% (Sjöberg and Dec Citation2006) mass fraction burned point, pressure at start of heat release (Alemahdi, García, and Tunér Citation2022), or compression ratio in a cooperative fuel research engine needed to keep combustion phasing constant for different mixtures (Alemahdi et al. Citation2022).
Dec and Sjöberg (Citation2004) attempted to smooth heat release in gasoline CAI engines by using -sensitivity to create a LTHR intensity distribution corresponding to a mixture
distribution generated via partial fuel stratification. It was hypothesized that richer strata would have significantly enhanced reactivity and would thus release more energy via “pre-ignition” reactions (i.e. LTHR), compared to leaner, less reactive strata. Consequently, LTHR would take place in stages across the cylinder (“sequential auto-ignition”) and cumulative heat release would be milder. Desired smoothing was achieved for a two-stage AI chemistry fuel (PRF-73, a mixture of 73% iso-octane and 27% n-heptane), but not for iso-octane, which at the naturally aspirated conditions studied did not exhibit NTC behavior. This led the authors to conclude that mixtures exhibiting single-stage AI chemistry could not sequentially auto-ignite as their reactivity did not increase appreciably with
, i.e. they had weak
-sensitivity. For the iso-octane mixtures, the thermal effects of increased direct fuel injection (charge cooling and reduction in the compressed mixture’s ratio of specific heats,
) dominated the mild chemical effects.
Later studies by Dec and coworkers (Dec, Yang, and Dronniou Citation2011; Pintor, Dec, and Gentz Citation2019) on boosted CAI engines found that weak NTC fuels like iso-octane exhibited noticeable -sensitivity when compression pressures were increased, and could thus potentially be used for sequential auto-ignition. The
-sensitivity of gasoline and iso-octane was reported to increase significantly as inlet pressures were increased to 2.5 bar. Chemical kinetics modeling using a detailed (2027 species) Lawrence Livermore National Laboratory (LLNL) mechanism revealed that highest
-sensitivities existed in the NTC region, especially near the “cold side of the NTC zone.” The emergence of
-sensitivity at high pressures was, however, attributed to intermediate (850–1200 K), not low, temperature heat release reactions based on a categorization approach that used H2O2 as a combustion tracer.
This work will explore, in-depth, the reactivity effects of iso-octane and the potential for promoting LTHR via fuel enrichment, as well as managing the intensity and phasing of LTHR by controlling mixture thermal conditions. Previous iso-octane LTHR and -sensitivity studies (Alemahdi et al. Citation2022; Dec and Sjöberg Citation2004; Sjöberg and Dec Citation2006; Yang et al. Citation2011) have been limited in their explorations by difficulties in observing reactivity effects on LTHR in isolation because of confounding effects of high-temperature reactions, which change the thermal and chemical properties of the compressed mixture. Dec and Sjöberg (Citation2004) used a sequenced firing technique to partially isolate chemical kinetics effects from thermal effects where every
cycle had a richer mixture than the preceding 18 cycles. Previous studies have experimentally looked at mixture strengths up to
(Sjöberg and Dec Citation2006; Yang et al. Citation2011), likely because of high-temperature heat release intensity and pressure rise rate limitations.
A recently developed experimental technique (White, Bajwa, and Leach Citation2023) that enables isolated investigation of LTHR is used here to study the LTHR and -sensitivity characteristics of iso-octane/air mixtures ranging in strength from
-
. The technique realizes isolated LTHR by motoring a moderate compression ratio gasoline direct injection engine at elevated intake temperatures and, if need be, pressures to induce LTHR reactions without triggering high temperature heat release. It thus captures the net effects of the mixture’s thermal state and chemical reactivity on LTHR. The contributions of the two changes are then isolated by using chemical kinetics simulations to compute mixture ID and cylinder pressure measurements to estimate the compressed mixture’s pressure-temperature (P-T) histories.
Methodology
Experimental facility
A single cylinder, gasoline direct injection engine based on a Ricardo Hydra bottom end was used for the experimental investigations. Its technical specifications are summarized in . The engine was coupled to a 57 kW AC motoring dynamometer (Vascat MAC-Q) that maintained the required speed (±1 rpm). Fuel injection settings (timing, duration, rail pressure) were controlled by a Schaeffler Protronic ECU via an ETAS INCA interface. Ignition was disabled during the LTHR testing using the ECU. The engine was operated at wide open throttle and intake pressure was maintained at desired levels using an external boosting rig. Intake air was heated using an electric heater installed upstream of the throttle valve and intake plenum. Engine coolant and oil temperatures were maintained at 90°C during experiments using closed loop temperature control systems.
Table 1. Engine specifications.
Inlet pressure and temperature were measured around 35 cm upstream of the inlet ports using a flexible silicon pressure sensor (Druck UNIK 5000) and a 3 mm k-type thermocouple, respectively. Fuel and air flow rates were measured using a Coriolis (Siemens FC Mass 2100) and a hot wire (Sierra-CP Airtrak 628S) flow meter, respectively. A Horiba MEXA-ONE emissions analyzer was used to measure exhaust composition. These “slow-speed” parameters were recorded at a frequency of 1 Hz using a Sierra-CP CADET system. A water-cooled piezoelectric transducer (Kistler-6041B) was used to measure cylinder pressure, which was logged at a resolution of 0.1°CA using an AVL Indiset data acquisition system. Slow speed data was logged for 30 seconds, while high-speed measurements were recorded for 300 cycles. AVL Concerto and custom MATLAB scripts were used for data post-processing. More details about the test cell can be found in previous publications (Leach, Davy, and Terry Citation2021; Papaioannou et al. Citation2019).
Operating conditions
Tests were performed at a constant speed of 1500 rpm, with the exception of some preliminary test points to demonstrate the difference between LTHR and HTHR that were at 1000 rpm (). The inlet pressure was maintained at 1.5 bar (absolute), and mixture strengths were varied from very lean ( 0.02) to very rich (
= 1.2–1.6) conditions at five inlet temperatures (40–120°C). Equivalence ratios were calculated from fuel and air flow measurements, and verified from exhaust emissions using the “Spindt” method (Bresenham, Reisel, and Neusen Citation1998) up to
0.55. Beyond that, the unburned hydrocarbon sensor started to saturate and only the air and fuel flow measurements were used for determining
. Inlet temperature was maintained within ±2°C and pressure within ±0.01 bar. Engine settings are listed in summary of all the test points (174 in total) is shown in .
Figure 2. P-T trajectory for mixtures at
°C and 1000 rpm undergoing isolated LTHR and LTHR + HTHR with research octane number (RON) and motor octane number (MON) trajectories shown for reference.
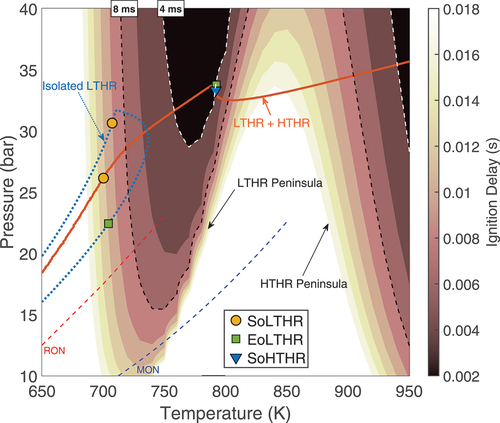
Figure 3. Apparent rate of heat release for mixtures at
°C and 1000 rpm undergoing isolated LTHR and LTHR + HTHR.
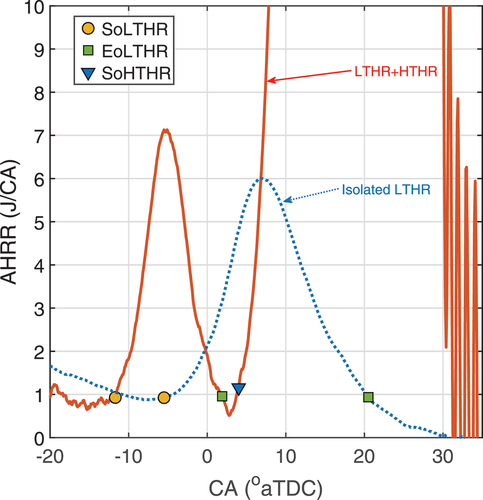
Table 2. Engine settings.
Apparent heat release analysis
Net apparent heat release rate (AHRR) was calculated from measured cylinder pressure as described in (White, Bajwa, and Leach Citation2023). A heuristic feature identification-based method, adapted from Saisirirat et al. (Citation2009), was then to determine LTHR duration, magnitude, and phasing. Start of LTHR (SoLTHR) was defined as the positive inflection point in the AHRR curve and end of LTHR (EoLTHR) was defined as the point when AHRR returned to the SoLTHR level. Start of high-temperature heat release (SoHTHR) was determined similarly. LTHR duration was defined as the difference between EoLTHR and SoLTHR, and the center of combustion (median of LTHR crest, CA50) was used to index LTHR phasing.
Modelling
Ignition delay simulations were performed in CHEMKIN using the closed homogeneous ignition delay model with a constant volume. The simulations modeled 200 ms of reactions, and reported ID defined by a 50 K rise in temperature in order to detect the relatively small rises in temperature caused by LTHR. The approach has been successfully used in previous engine LTHR studies (Splitter et al. Citation2019; Szybist and Splitter Citation2017) for indexing LTHR ID times. Note, however, that the LTHR ID calculated here is slightly different from the first-stage ID calculated in the literature from the temperature rise rate (Pan et al. Citation2016; Tao et al. Citation2019). The LTHR ID definition was selected to manage the computational expense of indexing low temperature reactivity at the wide range of mixture temperature, pressure, and points studied. Mixtures of air and iso-octane were modeled at multiple equivalence ratios ranging between 0.064 and 1.3 using a reduced Lawrence Livermore National Laboratory (LLNL) gasoline surrogate mechanism with 165 species and 839 reactions (Wu et al. Citation2017).
P-T trajectories were generated using measured cylinder pressure and calculated average cylinder temperature. The cylinder temperature was calculated using the ideal gas law with measured cylinder pressure, calculated cylinder volume, and estimated trapped cylinder mass.
Results
Low vs high temperature heat release
shows pressure-temperature (P-T) traces on an ID map for two types of LTHR cycles – one (the orange solid line) which led to high-temperature heat release (HTHR) and one which terminated after LTHR, i.e. exhibited isolated LTHR (the blue dashed line). The corresponding AHRR curves are shown in . The results demonstrate the distinct nature of the two heat release stages, whereby the first stage exists in the LTHR band (700–800 K), referred to here as the LTHR peninsula; and the high temperature stage takes place in the so-called HTHR peninsula. The end of LTHR and the subsequent start of HTHR in the HTHR cycle are characterized by the NTC-induced drop in cylinder pressure, followed by a rapid rise in mixture temperature resulting from high temperature combustion. Whereas, for the isolated LTHR cycle, the pressure and temperature drop after the end of LTHR because of expansion. Based on these distinct LTHR and HTHR characteristics it is reasoned that the measurements from isolated LTHR experiments carry signatures of only LTHR. Note that the results in are from a 1000 rpm case because HTHR was not observed at higher speeds, as has been discussed in White, Bajwa, and Leach (Citation2023).
LTHR strength indices
To discuss the effects of changes in mixture state and reactivity on the strength of LTHR, high sensitivity heat release indicators are needed. The isolated LTHR experimental approach makes available multiple parameters to choose from – some directly measured ones like exhaust gas temperature (EGT), exhaust emissions, engine torque and indicated mean effective pressure (IMEP); and some indirectly computed ones like cumulative heat release (CHR) and integrated Livengood-Wu (LW) type progress variables (Pan et al. Citation2016).
The following parameters were compared using the entire experimental data set () to select appropriate LTHR indicators: IMEP, CHR, EGT rise relative to motored EGT, and exhaust CO, CO2, NOx, and O2 concentrations. LW scores, which have been shown to be high-sensitivity indicators of LTHR intensity (Pan et al. Citation2016; White, Bajwa, and Leach Citation2023), were not considered because their computation requires accurate quantification of cylinder temperature, which was difficult to accomplish for the current engine because of uncertainty associated with its residual gas fraction. Rough bulk cylinder temperatures computed using an estimated residual gas fraction of 10% are, however, used throughout the paper for qualitative discussions of “effective” ID times. These discussions assume that the shorter the effective ID for a given process, as computed by the LW progress variable score , the stronger will be the resulting LTHR.
Exhaust CO and temperature rise exhibited consistent trends with and high sensitivity to changing LTHR intensity, as shown in , respectively, which makes them promising LTHR intensity indicators. Both increased monotonically with CHR beyond an initial weak LTHR region (CHR <3 J) at approximate rates of 40 ppm/J and 0.5°C/J, respectively. The initial CHR insensitivity to heat release intensity highlights limitations of the apparent heat release rate based LTHR indexing method used when very small quantities of energy are released.
EGT rise results presented a wider spread in the weak LTHR (CHR = 0) band because, unlike exhaust CO, the effects of evaporative cooling from fuel vaporization (charge cooling) were directly captured. These effects were particularly pronounced for the very rich cases (see color bar to right) where there was no sensible LTHR as per the CO and CHR results. EGT results can also be influenced by variations in heat release phasing as they depend on the cylinder temperature and pressure at the point of exhaust valve opening, whereas post-LTHR CO concentrations can be assumed to be “frozen.” Therefore, CO was considered to be a more robust LTHR indicator and is thus preferred in the following discussion.
Exhaust CO2 also captures LTHR intensity changes as can be seen from the exhaust CO2 vs CO scatter plot in . It, however, has lower sensitivity than CO (11 vs 40 ppm/J) and is, therefore, a weak LTHR indicator. An inconsistency in trends can be observed at rich cases in the CO2 results, whereby CO2 concentrations do not return to motoring levels (440–470 ppm) even when CO results indicate cessation of LTHR reactions. This likely represents a limitation, for very rich mixtures, of the non-dispersive infrared type analyzer used. Notwithstanding this, all LTHR indicators considered (CO, CO2, CHR, EGT rise) showed consistent trends between and had correlation coefficients greater than 92%.
Equivalence ratio effects
LTHR intensity effects
The effects of changing on LTHR intensity are shown in by exhaust CO concentration measurements at the constant inlet temperature of 60°C. LTHR can be observed at very lean conditions, outside of the typical lean flammability limits of deflagrative combustion, which for iso-octane/air mixtures have been reported to range between
and
(Liu, Akram, and Wu Citation2020). CO concentration of 75 ppm was recorded at
, which is indicative of oxidation reactions taking place in the very lean mixture. LTHR at such lean conditions can be attributed to its occurrence at low temperatures and its volumetric nature, whereby oxidation reactions take place in the dilute homogeneous fuel-air mixture throughout the combustion chamber and cumulatively generate sensible heat release signatures.
In , increasing up to around
gradually strengthens LTHR, beyond which LTHR weakens rapidly reaching near-motoring levels for stoichiometric mixtures. These observations can be explained by the differences in the ID of the various
mixtures and their respective compression P-T histories. ID differences represent the effects of changes in AI chemistry of the mixture, specifically its reactivity, while the P-T trajectories represent thermal conditions.
ID effects
presents selected ID contours at different levels to illustrate the increase in mixture reactivity accompanying mixture enrichment. 8 and 4 ms ID contours are selected to, respectively, represent contours close to the periphery and the core of the LTHR peninsula. Doing so permits direct comparison of reactivity across different
levels. provides an example of a complete ID map at a constant
value. The contours in show that increasing
expands the LTHR peninsula, potentially enabling mixtures at lower pressure and temperature to undergo LTHR. The rightward expansion also increases the likelihood of LTHR reactions for higher temperature mixtures. The reactivity enhancement is more pronounced toward the high temperature (right) edge of the LTHR peninsula. This is consistent with detailed chemical kinetics modeling results from (Zhao and Law Citation2013) and (Pintor, Dec, and Gentz Citation2019). Another observation from is that relativity low marginal reactivity enhancement takes place for mixtures richer than
, i.e.
-sensitivity decreases for relatively rich mixtures.
The thermal effects of fuel enrichment result primarily from increased charge cooling as greater quantities of fuel are injected, and secondarily from reduction in the compressed mixture’s ratio of specific heats, . The charge cooling effects are expected to be less significant in non direct injection engines. Both of the fueling effects lower the cylinder pressure and temperature during compression, and move P-T trajectories leftward in the P-T space. This can be seen in in which the ID contours from are overlaid with P-T trajectories for various
cases. The leftward movement of the P-T traces from
to
moves the mixture thermal states closer to the low ID core of the LTHR peninsula, thus reducing the effective ID; plus, it permits increased exploitation of the rightward LTHR peninsula expansion (i.e. the high
-sensitivity region). The initial increase in LTHR intensity reported in is the combined result of these thermal and chemical influences of increased fueling. Additional fuel enrichment increases the effective ID because the compression P-T traces are shifted further left and brought close to the left edge of the LTHR peninsula where mixture reactivity is not enhanced appreciably (i.e. low
-sensitivity region).
LTHR based 
sensitivity characterization
results can be used to describe sensitivity behavior of the various compressed mixtures by using LTHR intensity as a reactivity index. At the selected inlet temperature and engine conditions, the near-linear increase in LTHR intensity up to
corresponds to a constant
sensitivity of around 420 ppmCO/0.1
, which translates to 10 J/0.1
using , i.e. an additional 10 J of heat is released per cycle via low temperature reactions for every 0.1 increase in
. This is followed by a period negative
sensitivity up to
. Further fuel addition renders the mixture
insensitive. The negative
-sensitivity region represents the relatively rich conditions where fuel injection induced P-T lowering effects dominate over mixture reactivity enhancement from mixture enrichment. Other LTHR strength indices discussed earlier can also be used as reactivity indices to describe
sensitivity changes.
LTHR phasing effects
The effects of changes on heat release phasing with respect to the total amount of heat released are shown in for mixtures in which heat release was detected using the AHRR technique employed. Combustion phasing and duration changes are summarized in . Upon mixture enrichment, combustion phasing is initially retarded from around − 7 CA°aTDC to 6 CA°aTDC as LTHR intensity increases up to
; after which, as LTHR weakens upon further fuel addition, combustion phasing retards at a much slower rate before settling at around 8 CA°aTDC for the richest cases. The retardation of combustion phasing is largely caused by combustion duration elongation as LTHR strengthens. Therefore, the combustion duration increases by almost the same number of degrees as the center of combustion retards. The slight decrease in combustion duration at
results from charge cooling induced LTHR weakening. The SoLTHR is slightly delayed upon mixture enrichment from − 6 to − 12 CA°aTDC (yellow circles in ), which contributes minimally to LTHR phasing retardation. The low sensitivity of SoLTHR to
is similar to results from (Pintor, Dec, and Gentz Citation2019) who, for a firing CAI engine, found the start of LTHR to be
-insensitive but that of main combustion (HTHR) to be
-sensitive. The current isolated-LTHR results show that even in the absence of hot combustion residuals, SoLTHR is
-insensitive. Therefore, charge enrichment or heating alone cannot be used to control SoLTHR. Other means of decreasing effective ID before SoLTHR would be needed, e.g. increasing pressure was shown to advance SoLTHR in (White, Bajwa, and Leach Citation2023).
Figure 10. Changes in LTHR intensity and phasing with changing at
°C. Colour map is scaled from
(lightest) to
(darkest).
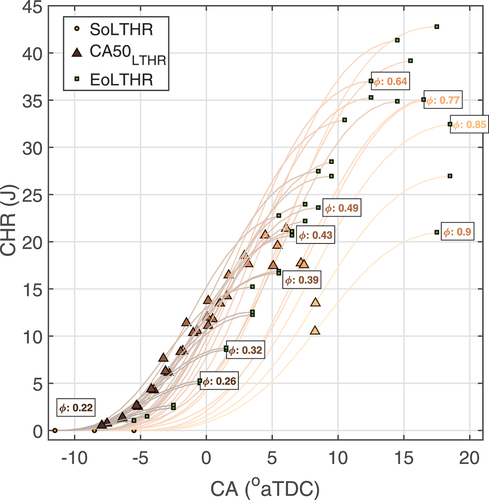
The LTHR phasing results also have the same trends as those from Yang et al. (Citation2011) who reported that CA10 (“hot-ignition timing;” similar to CA50 here) for iso-octane/air mixtures retarded as mixtures became richer. The phasing shift was attributed to reduction in compression temperatures and absence of two-stage AI chemistry at the engine inlet conditions (1 bar, 174°C). The reasons for CA50 retardation for the current boosted engine are, however, different as the iso-octane/air mixtures do exhibit NTC behavior leading to a significant reactivity enhancement (positive -sensitivity), and resulting in stronger and longer LTHR with later LTHR centers. This, then, leads to an advantage of direct quantification of LTHR, directly coupled to the NTC characteristics of the reacting mixture and thus prevents obfuscation by the “main” heat release.
A practical implication of the AHRR results is that the SoLTHR cannot serve as a LTHR -sensitivity indicator, whereas, LTHR phasing and duration, both of which increase as LTHR strengthens (), can be used for characterizing
-sensitivity in AHRR-sensible regions.
Temperature effects
It was concluded from results that the increase in LTHR upon fuel enrichment was limited by the thermal cooling effects of fuel injection which shifted the compressed mixture’s thermal state leftward to the low -sensitivity region. It is hypothesized that if the P-T trajectories can somehow be translated rightward, the high
-sensitivity region can be exploited to overpower the reactivity diminishing thermal effects of fueling. This is attempted by increasing inlet temperature to raise the compression temperatures.
Inlet temperature is varied from 40 to 120°C in 20°C increments. The resulting effects on LTHR intensity are shown in at different levels. Increasing inlet temperature raises the peak LTHR intensity and delays the following charge cooling drop to richer equivalence ratios. This is shown separately in . The peak LTHR intensity nearly doubles as the inlet temperatures rise from 40°C to 120°C and the corresponding
(
) shifts from
to
.
marks the equivalence ratio where
-sensitivity changes from positive to negative.
Figure 13. (a) Maximum exhaust CO concentration and (b) corresponding at different inlet temperatures.
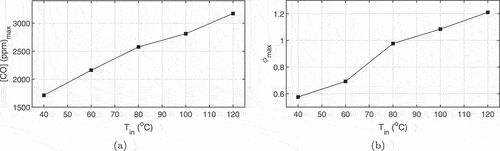
These trends can be explained by looking at the respective thermal state histories to deduce the effective ID times. shows an ID map for mixtures with P-T traces at different inlet temperatures overlaid. At such relatively lean conditions, charge cooling effects are weak and the LTHR peninsula is relatively narrow. Hence, increasing the inlet temperature significantly shifts the P-T traces from the LTHR peninsula core rightward across the high-temperature edge, leading to an increase in the effective ID time. Consequently, at very lean conditions (
), inlet heating has the net effect of weakening LTHR as confirmed by the measured LTHR results in .
As mixtures become richer, charge cooling and specific heat ratio effects become more pronounced as can be seen by the leftward shift in the P-T trajectories (relative to leaner mixtures at the same temperatures) for mixtures shown in . This decreases the effective ID time for inlet temperature cases of 60°C and higher, and thus strengthens LTHR. For the 40°C case, however, the excessive leftward shift increases the effective ID time and weakens LTHR (). shows P-T traces for the stoichiometric case and the same trends continue, whereby the high temperature cases (80–120°C) experience increased reactivity while for the two low-temperature cases, LTHR is quenched as they are shifted to the low temperature, long ID region. Thus, for relatively rich mixtures, increasing inlet temperature strengthens LTHR because the thermal effects of fueling shift the compression P-T histories to high reactivity regions. These trends are consistent with those reported by Szybist and Splitter (Citation2017) for stoichiometric iso-octane/air mixtures with similar compression P-T histories (“condition C”).
The increase in the peak LTHR magnitude with inlet heating () is the net result of the interplays between the two competing thermal influences (charge cooling from fueling, and inlet heating), and the chemical effects of asymmetric LTHR ID peninsula expansion discussed above.
Another observation from the ID maps of the rich mixtures () is that for these high reactivity mixtures, the highest inlet temperatures mixtures benefit from two regions of ID decrease – first as the thermal states pass through the LTHR peninsula core during compression (ID decreases from > 18 to <4 ms), and then toward the end of LTHR as mixture states start encroaching into the high temperature heat release region. Higher inlet temperatures (and/or lower pressures) that cause flattening of the P-T curves beyond the 120°C curve can lead to HTHR events. This was experienced sporadically during experiments at 120°C, likely triggered by momentary excursions in inlet pressures and temperatures and/or presence of rich fuel pockets.
In closing, the hypothesis posited at the start of this section is shown to be correct, i.e. inlet heating can be used to create appropriate thermal conditions to maximize the exploitation of the high -sensitivity region. As can be inferred from , strong, positive, linear
sensitivity of around 10 J/0.1
can be realized for mixtures ranging in strength from very lean (
) to rich (
) by increasing mixture temperature. The magnitude of the
-sensitivity does not change significantly for the various mixture temperatures as they are richened, but the onset of negative sensitivity is delayed by increasing temperatures.
Discussion
Some potential implications of the observed iso-octane LTHR behavior and the underlying thermo-chemical influences on engine combustion and control are briefly discussed next.
LTHR reactions are often precursors to high-temperature AI reactions – end gas AI in SI engines or controlled auto-ignition – and LTHR intensity can thus be treated as a predictor of high-temperature AI. Some support for this can be found from non-isolated-LTHR experimental studies by Kalghathi and co-workers (Citation2013, Citation2003) in which fuels with higher ID-based LW scores (i.e. shorter effective ID times) were reported to have a higher propensity to knock in SI engines and promote AI in CAI engines; and from Shibata et al. (Citation2005) who found LTHR and HTHR 50% mass fraction burned points in a CAI engine to be linearly related. This is not to imply that end gas AI always leads to knocking. Optical studies have demonstrated that if in-cylinder thermal states are maintained at relatively mild levels, by e.g. managing in-cylinder flows, end gas AI without high frequency pressure fluctuations typical of knocking can take place (Iijima et al. Citation2013), which is the basis of spark-assisted CAI engines. In fact, LTHR can also have a knock inhibiting effect if it is staged appropriately to exploit the temperature drop induced by NTC reactions (Splitter et al. Citation2019). Thus, depending on the intensity and phasing of LTHR reactions relative to main heat release, they can act to promote, moderate, or inhibit high-temperature AI.
Iso-octane LTHR characterization data at different thermal and chemical conditions presented above () can be used to avoid regions of high-intensity LTHR in SI engines to prevent knock or to control AI in CAI engines. The existence of strong, positive sensitivity and the possibility of extending it via mixture heating can help realize staged combustion via chemical stratification in gasoline engines. The negative
sensitivity region at relatively rich conditions can potentially also be exploited to moderate combustion. Depending on the mixture temperature, the negative
sensitivity region could still be lean of stoichiometric, e.g. 40°C and 60°C cases in , potentially making negative
sensitivity biased chemical stratification viable from a fuel conversion efficiency perspective.
The occurrence of LTHR at lean conditions could be an enabler for stable ultra lean ( (Hu et al. Citation2020)) combustion in SI engines, which is prone to combustion instabilities resulting from cyclic variability during early kernel growth and susceptibility of nascent flames to extinction. If compressed mixture reactivity can be tailored to have pre-spark LTHR reactions, the rise in temperature can make deflagrative combustion more resilient by increasing flame speeds.
Ultra lean LTHR can also potentially be used to consume unburned fuel in dilute end gas mixtures if appropriate thermal conditions can be created. This can improve the combustion efficiency of ultra-lean SI engines and also compensate for slow combustion rates typical of lean deflagrative combustion. Moreover, because the intensity of LTHR is low at lean conditions (), the likelihood of knocking is expected to be low.
Some control strategies, in addition to inlet heating and supercharging, that can help create the needed in-cylinder thermal and chemical conditions to tailor mixture reactivity are listed next. Chemical stratification can be achieved via late and/or multiple injection events, e.g. Alemahdi et al. (Citation2022) and Dec, Yang, and Dronniou (Citation2011) achieved partial fuel stratification by injecting the majority (80–90%) of the fuel early during the intake stroke and the rest, late during the compression stroke. Thermal stratification can be created using different combustion product retention strategies like recompression via negative valve overlap or rebreathing via early/late positive valve overlap (Lang et al. Citation2005). Note, however, that some level of thermal stratification exists naturally in engines because of non-uniformities in energy fluxes, and provides a certain degree of sequential combustion by creating a spatial ID distribution (Dec, Hwang, and Sjöberg Citation2006). Direct injection-induced thermal effects can be managed by changing injection timings, e.g. retarding injection to reduce charge cooling and specific heat ratio effects, of course with associated effects on mixture homogeneity and volumetric efficiency. In SI and spark-assisted CAI engines, the progression of deflagrative combustion can also be managed to generate desired P-T histories of the end gases (Olesky et al. Citation2013). Evaporative cooling from water injection can also be used to change the compression P-T states (Golzari et al. Citation2021). An uncontrollable lever that could impact LTHR in real engines is the occurrence of partially burning cycles, which can increase mixture reactivity in succeeding cycles because of fuel reformation reactions (Sjöberg et al. Citation2004).
Conclusions
The paper explored the LTHR characteristics of iso-octane/air mixtures at different equivalence ratio levels and inlet air temperatures by monitoring changes in LTHR intensity and its phasing. LTHR intensity was indexed by exhaust CO concentration, which was found to be a high sensitivity and reliability signature of LTHR. Major findings of the experimental study are as follows:
LTHR can take place at very lean (
) conditions. It gains strength as the mixture becomes richer and more reactive. However, beyond a certain
limit, in direct injection engines, the thermal cooling effects of fueling overpower reactivity enhancement, and LTHR is quenched.
The non-monotonic behavior of LTHR intensity, phasing, and duration can be used to characterize
-sensitivity at different engine inlet conditions.
Iso-octane/air mixtures exhibit positive or negative
-sensitivities depending on the dominant effect – chemical or thermal. Dominance of the former confers positive sensitivity, while that of the latter produces negative sensitivity and can even render mixtures
-insensitive.
Increasing mixture temperature counters the thermal effects of fuel enrichment and shifts the positive to negative
-sensitivity transition point to higher equivalence ratios and permits more energy release via low temperature reactions. Peak LTHR intensity nearly doubled as the inlet temperatures were increased from 40°C to 120°C, and
increased from
to
.
By appropriately adjusting mixture thermal conditions using thermal control levers (charge cooling and intake heating in this case), strong, positive, linear
sensitivity can be realized across a wide range of
levels.
Nomenclature
AHRR | = | Apparent heat release rate |
AI | = | Auto-ignition |
aTDC | = | After top dead centre |
CA | = | Crank angle |
CA50 | = | CA location at 50% heat release point |
CAI | = | Controlled auto-ignition |
CHR | = | Cumulative heat release |
EGT | = | Exhaust gas temperature |
EoLTHR | = | End of LTHR |
HTHR | = | High temperature heat release |
ID | = | Ignition delay |
IMEP | = | Indicated mean effective pressure |
LTHR | = | Low temperature heat release |
LW | = | Livengood-Wu progress variable |
mot | = | Motored (no fuel) |
NTC | = | Negative temperature coefficient |
P | = | Pressure |
PRF | = | Primary reference fuel |
SI | = | Spark ignition |
SoHTHR | = | Start of HTHR |
SoLTHR | = | Start of LTHR |
t | = | time |
T | = | Temperature |
= | Ratio of specific heats | |
= | Fuel-air equivalence ratio | |
= |
|
Acknowledgements
This research was supported by an Engineering and Physical Sciences Research Council Prosperity Partnership, grant number EP/T005327/1. The Prosperity Partnership is a collaboration between Jaguar Land Rover, Siemens Digital Industries Software, the University of Bath, and the University of Oxford. The authors would also like to thank the Dept. of Engineering Science technicians and maintenance teams for facilities support.
Disclosure statement
No potential conflict of interest was reported by the author(s).
Additional information
Funding
References
- Alemahdi, N., A. García, E. Boufaim, G. Aferiat, and M. Tunér. 2022. Development of an empirical test method to quantify the ϕ-sensitivity of liquid fuels. Energy Convers. Manage. 254:115257. ISSN: 0196- 8904. https://www.sciencedirect.com/science/article/pii/S019689042200053X. doi:10.1016/j.enconman.2022.115257.
- Alemahdi, N., A. García, and M. Tunér. 2022. Understanding the effect of Intake temperature on the ϕ-sensitivity of toluene-ethanol reference fuels and neat ethanol. Int. J. Engine Res. (0):14680874221134147. doi:10.1177/14680874221134147.
- Bajwa, A. U., F. C. P. Leach, and M. H. Davy. 2023. Prospects of controlled auto-ignition based thermal propulsion units for modern gasoline vehicles. Energies 16 (9):3887. ISSN: 1996-1073. doi: 10.3390/en16093887.
- Borgqvist, P., P. Tunestal, and B. Johansson. 2013. Comparison of Negative Valve Overlap (NVO) and Rebreathing valve strategies on a gasoline PPC engine at low load and idle operating conditions. SAE Int. J. Engines 6 (1):366–78. ISSN: 19463936, 19463944. Accessed 11 7, 2022. http://www.jstor.org/stable/26277624.
- Bresenham, D., J. Reisel, and K. Neusen. 1998. Spindt air-fuel ratio method generalization for oxygenated fuels. Sae Trans. 107:2154–71. ISSN: 0096736X, ISSN: 0096736X. Accessed 09 22, 2022. http://www.jstor.org/stable/44736682.
- Collin, R., J. Nygren, M. Richter, M. Aldén, L. Hildingsson, and B. Johansson. 2003. Simultaneous OH- and Formaldehyde-LIF measurements in an HCCI engine. Sae Trans. 112:2479–86. ISSN: 0096736X, ISSN: 0096736X. Accessed 12 31, 2022. http://www.jstor.org/stable/44742465.
- Dec, J. E., W. Hwang, and M. Sjöberg. 2006. An investigation of Ther- mal stratification in HCCI engines using chemiluminescence imaging. Sae Trans. 115:759–76. ISSN: 0096736X, 25771531. Accessed 12 31, 2022. http://www.jstor.org/stable/44687346.
- Dec, J. E., and M. Sjöberg. 2004. Isolating the effects of fuel chemistry on combustion phasing in an HCCI engine and the potential of fuel stratification for ignition control. Sae Trans. 113:239–57. ISSN: 0096736X, 25771531. Accessed 10 26, 2022. http://www.jstor.org/stable/44740754.
- Dec, J. E., Y. Yang, and N. Dronniou. 2011. Boosted HCCI – controlling pressure-rise rates for performance improvements using partial fuel stratification with conventional gasoline. SAE Int. J. Engines. 4(1):1169–89. ISSN: 19463936, 19463944. Accessed 12 31, 2022. http://www.jstor.org/stable/26278213.
- DelVescovo, D. A., D. A. Splitter, J. P. Szybist, and G. S. Jatana. 2020. Modeling pre-spark heat release and low temperature chemistry of iso- octane in a boosted spark-ignition engine. Combust. Flame 212:39–52. ISSN: 0010-2180. https://www.sciencedirect.com/science/article/pii/S0010218019304651. doi:10.1016/j.combustflame.2019.10.009.
- Golzari, R., H. Zhao, J. Hall, M. Bassett, J. Williams, and R. Pearson. 2021. Impact of intake port injection of water on boosted downsized gasoline direct injection engine combustion, efficiency and emissions. Int. J. Engine Res. 22 (1):295–315. doi:10.1177/1468087419832791.
- Hu, Z., J. Zhang, M. Sjöberg, and W. Zeng. 2020. The use of partial fuel stratification to enable stable ultra-lean deflagration-based Spark-Ignition engine operation with controlled end-gas autoignition of gasoline and E85. Int. J. Engine Res. 21 (9):1678–95. doi:10.1177/1468087419889702.
- Iijima, A., M. Tanabe, K. Yoshida, H. Shoji, N. Itoh, A. Terashima, and T. Tojo. 2013. Visualization and spectroscopic measurement of knocking combustion accompanied by cylinder pressure oscillations in an HCCI engine. SAE Int. J. Engines 6 (4):2150–63. ISSN: 19463936, 19463944. Accessed 11 4, 2022. http://www.jstor.org/stable/26272341.
- Kalghatgi, G. Oct. 2013. Fuel/Engine Interactions. SAE International. doi:10.4271/R-409.
- Kalghatgi, G., P. Risberg, and H.-E. Ångstrom. May, 2003. “A method of defining ignition quality of fuels in HCCI engines”. In: 2003 JSAE/SAE International Spring Fuels and Lubricants Meeting. SAE International. doi:10.4271/2003-01-1816.
- Kalghatgi, G., P. Risberg, and H.-E. Ångström. Jan, 2007. Partially pre-mixed auto-ignition of gasoline to attain low smoke and low NOx at high load in a compression ignition engine and comparison with a diesel fuel. 2007 Fuels and Emissions Conference. SAE International. doi:10.4271/2007-01-0006.
- Lang, O., W. Salber, J. Hahn, S. Pischinger, K. Hortmann, and C. Bücker. 2005. Thermodynamical and mechanical approach towards a variable valve train for the controlled auto ignition combustion process. Sae Trans. 114: 722–34. ISSN: 0096736X, 25771531 Accessed 11 7, 2022. http://www.jstor.org/stable/44722038.
- Leach, F. C. P., M. H. Davy, and B. Terry. 2021. Combustion and emissions from cerium oxide nanoparticle dosed diesel fuel in a high speed diesel research engine under low temperature combustion (LTC) conditions. Fuel 288:119636. ISSN: 0016-2361 https://www.sciencedirect.com/science/article/pii/S0016236120326326. doi:10.1016/j.fuel.2020.119636.
- Leppard, W. R. Oct, 1990. The chemical origin of fuel octane sensitivity. International Fuels and Lubricants Meeting and Exposition. SAE International. doi:10.4271/902137.
- Liu, F., M. Z. Akram, and H. Wu. 2020. Hydrogen effect on lean flammability limits and burning characteristics of an isooctane–air mixture. Fuel 266:117144. ISSN: 0016-2361. https://www.sciencedirect.com/science/article/pii/S0016236120301393. doi:10.1016/j.fuel.2020.117144.
- Olesky, L. M., J. B. Martz, G. A. Lavoie, J. Vavra, D. N. Assanis, and A. Babajimopoulos. 2013. The effects of spark timing, unburned gas temperature, and negative valve overlap on the rates of stoichiometric spark assisted compression ignition combustion. Appl. Energ. 105:407–17. ISSN: 0306-2619. https://www.sciencedirect.com/science/article/pii/S0306261913000470. doi:10.1016/j.apenergy.2013.01.038.
- Pan, J., P. Zhao, C. K. Law, and H. Wei. 2016. A predictive Liven- good–Wu correlation for two-stage ignition. Int. J. Engine Res. 17 (8):825–35. doi:10.1177/1468087415619516.
- Papaioannou, N., F. C. P. Leach, M. H. Davy, A. Weall, and B. Cooper. 2019. Evaluation of exhaust gas recirculation techniques on a high-speed direct injection diesel engine using first law analysis. P. I. Mech. Eng. 233 (3):710–26. doi:10.1177/0954407017749110.
- Pintor, D. L., J. E. Dec, and G. R. Gentz. 2019. Φ-sensitivity for LTGC engines: Understanding the fundamentals and tailoring fuel blends to maximize this property. SAE Technical Paper. ISSN: 2019-01-0961. https://www.osti.gov/servlets/purl/1576882.
- Saisirirat, P., F. Foucher, S. Chanchaona, and C. Mounaïm-Rousselle. Sept. 2009. Effects of Ethanol, n-Butanol — n-Heptane Blended on Low Temperature Heat Release and HRR Phasing in Diesel-HCCI. 9th International Conference on Engines and Vehicles. Consiglio Nazionale delle Ricerche. doi:10.4271/2009-24-0094.
- Senecal, K., and F. C. P. Leach. June, 2021. Racing toward zero: The untold story of driving green. SAE International.
- Shahanaghi, A., S. Karimkashi, O. Kaario, V. Vuorinen, T. Sarjovaara, and R. Tripathi. 2022. Temperature stratification induced ignition regimes for gasoline surrogates at engine-relevant conditions. Combust. Sci. Technol (0):1–41. doi:10.1080/00102202.2022.2124511.
- Shibata, G., K. Oyama, T. Urushihara, and T. Nakano. 2005. Correlation of low temperature heat release with fuel composition and HCCI engine combustion. SAE Technical Paper. ISSN: 0148-7191. doi:10.4271/2005-01-0138.
- Sjöberg, M., and J. E. Dec. 2006. Smoothing HCCI heat-release rates using partial fuel stratification with two-stage ignition fuels. Sae Trans. 115:318–34. ISSN: 0096736X, ISSN: 0096736X Accessed 10 26 2022. http://www.jstor.org/stable/44687308.
- Sjöberg, M., J. E. Dec, A. Babajimopoulos, and D. Assanis. 2004. Comparing enhanced natural thermal stratification against retarded combustion phasing for smoothing of HCCI heat-release rates. Sae Trans. 113:1557–75. ISSN: 0096736X, 25771531 Accessed 10 26, 2022. http://www.jstor.org/stable/44723614.
- Splitter, D. A., A. Gilliam, J. Szybist, and J. Ghandhi. 2019. Effects of pre-spark heat release on engine knock limit. Proc. Combust. Inst. 37 (4):4893–900. ISSN: 1540-7489. doi:10.1016/j.proci.2018.05.145.
- Szybist, J. P., and D. A. Splitter. 2017. Pressure and temperature effects on fuels with varying octane sensitivity at high load in SI engines. Combust. Flame 177:49–66. ISSN: 0010-2180. doi:10.1016/j.combustflame.2016.12.002.
- Tao, M., P. Zhao, J. P. Szybist, P. Lynch, and H. Ge. 2019. Insights into engine autoignition: Combining engine thermodynamic trajectory and fuel ignition delay iso-contour. Combust. Flame 200:207–18. ISSN: 0010-2180 https://www.sciencedirect.com/science/article/pii/S0010218018305091. doi:10.1016/j.combustflame.2018.11.025.
- Waqas, M. U., A. Hoth, C. P. Kolodziej, T. Rockstroh, J. Pulpeiro Gonzalez, and B. Johansson. 2019. Detection of low temperature heat release (LTHR) in the standard Cooperative Fuel Research (CFR) engine in both SI and HCCI combustion modes. Fuel 256:115745. ISSN: 0016-2361 https://www.sciencedirect.com/science/article/pii/S001623611931097X. doi:10.1016/j.fuel.2019.115745.
- White, S., A. U. Bajwa, and F. C. P. Leach. 2023. Isolated low temperature heat release in spark ignition engines. SAE Int. J. Adv. Curr. Pract. Mobility. doi:10.4271/2023-01-0235.
- Wu, Y., P. Pal, S. Som, and T. Lu. May, 2017. A skeletal chemical kinetic mechanism for gasoline and gasoline/ethanol blend surrogates for engine CFD applications. 10th International Conference on Chemical Kinetics, University of Illinois at Chicago.
- Yang, Y., J. E. Dec, N. Dronniou, and M. Sjöberg. 2011. Tailoring HCCI heat-release rates with partial fuel stratification: Comparison of two-stage and single-stage-ignition fuels. Proc. Combust. Inst. 33 (2):3047–55. ISSN: 1540-7489. https://www.sciencedirect.com/science/article/pii/S1540748910001999. doi:10.1016/j.proci.2010.06.114.
- Zhao, P., and C. K. Law. 2013. The role of global and detailed kinetics in the first-stage ignition delay in NTC-affected phenomena. Combust. Flame. 160 (11):2352–58. ISSN: 0010-2180 https://www.sciencedirect.com/science/article/pii/S0010218013002241. doi:10.1016/j.combustflame.2013.06.009.