ABSTRACT
Neofusicoccum parvum is one of the most aggressive Botryosphaeriaceae species associated with grapevine trunk diseases. This species may secrete enzymes capable of overcoming the plant barriers, leading to wood colonization. In addition to their roles in pathogenicity, there is an interest in taking advantage of N. parvum carbohydrate-active enzymes (CAZymes), related to plant cell wall degradation, for lignocellulose biorefining. Furthermore, N. parvum produces toxic secondary metabolites that may contribute to its virulence. In order to increase knowledge on the mechanisms underlying pathogenicity and virulence, as well as the exploration of its metabolism and CAZymes for lignocellulose biorefining, we evaluated the N. parvum strain Bt-67 capacity in producing lignocellulolytic enzymes and secondary metabolites when grown in vitro with two lignocellulosic biomasses: grapevine canes (GP) and wheat straw (WS). For this purpose, a multiphasic study combining enzymology, transcriptomic, and metabolomic analyses was performed. Enzyme assays showed higher xylanase, xylosidase, arabinofuranosidase, and glucosidase activities when the fungus was grown with WS. Fourier transform infrared (FTIR) spectroscopy confirmed the lignocellulosic biomass degradation caused by the secreted enzymes. Transcriptomics indicated that the N. parvum Bt-67 gene expression profiles in the presence of both biomasses were similar. In total, 134 genes coding CAZymes were up-regulated, where 94 of them were expressed in both biomass growth conditions. Lytic polysaccharide monooxygenases (LPMOs), glucosidases, and endoglucanases were the most represented CAZymes and correlated with the enzymatic activities obtained. The secondary metabolite production, analyzed by high-performance liquid chromatography–ultraviolet/visible spectophotometry–mass spectrometry (HPLC-UV/Vis-MS), was variable depending on the carbon source. The diversity of differentially produced metabolites was higher when N. parvum Bt-67 was grown with GP. Overall, these results provide insight into the influence of lignocellulosic biomass on virulence factor expressions. Moreover, this study opens the possibility of optimizing the enzyme production from N. parvum with potential use for lignocellulose biorefining.
GRAPHICAL ABSTRACT
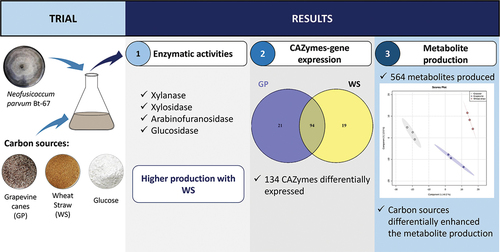
INTRODUCTION
Plant-pathogenic fungi deploy a wide array of cell wall–degrading enzymes (CWDEs) to overcome the host’s physical defenses and utilize their degradation products for growth and reproduction (Kubicek et al. Citation2014). The fungal degradation capacity relies on complex degradative machinery able to catalyze two processes: direct enzymatic depolymerization and generation of oxidative species that act on lignocellulosic biomass (Cragg et al. Citation2015). According to the cell wall polymer that they degrade, the CWDEs are (i) ligninolytic enzymes (e.g., laccases and peroxidases), (ii) cellulolytic enzymes (e.g., endoglucanases, exoglucanases, and β-glucosidases), and (iii) hemicellulolytic enzymes (e.g., endoxylanases, αarabinofuranosidase, β-xylosidase, and others) (Saldarriaga-Hernández et al. Citation2020). The enzymes using carbohydrates as substrates are grouped in carbohydrate-active enzyme (CAZymes) families within five classes: glycoside hydrolases (GHs), glycosyltransferases (GTs), polysaccharide lyases (PLs), carbohydrate esterases (CEs), and auxiliary activities (AAs) (Lombard et al. Citation2014). These enzymes can be accompanied by carbohydrate-binding modules (CBMs), which promote the association of the enzyme with the substrate (Boraston et al. Citation2004). This wide variety of CAZymes in fungal plant pathogens makes them a promissory source for novel lignocellulolytic enzymes (Gibson et al. Citation2011).
Neofusicoccum parvum, included in the Botryosphaeriaceae family (phylum Ascomycota), is a ubiquitous fungus that causes disease in a broad range of plant species (Phillips et al. Citation2013). Its pathogenicity has been confirmed on 90 different hosts, mainly woody angiosperms (Sakalidis et al. Citation2013). Neofusicoccum parvum is one of the most aggressive Botryosphaeriaceae species in grapevine, commonly reported as a causal agent of Botryosphaeria dieback (Mondello et al. Citation2018; Travadon et al. Citation2013; Úrbez-Torres Citation2011; Úrbez-Torres and Gubler Citation2009). This disease, included among the grapevine trunk diseases, significantly affects the productivity of vineyards worldwide (Bertsch et al. Citation2013; Fontaine et al. Citation2016). Neofusicoccum parvum may enter the grapevine through fresh wounds (e.g., pruning wounds) or via natural openings (e.g., lenticels) and colonizes the woody tissues, causing discolored and decayed zones in the wood (Claverie et al. Citation2020; Reis et al. Citation2020). As a wood colonizer, this pathogen secretes enzymes capable of degrading plant cell polymers (Massonnet et al. Citation2018; Stempien et al. Citation2017). Genomic analyses of different N. parvum strains have pointed out that this fungal species possesses an important repertoire of lignocellulolytic enzymes (Belair et al. Citation2023; Blanco-Ulate et al. Citation2013; Massonnet et al. Citation2018; Morales-Cruz et al. Citation2015; Nagel et al. Citation2021; Yu et al. Citation2022). Interestingly, it has been found that N. parvum possesses one of the highest numbers of CAZymes within the Botryosphaeriaceae family (Belair et al. Citation2023; Garcia et al. Citation2021; Nagel et al. Citation2021; Yu et al. Citation2022). Although numerous genomic studies have been conducted for N. parvum, only one has attempted to investigate the CAZyme-coding gene expression profile (Massonnet et al. Citation2018). This study reported 73 putative CAZymes involved in host cell wall degradation when in vitro fungal growth occurred with grapevine wood. Despite these advances, there is a paucity of information on how N. parvum adapts its transcriptome under different conditions. Further, it has been suggested that N. parvum, along with other Botryosphaeriaceae species, is a good candidate for producing biotechnologically relevant CAZymes (Belair et al. Citation2023; Esteves et al. Citation2014). Whether the N. parvum CAZymes can be applied in a biotechnological context and which conditions are conducive to their production still require substantial support.
In addition to the necrosis in the woody tissue of grapevine, the infection by N. parvum also causes foliar symptoms that are characterized by wine-red or yellowish-orange spots on the leaf margins or leaf blade (Larignon et al. Citation2001; Mondello et al. Citation2018). As this fungus has never been isolated from leaves, it is thought that foliar symptoms may be remotely induced (Claverie et al. Citation2020). One hypothesis is that N. parvum may produce phytotoxic compounds that can translocate to leaves via transpiration stream (Bertsch et al. Citation2013). Multiple studies have described and evaluated the toxic secondary metabolites produced by N. parvum (Abou-Mansour et al. Citation2015; Andolfi et al. Citation2011; Evidente et al. Citation2010; Ramírez-Suero et al. Citation2014; Reveglia et al. Citation2019; Salvatore et al. Citation2021; Trotel-Aziz et al. Citation2022). These secondary metabolites are derived from either polyketide precursors or nonribosomal peptides and can interfere with enzymatic reactions and cellular transport, as well as cause damage to the cell membrane in the host (Massonnet et al. Citation2018). Among the different secondary metabolites produced by N. parvum, terremutin and mellein have been considered representative of their respective chemical families, inducing severe necrosis when applied to grapevine leaf disks and in planta (Abou-Mansour et al. Citation2015; Trotel-Aziz et al. Citation2022). Moreover, those two compounds and some of their derivatives were detected in grapevine wood from plants showing Botryosphaeria dieback (Abou-Mansour et al. Citation2015). Besides low-molecular-weight toxins, the virulence of N. parvum might also rely on exopolysaccharides (Martos et al. Citation2008) and necrosis- and ethylene-inducing proteins (Nazar Pour et al. Citation2020).
In order to develop effective strategies to control N. parvum, it is relevant to understand the factors facilitating host colonization, such as the CWDEs and phytotoxic compounds. Here, we evaluated the capacity of N. parvum to produce CAZymes related to plant cell wall degradation and secondary metabolites when growing in liquid culture in contact with two lignocellulosic biomasses. We focused on the strain N. parvum Bt-67 (Estremadura, Portugal; Reis et al. Citation2016) due to its high aggressiveness and ability to produce and secrete enzymes involved in pathogenicity and production of phytotoxic metabolites (Abou-Mansour et al. Citation2015; Belair et al. Citation2023; Ramírez-Suero et al. Citation2014; Stempien et al. Citation2018, Citation2017; Trotel-Aziz et al. Citation2022). As lignocellulosic biomasses, we selected one woody host biomass (grapevine canes) and one non-woody biomass from a non-host (wheat straw), which differ in chemical composition, carbon content, and structure. We hypothesized that the production of CWDEs, related to the expression and the secretion of CAZymes, and secondary metabolites in N. parvum varies according to the lignocellulose biomass composition. In this article, we tested this hypothesis using a multiphasic study combining enzymology, transcriptomic, and metabolomic analyses.
MATERIALS AND METHODS
Fungal strain and cultivation.
—The strain N. parvum Bt-67 was obtained from vineyards of the Estremadura area (Portugal) and registered in the Instituto Superior de Agronomia (University of Lisbon, Portugal). The fungus was grown on potato dextrose broth (PDB; BD, Le Pont-de-Claix, France) with agar (BD) at 28 C in the dark for 7 days before cultivation.
A portion of N. parvum Bt-67 mycelium (0.5 × 1.0 cm) was cultured in 50 mL of liquid minimum medium EP (Eriksson Citation1975). Two lignocellulosic biomasses were used as a carbon source: grapevine canes (GP) (Vitis vinifera cv. Chardonnay, 2–3 mm; collected in Champagne vineyard after winter pruning) and wheat straw (WS) (Apache variety, <2 mm; obtained from ARD, Pomacle-Bazancourt, France), with a concentration of 5 g·L−1. As control, a culture medium using only d-glucose (GLU; Carl Roth, Karlsruhe, Germany) at 10 mM as the carbon source was used. For each growth medium, control flasks were prepared without fungal inoculation. All flasks were incubated at 30 C with agitation at 100 rpm and in triplicates for 7–14 days. After cultivation, mycelia from each growth condition (i.e., EP medium with GP, WS, or GLU) were harvested and stored at −80 C before RNA and secondary metabolite extractions.
Chemical composition of the lignocellulosic biomasses.
—The chemical composition in hemicellulose, cellulose, lignin, and ash of both lignocellulosic biomasses was determined following the Van Soest method (Van Soest and Robertson Citation1980). This method consisted of sequential extractions using a neutral detergent (30 g sodium dodecyl sulfate, 20.61 g ethylenediaminetetraacetic disodium salt dihydrate, 6.81 g disodium tetraborate, 4.56 g disodium hydrogen phosphate anhydrous, and 10 mL ethylene glycol monoethyl ether per liter distilled water), an acid detergent (20 g cetyltrimethyl ammonium bromide and 26.63 mL sulfuric acid at 96% per liter distilled water), and sulfuric acid at 72%. The wheat straw chemical composition was previously determined by Cassarini et al. (Citation2022).
Enzymatic activity assays.
—Extracellular enzymes (secretome) were obtained by collecting 5 mL of supernatant from each fungal culture at two time points, 7 (T7) and 14 (T14) days of incubation. Enzymatic activity assays were measured in triplicates. Xylanase activity was estimated by measuring reducing sugars produced from beechwood xylan (Carl Roth) at 0.5% w/v mixed with 0.1 mL of the secretome in Tris-HCl 25 mM, pH 7, at 30 C for 10 min (Kidby and Davidson Citation1973). β-d-Xylosidase, α-l-arabinofuranosidase, and β-d-glucosidase activities were evaluated by determining the hydrolysis rate of p-nitrophenyl-β-d-xylopyranoside (Carbosynth, Compton, UK), p-nitrophenyl-α-l-arabinofuranoside (Sigma, St. Louis, MI, USA), and p-nitrophenyl-β-d-glucopyranoside (Carbosynth), respectively, to p-nitrophenol (pNP). Each reaction was performed in 1 mL containing 0.1 mL of secretome, 0.45 mL of substrate (10 mM), and 0.45 mL of buffer (Tris-HCl 25 mM, pH 7) for 5 min at 30 C. The extinction coefficient of pNP was 10 625 L·mol−1·cm−1 (Cassarini et al. Citation2021). Total peroxidase activities were quantified as described by Bach et al. (Citation2013). In brief, 0.1 mL of secretome was mixed with 0.1 mL of pyrogallol (PYGL; 160 mM) in ethylenediaminetetraacetic acid (EDTA; 20 mM), 0.1 mL of H2O2 (10 mM), and 1.7 mL of Tris-HCl 25 mM, pH 7, for a final reaction volume of 2 mL. For estimation of laccases activities, 0.1 mL of syringaldazine (SGZ; 0.5 mM in ethanol 96%) was mixed with 0.1 mL of secretome and buffer (Leonowicz and Grzywnowicz Citation1981). The reactions were at 30 C for 10 min. Endoglucanase activity was evaluated according to Rahman et al. (Citation2018). A series of enzymatic dilutions were prepared to determine the enzyme concentration capable of releasing 0.5 mg of glucose. For this, 0.5 mL of carboxymethyl cellulose (CMC; Sigma, St. Louis, MI, USA) solution (2% w/v in Tris-HCl 25 mM, pH 7) was mixed with 0.5 mL of each enzymatic dilution and incubated at 30 C. After 30 min, 2 mL of 3,5-dinitrosalicylic acid (DNS) reagent was added and the reaction was stopped in boiling water for 5 min. The values from “enzyme blank” (without CMC) and “substrate blank” (without enzymatic dilutions) were subtracted from the absorbance of each sample. Absorbance for xylose and PYGL was measured at 420 nm. For pNP, SGZ, and glucose derived from CMC, the wavelengths used were 401, 530, and 540 nm, respectively. All the spectrophotometric measurements were performed using a SPECORD 200 PLUS spectrometer (Analytik Jena, Jena, Germany).
Enzymatic activities were expressed in units (IU) per milliliter, where 1 IU was defined as the quantity of enzyme required to release 1 µmol of equivalent xylose (for xylanase activity), 1 µmol of pNP (for β-d-xylosidase, α-l-arabinofuranosidase, and β-d-glucosidase activities), or 1 µmol of degraded PYGL or SGZ (for peroxidase/laccase activities) per minute. Endoglucanase activity was expressed as unit (IU) of CMCase per milliliter, where 1 IU corresponds to the amount of enzyme able to release 1 µmol of glucose equivalent per minute from the substrate.
Substrate relative degradation analyses by ATR-FTIR.
—After cultivation, the plant biomass (GP and WS) was separated from the culture medium by filtration and placed in 50-mL Falcon tubes. Two successive washes with 5 mL of Milli-Q water followed by centrifugation were performed. Agitation by either pumping or vortexing was necessary when the mycelium was attached to the biomass. The samples were dried in ovens at 50 C for at least 4 days. The Attenuated total reflectance Fourier transform infrared (ATR-FTIR) spectroscopy was used to analyze the chemical bonds in GP and WS before and after N. parvum Bt-67 cultivation. ATR-FTIR was performed using a Nicolet 6700 (Thermo Fisher Scientific, Madison, WI, USA) spectrometer and a UATR Two (PerkinElmer, Norwalk, CT, USA) measurement module. The measurements were performed in the wavenumber range of 4000–500 cm−1 with a resolution of 4 cm−1. Each sample was measured three times. A mean spectrum from the three readings was calculated, the baseline was corrected using OMNIC software, and the peak heights were analyzed using TQ Analyst EZ Edition. The area of interest (1800–800 cm−1) was normalized. Ratios of absorbance of biomass in the absence (control) and presence of N. parvum Bt-67 were calculated. The measurements were performed from microbial growth triplicate assays.
Transcriptomic analysis.—
RNA extraction
Frozen mycelia from three replicates (7 days of growth on EP medium with GP, WS, or GLU) were used for RNA extraction. Total RNAs were extracted as described in Chang’s protocol, with minor modifications (Chang et al. Citation1993). For each sample, 200 mg of frozen tissue was ground to a fine powder in liquid nitrogen, lysed with 900 µL of cetyltrimethylammonium bromide (CTAB) extraction buffer, and then chloroform:isoamyl alcohol (24:1) was used to dissociate RNAs from proteins. After overnight precipitation with ¼ volume of 10 M LiCl, RNAs were collected and dissolved in RNase/DNase-free water. Then, total RNAs were purified with RNeasy Mini Kit (Qiagen, Hilden, Germany) according to the manufacturer’s recommendations. Treatment with RNase-free DNase I was applied during this purification step to ensure the elimination of genomic DNA. The quantity of RNA extracts was measured with a NanoDrop One spectrophotometer (Thermo Fisher, Madison, WI, USA), and quality was checked on Experion RNA StdSens Analysis Kit (Bio-Rad, Hercules, CA, USA), according to the manufacturer’s recommendations.
RNA-seq analysis
For the transcriptomic analysis, extracted total RNAs were sent to Novogene (Cambridge, UK). cDNA libraries were prepared and sequenced on an Illumina NovaSeq 6000 system (San Diego, CA, USA). The read quality was checked using FastQC (Andrews Citation2010). The high-quality reads were mapped to N. parvum UCRNP2 (Blanco-Ulate et al. Citation2013) using HISAT2 (Kim et al. Citation2015). The expression of transcripts was assessed by StringTie (Pertea et al. Citation2016). FPKM (fragments per kilobase of transcript sequence per million mapped reads) was used for estimating gene expression level. Gene Ontology (GO) analysis for significantly expressed transcripts (P-adjust < 0.05) was performed. First, the number of genes for each GO term was calculated by mapping all source genes in the GO database (http://www.geneontology.org/; Ashburner et al. Citation2000), then significantly enriched GO terms in source genes were estimated using Wallenius noncentral hypergeometric distribution. R package ballgown (Frazee et al. Citation2015) was used to determine differentially expressed genes. Genes with P < 0.05 (parametric F-test) and a log2(fold change) > 2 or < −2 were considered to be differentially expressed. Annotation of differentially expressed genes (DEGs) coding for carbohydrate-active enzyme (CAZyme) families was performed according to the CAZy database (http://www.cazy.org/; Lombard et al. Citation2014) and by using the dbCAN2 server (Zhang et al. Citation2018). Venn diagrams were created with Venny 2.1 (https://bioinfogp.cnb.csic.es/tools/venny/index.html; Oliveros Citation2007).
Secondary metabolite analysis.
—Two hundred milligrams of frozen mycelia (7 days of growth on EP medium with GP, WS, or GLU) was freeze-dried for 24 h. Freeze-dried samples were extracted with 1 mL methanol (MeOH). After centrifugation (2 min at 13 200 rpm), 20 µL of supernatant was analyzed by high-performance liquid chromatography–mass spectrometry (HPLC-MS; series 1200; Agilent, Waldbronn, Germany) equipped with a ultraviolet diode array detector (UV-DAD) and a coupled liquid chromatograph/mass selective detection (LC/MSD) trap atmospheric pressure chemical ionization mass spectrometer with positive and negative polarization. Methods were applied as described previously (Buckel et al. Citation2017).
Secondary metabolite analysis was performed using MetaboAnalyst 5.0 (Pang et al. Citation2021). First, raw MS data were aligned using built-in XCMS program following the automatic parameter adjustment algorithm. No correction for missing values and no filtering were necessary (all signatures were initially kept). The metabolic signatures detected within the biomass conditions and undetected in the GLU condition were removed, resulting in a list of 564 metabolic signatures (see SUPPLEMENTARY TABLE 3) in order to avoid segregation of the conditions via biomass compounds. The list of 564 metabolic signatures was later submitted to sum normalization and Pareto scaling before further statistical analysis.
Statistical analysis.
—For the enzymatic activities, significant differences among conditions were determined by one-way analysis of variance (ANOVA; P < 0.05) at each time point. Tukey’s honestly significant difference (HSD) tests (P < 0.05) were performed for multiple comparisons of means. One-way ANOVA was also used to determine significant differences (P < 0.05) in the substrate degradation analysis between the absorbance from the lignocellulosic biomass with N. parvum growth and the control group (without fungal growth). The secondary metabolite statistical analysis was performed using MetaboAnalyst 5.0. To inspect sample groupings, a principal component analysis (PCA) was performed. Subsequently, a partial least squares discriminant analysis (PLS-DA) was used to enhance discrimination between groups of samples. Q2 was determined to assess the predictability of the model.
Data availability.
—Raw transcriptome data have been deposited to the National Center for Biotechnology Information (NCBI) Sequence Read Archive (SRA; https://www.ncbi.nlm.nih.gov/sra) under accession number PRJNA852183.
RESULTS
Chemical composition of grapevine canes and wheat straw.
—The grapevine material used in this study contained (in percentage of dry matter) 18.2 ± 0.7% of solubles (including pectins, gums, galactans, soluble proteins, etc.), 20.1 ± 0.8% of hemicellulose, 39.2 ± 0.7% of cellulose, 21.6 ± 0.8% of lignin, and 0.2 ± 0.1% of ash. On the other hand, wheat straw contained 10.4 ± 1.5% of solubles, 31.1 ± 1.2% of hemicellulose, 47.4 ± 0.2% of cellulose, 8.5 ± 0.9% of lignin, and 2.2 ± 0.2% of ash (Cassarini et al. Citation2022).
Enzymatic activities.
—Activities of extracellular enzymes (secretome) acting on xylans (xylanases, xylosidase, and arabinosidase), glucans (endoglucanases and glucosidase), and lignin (peroxidases and laccases) were evaluated. Not all the enzyme activities were observed. In particular, no activity was detected for peroxidases and laccases, whereas endoglucanase activities were <0.02 IU·mL−1 when N. parvum grew with the lignocellulosic biomasses (WS and GP) and null with GLU.
Xylanase activities were higher when N. parvum Bt-67 grew in the presence of WS than GP (). In both biomass growth conditions, the enzymatic activity was higher at T14. For WS, xylanase activity levels were 1.30 ± 0.49 and 1.64 ± 0.23 IU·mL−1 at T7 and T14, respectively. In contrast, N. parvum Bt-67 showed a low xylanase activity when cultured with GP, with values of 0.18 ± 0.04 and 0.24 ± 0.03 IU·mL−1. Secretome from the GLU condition did not present any measurable activity. The β-d-xylosidase activity was significantly higher in the WS condition than for the GP secretome at the two time points (). In both lignocellulosic biomass conditions, the β-d-xylosidase activity increased over time. For T7 and T14, the activity levels for WS were 4.08 ± 1.22 and 10.04 ± 1.34 IU·mL−1, respectively, whereas GP showed activity values of 0.89 ± 0.27 and 1.80 ± 0.37 IU·mL−1 at the respective time points. No β-d-xylosidase activity was detected for the GLU condition. Like xylanase and β-d-xylosidase activities, α-l-arabinofuranosidase activity was higher in the case of WS than for the GP secretome, particularly at T14 (). At T7, α-l-arabinofuranosidase activity reached 0.51 ± 0.65, 0.19 ± 0.24, and 0.21 ± 0.18 IU·mL−1 for WS, GP, and GLU, respectively. No significant differences were found at this time point. At T14, α-l-arabinofuranosidase activities increased in the lignocellulosic biomass conditions but decreased in the GLU condition, showing 0.99 ± 0.13, 0.19 ± 0.04, and 0.07 ± 0.02 IU·mL−1 for WS, GP, and GLU, respectively. It should be noted that the variability in all the conditions was high at T7; however, it turned to low values at T14. β-d-Glucosidase was the highest enzymatic activity in N. parvum Bt-67 regardless of the type of lignocellulosic biomass. This enzymatic activity was significantly higher in WS, showing 6.53 ± 0.81 and 16.21 ± 0.98 IU·mL−1, respectively, for T7 and T14, whereas GP presented 4.84 ± 0.70 and 8.11 ± 0.54 IU·mL−1, respectively (). No β-d-glucosidase activity for the GLU condition was measured at T7, although a weak activity was detected at T14 (0.39 ± 0.36 IU·mL−1).
Figure 1. Enzymatic activities of Neofusicoccum parvum Bt-67 secretomes when grown on minimal media with grapevine canes (GP), wheat straw (WS), or glucose (GLU) at 7 and 14 days after inoculation. (A) Xylanase, (B) β-d-xylosidase, (C) α-l-arabinofuranosidase, and (D) β-d-glucosidase activities (IU·mL−1). Blue, red, and gray lines represent secretome activities from the GP, WS, and GLU conditions, respectively. All the measurements were performed in biological triplicates. Error bars indicate standard deviation. Different letters indicate significant differences among groups at each time point (Tukey’s HSD test; P < 0.05).
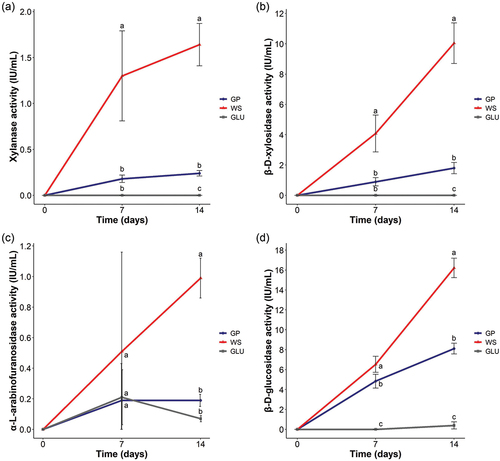
Evaluation of biomass degradation by ATR-FTIR.
—Attenuated total reflectance Fourier transform infrared (ATR-FTIR) spectroscopy analysis was performed in order to evaluate the chemical degradation of the lignocellulosic biomasses by the action of N. parvum Bt-67. shows the chemical bonds at the characteristic peaks (wavenumber) as well as the polymer potentially represented. The degradation ratios for each lignocellulosic biomass (GP and WS) in the absence (control) and presence of N. parvum Bt-67 are shown in . Ratios below 1 represent a relative degradation of the carbon bond. Contrarily, ratios above 1 represent a relative enrichment of the carbon bond due to the degradation of other carbon bonds.
Figure 2. Degradation ratios of chemical bonds representative of grapevine canes and wheat straw determined by attenuated total reflectance Fourier transform infrared (ATR-FTIR) spectroscopy at 14 days after inoculation. Ratios correspond to the value obtained from the lignocellulose biomass with fungal growth over lignocellulose biomass without fungal growth. Bond vibration: ν (stretching), δ (bending), s (symmetric), a (asymmetric). All the measurements were performed in biological triplicates. Error bars indicate standard deviation. Asterisk stands for chemical bonds significantly degraded or enriched after fungal growth (one-way ANOVA; P < 0.05; see also SUPPLEMENTARY FIGS. 1 and 2).
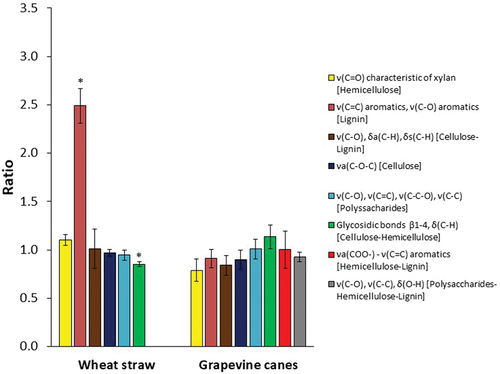
Table 1. Remarkable absorption bands in the attenuated total reflectance Fourier transform infrared (ATR-FTIR) spectra of wheat straw (WS) and grapevine canes (GP) (Adapa et al. Citation2009; Álvarez et al. Citation2020; Levasseur et al. Citation2013; Viel et al. Citation2018; Xu et al. Citation2013).
For WS, a significant degradation value was found for β1-4, bending C–H glycosidic bonds, representative of cellulose and hemicellulose (0.85 ± 0.03) (SUPPLEMENTARY FIG. 1B). In contrast, significant enrichment was found for stretching C=C and C–O bonds, representatives of aromatics (2.49 ± 0.18) (SUPPLEMENTARY FIG. 1F). The other ratios related to WS did not vary significantly. Regarding GP, degradation values were found for stretching C=O (hemicellulose), stretching C=C and C–O (lignin), stretching C–O, bending asymmetric C–H, and bending symmetric C–H (cellulose and lignin), stretching asymmetric C–O–C (cellulose), and stretching C–O, stretching C–C, and bending O–H (polysaccharides, hemicellulose, and lignin) bonds, with ratios of 0.79 ± 0.12, 0.91 ± 0.09, 0.84 ± 0.10, 0.90 ± 0.10, and 0.93 ± 0.05, respectively. Conversely, enrichment was observed for β1-4, bending C–H glycosidic bonds (cellulose and hemicellulose), showing a ratio of 1.10 ± 0.05. However, these values of degradation or enrichment between the groups with the presence of N. parvum Bt-67 and the absence of fungal growth were not significantly different (SUPPLEMENTARY FIG. 2).
Gene expression of N. parvum Bt-67 grown in presence of grapevine canes, wheat straw, and glucose.
—In total, 4156 genes were significantly expressed (P < 0.05). Based on these expressed genes, a heatmap together with hierarchical clusters was created (). This revealed that gene expression of N. parvum Bt-67 was segregated according to the carbon source (WS, GP, or GLU). Moreover, replicates of the same carbon source condition were clustered together, showing robustness in the experiment.
Figure 3. Heatmap and hierarchical clusters of significantly expressed genes of Neofusicoccum parvum Bt-67 grown with different carbon sources. GP = grapevine canes; WS = wheat straw; GLU = glucose. Numbers after carbon source treatments indicate biological repetitions. The scale from red to blue represents the log10(FPKM+1) value, where red indicates high expression levels and blue low expression levels. FPKM = fragments per kilobase of transcript sequence per millions mapped reads.
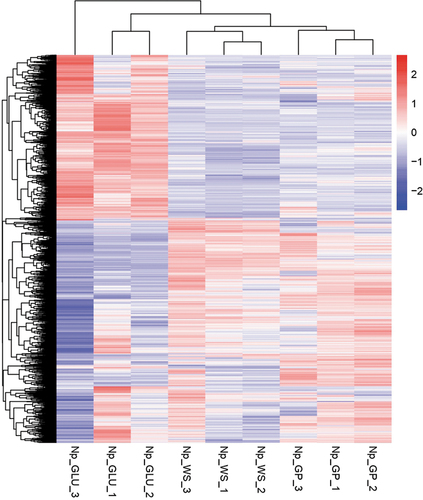
Gene Ontology (GO) of significantly expressed transcripts
In order to correlate biological functions and metabolic pathways (GO categories) with the significantly expressed genes, an enrichment analysis was performed with the data sets from N. parvum Bt-67 grown with GP and WS, comparing each with the control (GLU condition) data sets. For induced genes in the GP condition, the most relevant enrichments were hydrolase activity acting on glycosyl bonds (GO:0016798) and carbohydrate metabolic process (GO:0005975) (). Similarly, the most significant enrichment for the WS condition was hydrolase activity acting on glycosyl bonds (GO:0016798) (). In addition, the WS condition also showed significant enrichment for ribosome (GO:0005840), structural constituent of ribosome (GO:0003735), and structural molecule activity (GO:0005198). In contrast, induced genes in the GLU condition showed enrichment for isomerase activity (GO:0016853), generation of precursor metabolites and energy (GO:0006091), kinase activity (GO:0016301), and nucleobase-containing compound catabolic process (GO:0034655), when compared with GP, and enrichment for nucleobase-containing compound catabolic process (GO:0034655) and generation of precursor metabolites and energy (GO:0006091), when compared with WS.
Figure 4. Gene ontology (GO) classifications for up-regulated (Up) and down-regulated (Down) genes and metabolic pathways in Neofusicoccum parvum Bt-67 when grown in presence of (A) grapevine canes (GP) or (B) wheat straw (WS). Comparisons were performed against gene expression of growth with glucose (GLU). Asterisk denotes statistical significance (P-adjust < 0.05). Significance was calculated using Wallenius noncentral hypergeometric distribution.
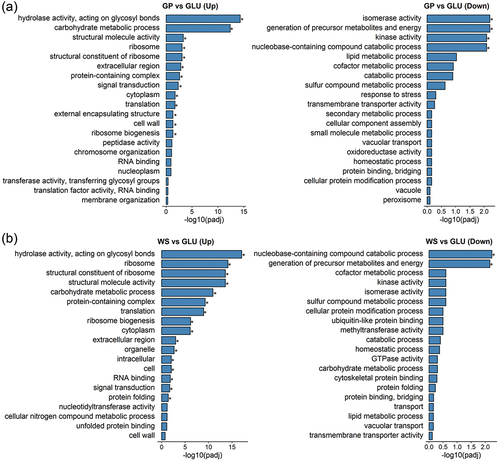
Differentially expressed genes (DEGs)
Following the identification of the biological function for significantly expressed genes, a differentially expressed gene (DEG) analysis was performed, where the growth in presence of glucose was used as the reference. As result, 1302 genes were identified as DEGs (P < 0.05; log2(fold change) > 2 or < −2) for GP (493 up-regulated and 809 down-regulated) and 1282 for WS (455 up-regulated and 827 down-regulated) with respect to reference (SUPPLEMENTARY FIG. 3). Out of the up-regulated genes, 222 and 184 were specific for GP and WS, respectively, whereas 356 and 374 genes were specifically down-regulated for GP and WS, respectively ().
Figure 5. Venn diagrams of the differentially expressed genes (DEGs) in Neofusicoccum parvum Bt-67 grown in presence of different lignocellulosic biomasses. GP = grapevine canes; WS = wheat straw. Growth with glucose was used as control. The number in each circle indicates the number of DEGs for each condition. The overlapping number indicates the number of DEGs in both conditions.
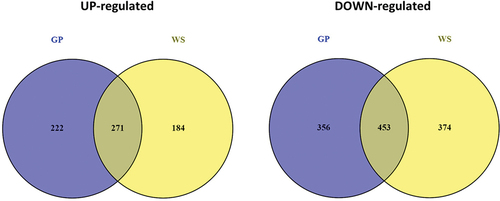
Among the up-regulated genes in the GP condition, the top five encoded for three unknown proteins (fold change = 17.09, 10.67, and 9.80 for ranks 1, 3, and 5, respectively), an endo-xylogalacturonan hydrolase A (Q9UUZ2; fold change = 11.75), and a putative glycoside hydrolase active on cellulose (Q00023; fold change = 10.14) (SUPPLEMENTARY TABLE 1). For up-regulated genes in the WS condition, the top five was represented by two unknown proteins (fold change = 11.18 and 9.82 for ranks 2 and 5, respectively), two previously highly expressed proteins in GP, which are an endo-xylogalacturonan hydrolase A (Q9UUZ2; fold change = 12.52) and a putative glycoside hydrolase active on cellulose (Q00023; fold change = 10.74), and a rhamnogalacturonan acetylesterase (Q00017; fold change = 10.07). Notably, these genes were related to carbohydrate-active enzymes (see Differentially expressed CAZymes). In contrast, out of the top five down-regulated genes in GP, it was only possible to annotate one gene, which encodes for a zinc-regulated transporter (O94639; fold change = −11.67). For WS, the top five down-regulated genes encoded for two unknown proteins (fold change = −13.85 and −12.59 for ranks 2 and 5, respectively), an alpha/beta (AB) hydrolase superfamily protein C1039.03 (Q9US38; fold change = −16.80), a cell wall protein ECM33 (fold change = −11.81), and an E3 ubiquitin protein ligase ZNRF3 (fold change = −11.33). None of these down-regulated genes were related to the CAZy classification.
Differentially expressed CAZymes
Out of 493 up-regulated genes in the GP condition, 115 genes encoded for CAZymes, including 20 auxiliary activities (AAs), 12 carbohydrate esterases (CEs), 70 glycoside hydrolases (GHs), one glycosyltransferase (GT), and 12 polysaccharide lyases (PLs) (SUPPLEMENTARY TABLE 2). Among these 115 CAZyme genes, 79 presented signal peptides, suggesting that these enzymes may be secreted. The most represented families were GH5 and AA9 with 9 genes each, followed by GH43 and GH3 with 8 and 6 genes, respectively. Some of these genes were also associated with carbohydrate-binding modules (CBMs), for example, one GH5_4 was linked to a CBM46 (gene ID = UCRNP2_7122). For the WS condition, 113 up-regulated genes encoded for CAZymes, including 20 AAs, 10 CEs, 69 GHs, one GT, and 13 PLs. Among them, 74 had signal peptides. AA9 and GH3 were the most represented CAZymes families with 9 genes each, followed by GH43 and GH5 with 7 genes each. In total, 94 up-regulated CAZymes were expressed under both conditions; moreover, 21 and 19 up-regulated CAZyme genes were specifically expressed in GP and WS conditions, respectively (; ).
Figure 6. Venn diagram of up-regulated genes encoding carbohydrate-active enzymes (CAZymes) in Neofusicoccum parvum Bt-67 grown in presence of different lignocellulosic biomasses. GP = grapevine canes; WS = wheat straw. Growth with glucose was used as control. The number in each circle indicates the number of up-regulated CAZyme genes for each condition. The overlapping number indicates the number of up-regulated CAZyme genes in both conditions.
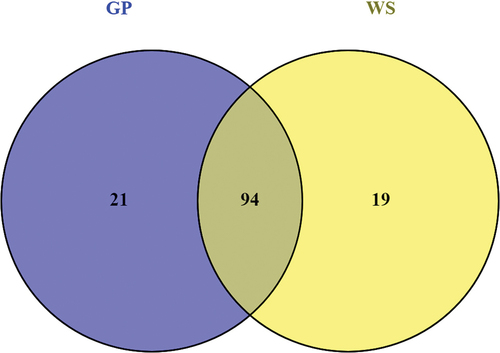
Table 2. Differentially expressed CAZymes (P < 0.05 and log2(fold change) > 2) of Neofusicoccum parvum Bt-67 during growth on minimal media containing grapevine canes (GP) or wheat straw (WS) relative to glucose (GLU).
Interestingly, under the two growth conditions (GP and WS), the four CAZymes families with the highest representation were the same, i.e., AA9, GH3, GH5, and GH43. These groups comprise cellulolytic (AA9s, GH3s, and GH5s) and hemicellulolytic (GH43s, but also some GH3s and GH5s) enzymes. The AA9 proteins are copper-dependent lytic polysaccharide monooxygenases (LPMOs). Almost all of the GH3s were annotated as β-glucosidase; in addition, a GH3 exo-1,4-β-xylosidase was only up-regulated in WS. GH5s comprise mainly endo-β-1,4-glucanases. In the GP condition, an additional putative glucan endo-1,6-β-glucosidase (GH5 subfamily 15) was identified. For GH43 enzymes, we identified a xylosidase/arabinosidase, an α-l-arabinofuranosidase, two exo-β-1,3-galactanases, one of which having a CBM family 32, a putative arabinan endo-1,5-α-l-arabinosidase, a putative exo-α-l-arabinofuranosidase, and an unspecific GH43 subfamily 29 enzyme. Moreover, an α-l-arabinofuranosidase (GH43 subfamily 36) was only expressed in the GP condition. Additional cellulolytic enzymes, such as cellobiohydrolases (GH6 and 7), and hemicellulolytic enzymes, such as xylanases (GH10, 11, 30), xyloglucan-specific endo-β-1,4-glucanase (GH12), β-mannosidase (GH2), acetylxylan esterases (CE1 and 5), and others, were found.
Regarding lignin-modifying enzymes, a laccase (AA1), two peroxidases (AA2), and two dehydrogenases (AA3) were differentially up-regulated for the GP condition (). In presence of WS, only three AA3 enzymes were differentially up-regulated. In addition, one peroxidase (AA2) and two dehydrogenases (AA3) were up-regulated in both biomass growth conditions. Furthermore, we also found up-regulation of pectinases, such as endo- and exo-polygalacturonases (GH28s), and different PLs (PL1, 3, 4, and 9). Interestingly, one endo-xylogalacturonan hydrolase (UCRNP2_438; GH28) was the most expressed CAZyme in both GP and WS, with fold rates of 11.7521 and 12.5253, respectively.
Secondary metabolite production.
—In total, 669 metabolites were detected among the three growth conditions. The metabolites associated with the lignocellulosic biomasses (e.g., ε-viniferin and vitisin C for GP) and undetected in the GLU condition were removed, resulting in a list of 564 compounds (SUPPLEMENTARY TABLE 3). Thirty-five out of 564 metabolites were only present when the fungus grew with the lignocellulosic biomasses, four were unique for the GLU condition, and 510 were produced in the three conditions (). Based on the metabolite peak areas, a principal component analysis (PCA) and a partial least squares discriminant analysis (PLS-DA) were performed. In the PCA, the first two components explained 65.4% of the variance, where PC1 accounted for 44.6% and PC2 for 20.8% (). In the PLS-DA, the first two components explained 56.8% of the variance, where component 1 accounted for 44.2% and the component 2 for 12.6% (). The Q2 was 0.75, indicating a good prediction ability. These analyses showed that the samples segregated according to the carbon source (GP, WS, or GLU).
Figure 7. Venn diagrams of metabolites produced by Neofusicoccum parvum Bt-67 grown in minimal media with grapevine canes (GP), wheat straw (WS), or glucose (GLU) as carbon sources. A. Total number of metabolites in the three conditions. B–D. The number of significantly different metabolites between each pair of conditions: GP vs. WS, GP vs. GLU, and GLU vs. WS. Significantly different metabolites were determined by one-way ANOVA (FDR < 0.05). Overlapping numbers indicate metabolites commonly produced in the compared conditions.
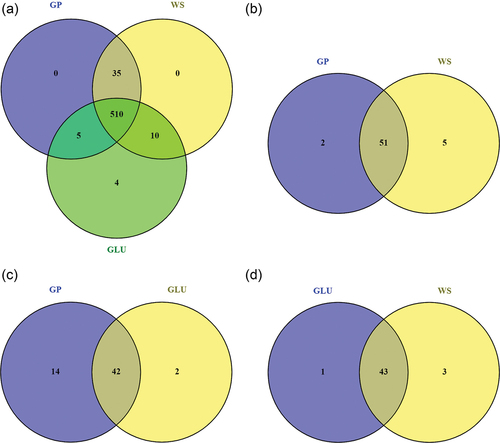
Figure 8. (A) Principal component analysis (PCA) and (B) partial least squares discriminant analysis (PLS-DA) based on the peak area of 564 metabolites produced by Neofusicoccum parvum Bt-67 grown in minimal media with different carbon sources: grapevine canes (blue), wheat straw (red), or glucose (gray). The percentage of variation of each component is indicated at each axis. Ellipses represent the 95% confidence interval. Q2 for the PLS-DA was 0.75.
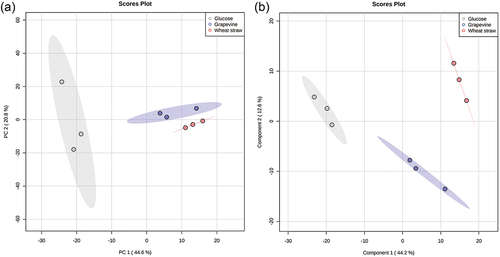
To determine which metabolites were the most discriminant for the growth conditions, one-way ANOVA was performed. As result, 166 out of 564 compounds were significantly different (FDR < 0.05; SUPPLEMENTARY TABLE 3). In addition, a heatmap was created with the top 50 most significant metabolites (SUPPLEMENTARY FIG. 4). This heatmap confirmed the results obtained by PCA and PLS-DA, showing that the biological replicates were grouped. The two most discriminant compounds (FDR < 0.05) were identified as bisnorcholic acid (m/z = 381; C22H36O5), followed by 10′-apo-β-caroten-10′-al (m/z = 415; C27H36O), an unknown compound (m/z = 521), and 2-hydroxy-4,7-dimethoxy-2H-1,4-benzoxazin-3(4H)-one (HDMBOA) (m/z = 226; C10H11NO5) ().
Table 3. The five most discriminant metabolites (FDR < 0.05) produced by Neofusicoccum parvum Bt-67 during growth on minimal media containing grapevine canes, wheat straw, or glucose.
One-way ANOVA was performed for each pair of conditions (i.e., GP-WS, GP-GLU, and WS-GLU). Comparison between GP and WS showed 58 metabolites significantly different (FDR < 0.05), of which two were specifically produced in the GP condition and five in the WS condition (). Between GP and GLU, 58 compounds presented significant differences, in which 14 were specific for GP and two for GLU (). Between GLU and WS, 47 compounds presented significant differences, of which one was specific for GLU and three for WS (). Subsequently, differentially produced metabolites were determined by considering a log2(fold change) > 2 or < −2 and an FDR < 0.05 (SUPPLEMENTARY FIG. 5). In the comparison between GP and WS, 30 metabolites were more abundant in the GP condition, whereas 17 were highly produced in the WS condition. For GP vs. GLU, 40 metabolites were more abundant in the GP, whereas 16 were highly produced in the GLU condition. For WS vs. GLU, 27 metabolites were more abundant in the WS condition, whereas 19 were highly produced in the GLU condition.
displays the top 10 differentially produced compounds in each growth condition. The complete list of differentially produced metabolites is shown in SUPPLEMENTARY TABLE 4.
Table 4. The top 10 differentially metabolites produced by Neofusicoccum parvum Bt-67 in comparison of each pair of carbon source conditions: grapevine canes (GP), wheat straw (WS), and glucose (GLU).
DISCUSSION
Neofusicoccum parvum Bt-67 produces a broad set of CAZymes when grown in presence of lignocellulosic biomass.
—In this work, we aimed to evaluate the capacity of N. parvum Bt-67 in producing CAZymes during growth on woody host (grapevine canes [GP]) and non-woody non-host substrates (wheat straw [WS]). Our results showed that this pathogenic fungus could express a wide variety of CAZymes. These CAZymes mainly targeted cellulose and hemicellulose; however, a significant number of pectinases were also identified. This is in line with Massonnet et al. (Citation2018), who observed that the presence of ground wood from grapevine (cv. Cabernet Sauvignon or cv. Merlot) in agar culture medium induced the expression of CAZymes in N. parvum isolate UCD646So. These authors identified 73 putative CAZymes involved in host cell wall degradation, mainly hemicellulases and pectinases. Here, we present 115 highly expressed CAZymes when growth occurred in presence of grapevine, in which CAZymes targeting hemicelluloses were the most abundant, followed by cellulolytic enzymes (; SUPPLEMENTARY TABLE 2). Besides lignocellulolytic enzymes expressed with the host material (GP), we also showed that N. parvum has the potential to produce CAZymes in presence of non-host material, such as wheat straw.
Our transcriptomic analysis revealed that similar sets of CAZymes were expressed when N. parvum Bt-67 grew in presence of GP and WS. A similar phenomenon has been observed in other wood decay fungi during growth on different substrates (Gaskell et al. Citation2016; MacDonald et al. Citation2011; Van den Wymelenberg et al. Citation2011; Wu et al. Citation2021). Notably, the most abundant up-regulated CAZymes families in N. parvum Bt-67 when grown on either GP or WS included LPMOs (AA9s), β-glucosidases (GH3s), endoglucanases (GH5s), and different GH43 enzymes, such as xylosidase and arabinosidase. These results match with genomic analyses, which indicated that those CAZymes families are the most abundant in N. parvum (Belair et al. Citation2023; Massonnet et al. Citation2018; Morales-Cruz et al. Citation2015). Significant expression of GH3, GH5, and AA9 enzymes has also been observed in wood decay Basidiomycetes (Eastwood et al. Citation2011; Umezawa et al. Citation2020). The predominance of GH5 endoglucanases, GH3 β-glucosidases, and AA9 LPMOs may lie in their ability to degrade cellulose, the main polymer in the plant cell wall. These enzymes, together with cellobiohydrolases (GH6s and GH7s), act synergistically on cellulose to raise d-glucose (Kubicek and Kubicek Citation2016; Saldarriaga-Hernández et al. Citation2020). It is noteworthy that three cellobiohydrolases (one GH6 and two GH7s) were also highly expressed in N. parvum Bt-67 in both lignocellulosic biomass growth conditions.
In order to successfully colonize plant tissue, pathogens must be able to degrade hemicellulose, which contributes to cell wall rigidity and, therefore, plant resistance (Miedes et al. Citation2014). Here, most of the CAZymes in N. parvum Bt-67 transcriptome were hemicellulolytic enzymes, including xylanases (GH10 and 11), xylosidases (GH3 and 43), arabinosidases (GH43, 62, and 146), acetylxylan esterases (CE1 and 5), and others. These enzymes act cooperatively in hemicellulose degradation. Interestingly, some hemicellulases have been reported as virulence factors in fungal pathogens (Brito et al. Citation2006; Nafisi et al. Citation2014; Nguyen et al. Citation2011; Yajima et al. Citation2009). However, different studies failed to demonstrate the role of these enzymes as virulence factors (Apel-Birkhold and Walton Citation1996; Gómez-Gómez et al. Citation2002; Sella et al. Citation2013; Wegener et al. Citation1999; Wu et al. Citation2006). Thus, the role of hemicellulases in pathogenicity, as well as other CWDEs in most plant pathogens, still needs to be demonstrated.
Herein, we found several pectin-degrading enzymes in the N. parvum Bt-67 transcriptome, including polygalacturonases (GH28) and different PLs (PL1, 3, 4, and 9). Remarkably, the highest expressed CAZymes in N. parvum Bt-67 when grown on both biomass growth conditions was a GH28 endo-xylogalacturonan hydrolase (UCRNP2_438). This is in line with Nazar Pour et al. (Citation2022), who found that the most produced enzymes were pectinases when N. parvum grew in presence of Eucalyptus stem, compared with the control condition without plant biomass. Interestingly, GH28 polygalacturonases have been described as virulence factors, being the first enzymes to be secreted when phytopathogens encounter plant cell walls (Kubicek et al. Citation2014). Although the lignocellulosic biomass may present poor content of pectins, these latter have a significant presence in the primary cell wall, contributing to plant resistance against pathogens (Miedes et al. Citation2014).
Production of CAZymes in N. parvum Bt-67 is substrate dependent.
—Although most of the DEG-encoding CAZymes were expressed in both lignocellulosic biomass conditions (94 out of 134), there were differences in CAZyme production, where 21 CAZymes were differentially up-regulated in the GP condition and 19 in WS. Moreover, most of the expression levels of up-regulated CAZymes in both lignocellulosic biomass conditions were higher in the WS condition, especially for cellulases and hemicellulases. These differences in abundance and expression levels of CAZymes produced by N. parvum Bt-67 were correlated with the enzymatic activity assays (). Here, all the evaluated hemicellulases (i.e., xylanase, β-d-xylosidase, and α-l-arabinofuranosidase), as well as β-d-glucosidase, showed higher activity levels in the WS condition. Interestingly, expression levels of xylanases (GH10 and GH11), β-xylosidase (GH3 and GH43), α-l-arabinofuranosidase (GH43 and GH62), and β-glucosidase (GH3) were generally greater when N. parvum Bt-67 grew on WS (SUPPLEMENTARY TABLE 2).
These differences in CAZyme production in our growing conditions, particularly for the (hemi-)cellulolytic enzymes, may be explained by the fact that wheat straw contains a higher amount of cellulose and hemicellulose than grapevine canes (47.4 ± 0.2% vs. 40.4 ± 1.48% and 31.1 ± 1.2% vs. 19.2 ± 1.10%, respectively). Similar explanations have been indicated for the main fungal producer of cellulase and hemicellulase cocktails, Trichoderma reesei, when comparing the CAZyme production during growth on contrasting substrates, including wheat straw (Adav et al. Citation2012; Häkkinen et al. Citation2012). Besides the polysaccharide composition in the different substrates, the structure of hemicellulose may directly influence the fungal CAZyme production. For example, Häkkinen et al. (Citation2012) provided evidence that the induction of CAZyme genes was dependent on the xylan type. These authors also pointed out that the side chains of hemicellulose may play a role in the induction process.
Apart from the cellulose and hemicellulose contents, lignin may influence the CAZyme production since this polymer acts as a barrier in the hydrolysis process. The lignin content in grapevine canes was much higher than in wheat straw (21.4 ± 0.64% vs. 8.5 ± 0.9%). This is in line with previous studies in other wood decay fungi, which reported lower expression and enzymatic activity levels of (hemi-)cellulolytic enzymes when the cultures occurred in presence of substrates with high lignin content (Metreveli et al. Citation2021; Mukherjee and Khowala Citation2016; Rajesh Banu et al. Citation2021; Rytioja et al. Citation2017). Interestingly, on the one hand, we found that when N. parvum Bt-67 grew on the biomass with high lignin content (GP), one AA1 laccase and one AA2 peroxidase, which might be involved in lignin modification, were up-regulated. On the other hand, the fungus in the WS condition only up-regulated one AA2 peroxidase, which was also up-regulated in presence of GP. It should be noted that the expression level of this peroxidase was higher in the GP condition. This is consistent with the higher production of lignin-modifying enzymes of woody-decay fungi in cultures with different wood substrates, compared with growths in non-woody substrates (Fernández-Fueyo et al. Citation2016; Rytioja et al. Citation2017).
Secreted CAZymes by N. parvum Bt-67 degrade the lignocellulosic polymers.
—The degradation of the lignocellulosic components in GP and WS caused by the N. parvum Bt-67 secreted CAZymes was confirmed by the FTIR analysis (). These results are consistent with the transcriptomic analysis and enzymatic activity assays, which demonstrated the cellulolytic and hemicellulolytic enzyme production in both lignocellulosic biomass conditions. In addition, degradation values for lignin were only observed in GP, which correlates with the higher abundance and expression levels of lignin-modifying enzymes (i.e., laccases and peroxidases) when the pathogen grew in presence of this biomass. Nevertheless, degradation values of lignin were not significant (; SUPPLEMENTARY FIG. 2), which accords with the non-detection of laccase/peroxidase activities.
Secondary metabolite production is substrate dependent.
—We detected 564 metabolites produced by the fungus in the three growth conditions (GP, WS, and GLU). We found a higher number of metabolites when the fungus grew with plant biomass (GP or WS) than GLU as the sole carbon source. These findings are not surprising since glucose, which is rapidly metabolized, interferes with the biosynthesis of many secondary metabolites (Demain Citation1986). This interference may be partially due to the carbon catabolite repression (CCR) mechanism in fungi (Brakhage Citation2013). Contrarily to glucose, polysaccharides, oligosaccharides, and oils may favor metabolite production (Demain Citation1986).
PCA and PLS-DA of the metabolites produced by N. parvum Bt-67 allowed the distinction of the growth conditions (). The most discriminant metabolite (FDR < 0.05) was bisnorcholic acid, which belongs to the class of trihydroxy bile acids (Kuramoto et al. Citation1990). Bile acids are steroid compounds with relevant biological functions in mammals, such as solubilization and adsorption of fats, cholesterol, and lipid-soluble vitamins (Kollerov et al. Citation2016). In fungi, bile acids have only been detected in Penicillium sp. (Ohashi et al. Citation2008). In addition, filamentous fungi can transform bile acids (Kollerov et al. Citation2016). Although bile acids might have similar functions in fungi as those described in animals (e.g., solubilization of ergosterol, the main constituent of the fungal plasma membrane), their exact role is unknown. Following bisnorcholic acid, 10′-apo-β-caroten-10′-al was one of the most discriminant compounds. This metabolite is an apo carotenoid produced by the cleavage of β-carotene at the 10′ position (Koschmieder et al. Citation2021). Besides being essential pigments for plants (Koschmieder et al. Citation2021), carotenoids can be produced by fungi (Avalos and Carmen Limón Citation2015). In the latter, the main function of carotenoids is associated with the protection of cells against adverse conditions, such as oxidative stress caused by reactive oxygen species (ROS) and radiation (Avalos and Carmen Limón Citation2015).
Another relevant compound was 2-hydroxy-4,7-dimethoxy-2H-1,4-benzoxazin-3(4H)-one (HDMBOA) (), which is a benzoxazine found in plants from different families, including Poaceae, Acanthaceae, Ranunculaceae, and Scrophulariaceae (Escobar et al. Citation1997). Benzoxazinones have inhibitory effects on plant growth, as well as antimicrobial and insecticide activities (Macías et al. Citation2005). Benzoxazinoids are considered traditional phytoanticipins (Pedras and Yaya Citation2015). Here, HDMBOA was detected in all the replicates of both biomass growth conditions and two out of three replicates in GLU (SUPPLEMENTARY TABLE 3). A possible explanation for this might be that N. parvum Bt-67 has obtained the capacity to produce this plant-derived compound through the so-called horizontal gene transfer (HGT). It has been hypothesized that HGT can take place during interactions between plants and their endophytic counterpart or vice versa (Nicoletti and Fiorentino Citation2015; Salvatore et al. Citation2021). Since N. parvum can have an endophytic lifestyle, HGT might occur. Indeed, the production of plant metabolites by Botryosphaeriaceae has been reported previously. For example, Shi et al. (Citation2012) found that grapevine endophytes, including one Botryosphaeria strain, were able to produce resveratrol, a stilbene phytoalexin produced by plants in response to stress factors. Terpenoid compounds from plants have been extracted from Lasiodiplodia theobromae (Zaher et al. Citation2015) and Botryosphaeria sp. (Yuan et al. Citation2009). Interestingly, we observed that N. parvum Bt-67 was able to differentially produce a plant terpenoid compound recognized as dihydrofukinolide (), a sesquiterpene lactone metabolite identified in Petasites japonicus (Naya et al. Citation1972) and Glycine max (Gupta et al. Citation2018).
The discriminant compounds described above () were highly produced in the WS condition. The presence of WS also influenced the production of ovalicin (), which is a sesquiterpene methyl ether with antibiotic properties (Cane and Levin Citation1976). Structurally, ovalicin is closely related to fumagillin, a well-known antibiotic metabolite produced by Aspergillus fumigatus (Cane and Levin Citation1976). Ovalicin, as well as fumagillin, targets the enzyme methionine aminopeptidase (type 2), which plays an essential role in posttranscriptional modifications of proteins (Griffith et al. Citation1997; Ross et al. Citation2005). Another metabolite positively affected by WS was 5-amino-2,3-dihydro-6-(3-hydroxy-4-methoxy-1-oxobutyl)-2,2-dimethyl-4H-1-benzopyran-4-one, a benzopyran analogous to the mycotoxin fusarochromanone found in Fusarium equiseti (Xie et al. Citation1990). This latter compound inhibits cell proliferation and reduces cell viability by arresting cells at the G0/G1 phase of the cell cycle (Gu et al. Citation2014).
It should be noted that the diversity of differentially produced metabolites was higher in presence of GP (SUPPLEMENTARY FIG. 5; SUPPLEMENTARY TABLE 4). In this growth condition, the most known accumulated metabolites were PC(14:0/22:4(7Z,10Z,13Z,16Z)) and PA(16:1(9Z)/24:1(15Z)). The first compound is a phosphatidylcholine (PC), whereas the second is a phosphatidic acid (PA). Phospholipids, such as PC and PA, are essential for maintaining the structure and functionality of membranes (Lagace and Ridgway Citation2013). They are found in large amounts in fungal membranes (Siebers et al. Citation2016). Phospholipids appear to be relevant for the interaction of symbiotic and pathogenic microorganisms with host cells (Wang et al. Citation2019). For example, PC is pivotal for the functional symbiosis between the bacterium Bradyrhizobium japonicum and soybean (Minder et al. Citation2001), as well as for the obligate biotrophic fungus Rhizophagus irregularis colonizing Lotus japonicus (Wewer et al. Citation2014). Phospholipids have also been described as virulence determinants. Wessel et al. (Citation2006) observed that Agrobacterium tumefaciens mutants unable to synthesize PC exhibited a drastic virulence defect. Wang et al. (Citation2019) showed that phospholipid biosynthetic genes are required for full virulence, mycotoxin production, fungal development, and abiotic stress adaptation of Fusarium graminearum. Hence, it is likely that the higher production of PC and PA by N. parvum Bt-67 in contact with GP corresponds to the fungus’ effort to establish interaction with the host tissue.
The GP growth condition also influenced the production of trigonelline, an alkaloid extracted from various plants, including Fabaceae species and coffee (Ashihara et al. Citation2015). In plants, trigonelline may have roles as a nutrient source, osmoregulator during drought or salt stress, or cell cycle regulator (Ashihara et al. Citation2015). Trigonelline seems to be another example of plant metabolites produced by endophytic fungi. Teimoori-Boghsani et al. (Citation2020) found that isolates of Talaromyces spp. from Salvia abrotanoides produced trigonelline. This metabolite has also been detected in fermentation cultures of Trichoderma asperellum (Ni et al. Citation2019). Another plant metabolite differentially produced in the GP condition was oripavine, a morphinane alkaloid found in Papaver spp. (Hosztafi Citation2014).
In the GLU condition, dipeptides, such as phenylalanylthreonine, alanylisoleucine, isoleucylvaline, and arginylphenylalanine, were among the most differentially produced metabolites. Most of the dipeptides seem to be short-lived intermediates on their way to specific amino acid degradation pathways following further proteolysis (Thirumalaikumar et al. Citation2021). However, some dipeptides may have physiological roles. In the yeast Saccharomyces cerevisiae, dipeptides activate the ubiquitin ligase E3 component N-recognin 1 (UBR1), which mediates the degradation of Cup9, a transcriptional repressor of peptide import (Turner et al. Citation2000). Dipeptides can also have antioxidative activities, protecting cells from oxidative stress (Ozawa et al. Citation2022).
In addition to dipeptides, compounds belonging to the steroid class, such as tetrahydrocorticosterone, 19-norandrosteron, cortexolone, and androsterone, were differentially produced in the GLU condition (; SUPPLEMENTARY TABLE 4). In animals, these compounds are considered steroid hormones, i.e., signaling molecules that mediate vital physiological functions (Dai et al. Citation2012). Interestingly, steroid signal molecules are not exclusive to animals. Steroid hormones, such as testosterone and androstenedione, have been found in fungi, including the plant-pathogenic fungus Cochliobolus lunatus (Kastelic-Suhadolc et al. Citation1994) and the edible mushroom Pleurotus ostreatus (Plemenitaš et al. Citation1999). In addition, the existence of steroid-binding proteins with a high affinity for steroid hormones, together with the steroid biotransformation capacity, has been demonstrated in distinct fungal species (Črešnar and Žakelj-Mavrič Citation2009; Kollerov et al. Citation2020). That implies that fungi possess a steroid signaling system (Žakelj-Mavrič et al. Citation1995); however, the role of steroid hormones in fungi, as well as the other components associated with this signaling system, has not been well understood. Previous studies have found that steroid hormones induce stress responses in fungi. For example, progesterone applications on the yeasts S. cerevisiae and Candida albicans influenced the up-regulation of stress-responsive genes, such as those involved in multidrug resistance, heat shock, osmotic stress, nitrogen depletion, amino acid starvation, and oxidative stress (Banerjee et al. Citation2007, Citation2004). Steroid hormones may also affect fungal growth. Cvelbar et al. (Citation2013) observed that steroid compounds reduced the amount of ergosterol in S. cerevisiae and Hortaea werneckii, but not in Aspergillus oryzae. Considering those previous studies, we could speculate that N. parvum Bt-67 differentially produced steroid hormones to regulate its hyphal growth (e.g., by interfering with the ergosterol biosynthesis) or to enhance its stress responses to starvation during the GLU condition. On the other hand, it might be possible that the differential production of these steroids is associated with other physiological processes in the fungus, such as the regulation of the reproductive phase. Lin et al. (Citation2016) found that pregnenolone produced and secreted by Trichoderma citrinoviride inhibited its conidiation. The Trichoderma pregnenolone also inhibited the conidiation of Fusarium graminearum (Lin et al. Citation2016). Remarkably, the conidiation inhibition was specific for pregnenolone over other steroids (Lin et al. Citation2016). The role of steroid hormones in plant-pathogenic fungi remains to be elucidated.
CONCLUSION
In this study, we found that N. parvum Bt-67 has a differential production of pathogenicity- and virulence-related factors depending on the lignocellulosic biomass. Interestingly, CAZyme expression levels, enzymatic activities, and lignocellulose biomass degradation were higher when the fungus grew with the non-host biomass (WS). These results suggest that wheat straw might be a promising carbon source to produce CAZymes of interest from N. parvum.
In addition, the distinct carbon sources differently enhanced the production of secondary metabolites. Remarkably, the diversity of differentially produced metabolites was higher when N. parvum Bt-67 grew with the host biomass (GP). Some differentially produced metabolites, such as phospholipids and ovalicin, seem to be relevant to the plant-microbe interaction. Others may be involved in the fungal physiology and response to stress conditions, such as diverse steroid compounds and carotenoids. Nevertheless, the exact role of most of the secondary metabolites, either those associated with the plant-pathogen interaction or those with the physiology of the fungus itself, requires further investigation.
Supplemental Material
Download Zip (2.5 MB)DISCLOSURE STATEMENT
No potential conflict of interest was reported by the author(s).
SUPPLEMENTARY MATERIAL
Supplemental data for this article can be accessed online at https://doi.org/10.1080/00275514.2023.2216122
Additional information
Funding
LITERATURE CITED
- Abou-Mansour E, Débieux J-L, Ramírez-Suero M, Bénard-Gellon M, Magnin-Robert M, Spagnolo A, Chong J, Farine S, Bertsch C, L’Haridon F, et al. 2015. Phytotoxic metabolites from Neofusicoccum parvum, a pathogen of Botryosphaeria dieback of grapevine. Phytochemistry. 115:207–15. doi:10.1016/j.phytochem.2015.01.012.
- Adapa PK, Karunakaran C, Tabil LG, Schoenau GJ. 2009. Potential applications of infrared and Raman spectromicroscopy for agricultural biomass. Agric Eng Int: CIGR J. 11:1081.
- Adav SS, Chao LT, Sze SK. 2012. Quantitative secretomic analysis of Trichoderma reesei strains reveals enzymatic composition for lignocellulosic biomass degradation. Mol Cell Proteomics. 11(7):M111.012419. doi:10.1074/mcp.M111.012419.
- Álvarez Á, Yáñez J, Neira Y, Castillo-Felices R, Hinrichsen P. 2020. Simple distinction of grapevine (Vitis vinifera L.) genotypes by direct ATR-FTIR. Food Chem. 328:127164. doi:10.1016/j.foodchem.2020.127164.
- Andolfi A, Mugnai L, Luque J, Surico G, Cimmino A, Evidente A. 2011. Phytotoxins produced by fungi associated with grapevine trunk diseases. Toxins. 3(12):1569–605. doi:10.3390/toxins3121569.
- Andrews S. 2010. A quality control tool for high throughput sequence data. Ver. 0.11.9. [FastQC]. Cambridge (UK): Babraham Institute.
- Apel-Birkhold PC, Walton JD. 1996. Cloning, disruption, and expression of two endo-beta 1, 4-xylanase genes, XYL2 and XYL3, from Cochliobolus carbonum. Appl Environ Microbiol. 62(11):4129–35. doi:10.1128/aem.62.11.4129-4135.1996.
- Ashburner M, Ball CA, Blake JA, Botstein D, Butler H, Cherry JM, Davis AP, Dolinski K, Dwight SS, Eppig JT, et al. 2000. Gene Ontology: tool for the unification of biology. Nat Genet. 25(1):25–29. doi:10.1038/75556.
- Ashihara H, Ludwig IA, Katahira R, Yokota T, Fujimura T, Crozier A. 2015. Trigonelline and related nicotinic acid metabolites: occurrence, biosynthesis, taxonomic considerations, and their roles in planta and in human health. Phytochem Rev. 14(5):765–98. doi:10.1007/s11101-014-9375-z.
- Avalos J, Carmen Limón M. 2015. Biological roles of fungal carotenoids. Curr Genet. 61(3):309–24. doi:10.1007/s00294-014-0454-x.
- Bach CE, Warnock DD, Van Horn DJ, Weintraub MN, Sinsabaugh RL, Allison SD, German DP. 2013. Measuring phenol oxidase and peroxidase activities with pyrogallol, l-DOPA, and ABTS: effect of assay conditions and soil type. Soil Biol Biochem. 67:183–91. doi:10.1016/j.soilbio.2013.08.022.
- Banerjee D, Martin N, Nandi S, Shukla S, Dominguez A, Mukhopadhyay G, Prasad R. 2007. A genome-wide steroid response study of the major human fungal pathogen Candida albicans. Mycopathologia. 164(1):1–17. doi:10.1007/s11046-007-9025-8.
- Banerjee D, Pillai B, Karnani N, Mukhopadhyay G, Prasad R. 2004. Genome-wide expression profile of steroid response in Saccharomyces cerevisiae. Biochem Biophys Res Commun. 317(2):406–13. doi:10.1016/j.bbrc.2004.03.053.
- Belair M, Restrepo-Leal JD, Praz C, Fontaine F, Rémond C, Fernandez O, Besaury L. 2023. Botryosphaeriaceae gene machinery: correlation between diversity and virulence. Fungal Biol. 127(5):1010–31. doi:10.1016/j.funbio.2023.03.004.
- Bertsch C, Ramírez-Suero M, Magnin-Robert M, Larignon P, Chong J, Abou-Mansour E, Spagnolo A, Clément C, Fontaine F. 2013. Grapevine trunk diseases: complex and still poorly understood. Plant Pathol. 62:243–65.
- Blanco-Ulate B, Rolshausen P, Cantu D. 2013. Draft genome sequence of Neofusicoccum parvum isolate UCR-NP2, a fungal vascular pathogen associated with grapevine cankers. Genome Announc. 1(3):e00339–13. doi:10.1128/genomeA.00339-13.
- Boraston AB, Bolam DN, Gilbert HJ, Davies GJ. 2004. Carbohydrate-binding modules: fine-tuning polysaccharide recognition. Biochem J. 382:769–81.
- Brakhage AA. 2013. Regulation of fungal secondary metabolism. Nat Rev Microbiol. 11:21–32.
- Brito N, Espino JJ, González C. 2006. The endo-β-1,4-Xylanase Xyn11A is required for virulence in Botrytis cinerea. Mol Plant Microbe Interact. 19(1):25–32. doi:10.1094/MPMI-19-0025.
- Buckel I, Andernach L, Schüffler A, Piepenbring M, Opatz T, Thines E. 2017. Phytotoxic dioxolanones are potential virulence factors in the infection process of Guignardia bidwellii. Sci Rep. 7(1):8926. doi:10.1038/s41598-017-09157-6.
- Cane DE, Levin RH. 1976. Application of carbon-13 magnetic resonance to isoprenoid biosynthesis. II. Ovalicin and the use of doubly labeled mevalonate. J Am Chem Soc. 98(5):1183–88. doi:10.1021/ja00421a022.
- Cassarini M, Besaury L, Rémond C. 2021. Valorisation of wheat bran to produce natural pigments using selected microorganisms. J Biotechnol. 339:81–92. doi:10.1016/j.jbiotec.2021.08.003.
- Cassarini M, Crônier D, Besaury L, Rémond C. 2022. Protein-rich agro-industrial co-products are key substrates for growth of Chromobacterium vaccinii and its violacein bioproduction. Waste Biomass Valori. 13(11):4459–68. doi:10.1007/s12649-022-01798-7.
- Chang S, Puryear J, Cairney J. 1993. A simple and efficient method for isolating RNA from pine trees. Plant Mol Biol Rep. 11(2):113–16. doi:10.1007/BF02670468.
- Claverie M, Notaro M, Fontaine F, Wery J. 2020. Current knowledge on grapevine trunk diseases with complex etiology: a systemic approach. Phytopathol Mediterr. 59(1):29–53. doi:10.36253/phyto-11150.
- Cragg SM, Beckham GT, Bruce NC, Bugg TD, Distel DL, Dupree P, Etxabe AG, Goodell BS, Jellison J, McGeehan JE, et al. 2015. Lignocellulose degradation mechanisms across the tree of life. Curr Opin Chem Biol. 29:108–19. doi:10.1016/j.cbpa.2015.10.018.
- Črešnar B, Žakelj-Mavrič M. 2009. Aspects of the steroid response in fungi. Chem Biol Interact. 178(1–3):303–09. doi:10.1016/j.cbi.2008.11.002.
- Cvelbar D, Žist V, Kobal K, Žigon D, Žakelj-Mavrič M. 2013. Steroid toxicity and detoxification in ascomycetous fungi. Chem Biol Interact. 202(1–3):243–58. doi:10.1016/j.cbi.2012.11.025.
- Dai W, Huang Q, Yin P, Li J, Zhou J, Kong H, Zhao C, Lu X, Xu G. 2012. Comprehensive and highly sensitive urinary steroid hormone profiling method based on stable isotope-labeling liquid chromatography–mass spectrometry. Anal Chem. 84(23):10245–51. doi:10.1021/ac301984t.
- Demain AL. 1986. Regulation of secondary metabolism in fungi. Pure Appl Chem. 58(2):219–26. doi:10.1351/pac198658020219.
- Eastwood DC, Floudas D, Binder M, Majcherczyk A, Schneider P, Aerts A, Asiegbu FO, Baker SE, Barry K, Bendiksby M, et al. 2011. The plant cell wall—decomposing machinery underlies the functional diversity of forest fungi. Science. 333(6043):762–65. doi:10.1126/science.1205411.
- Eriksson K-E PB. 1975. Extracellular enzyme system utilized by the fungus Sporotrichum pulverulentum (Chrysosporium lignorum) for the breakdown of cellulose. Eur J Biochem. 51(1):193–206. doi:10.1111/j.1432-1033.1975.tb03919.x.
- Escobar CA, Kluge M, Sicker D. 1997. Syntheses of 2-hydroxy-4,7-dimethoxy-2H-1,4-benzoxazin-3(4H)-one: a precursor of a bioactive electrophile from Gramineae. Tetrahedron Lett. 38(6):1017–20. doi:10.1016/S0040-4039(96)02492-6.
- Esteves AC, Saraiva M, Correia A, Alves A. 2014. Botryosphaeriales fungi produce extracellular enzymes with biotechnological potential. Can J Microbiol. 60(5):332–42. doi:10.1139/cjm-2014-0134.
- Evidente A, Punzo B, Andolfi A, Cimmino A, Melck D, Luque J. 2010. Lipophilic phytotoxins produced by Neofusicoccum parvum, a grapevine canker agent. Phytopathol Mediterr. 49:74–79.
- Fernández-Fueyo E, Ruiz-Dueñas FJ, López-Lucendo MF, Pérez-Boada M, Rencoret J, Gutiérrez A, Pisabarro AG, Ramírez L, Martínez AT. 2016. A secretomic view of woody and nonwoody lignocellulose degradation by Pleurotus ostreatus. Biotechnol Biofuels. 9(1):49. doi:10.1186/s13068-016-0462-9.
- Fontaine F, Pinto C, Vallet J, Clément C, Gomes AC, Spagnolo A. 2016. The effects of grapevine trunk diseases (GTDs) on vine physiology. Eur J Plant Pathol. 144(4):707–21. doi:10.1007/s10658-015-0770-0.
- Frazee AC, Pertea G, Jaffe AE, Langmead B, Salzberg SL, Leek JT. 2015. Ballgown bridges the gap between transcriptome assembly and expression analysis. Nat Biotechnol. 33(3):243–46. doi:10.1038/nbt.3172.
- Garcia JF, Lawrence DP, Morales-Cruz A, Travadon R, Minio A, Hernandez-Martinez R, Rolshausen PE, Baumgartner K, Cantu D. 2021. Phylogenomics of plant-associated Botryosphaeriaceae species. Front Microbiol. 12: 652802.
- Gaskell J, Blanchette RA, Stewart PE, BonDurant SS, Adams M, Sabat G, Kersten P, Cullen D. 2016. Transcriptome and secretome analyses of the wood decay fungus Wolfiporia cocos support alternative mechanisms of lignocellulose conversion. Appl Environ Microbiol. 82(13):3979–87. doi:10.1128/AEM.00639-16.
- Gibson DM, King BC, Hayes ML, Bergstrom GC. 2011. Plant pathogens as a source of diverse enzymes for lignocellulose digestion. Curr Opin Microbiol. 14(3):264–70. doi:10.1016/j.mib.2011.04.002.
- Gómez-Gómez E, Ruı́z-Roldán MC, Di Pietro A, Roncero MIG, Hera C. 2002. Role in pathogenesis of two endo-β-1,4-xylanase genes from the vascular wilt fungus Fusarium oxysporum. Fungal Genet Biol. 35(3):213–22. doi:10.1006/fgbi.2001.1318.
- Griffith EC, Su Z, Turk BE, Chen S, Chang Y-H, Wu Z, Biemann K, Liu JO. 1997. Methionine aminopeptidase (type 2) is the common target for angiogenesis inhibitors AGM-1470 and ovalicin. Chem Biol. 4(6):461–71. doi:10.1016/S1074-5521(97)90198-8.
- Gu Y, Chen X, Shang C, Singh K, Barzegar M, Mahdavian E, Salvatore BA, Jiang S, Huang S. 2014. Fusarochromanone induces G1 cell cycle arrest and apoptosis in COS7 and HEK293 cells. PLOS ONE. 9(11):e112641. doi:10.1371/journal.pone.0112641.
- Gupta R, Min CW, Kramer K, Agrawal GK, Rakwal R, Park K-H, Wang Y, Finkemeier I, Kim ST. 2018. A Multi-omics analysis of Glycine max leaves reveals alteration in flavonoid and isoflavonoid metabolism upon ethylene and abscisic acid treatment. Proteomics. 18(7):1700366. doi:10.1002/pmic.201700366.
- Häkkinen M, Arvas M, Oja M, Aro N, Penttilä M, Saloheimo M, Pakula TM. 2012. Re-annotation of the CAZy genes of Trichoderma reesei and transcription in the presence of lignocellulosic substrates. Microb Cell Fact. 11(1):134. doi:10.1186/1475-2859-11-134.
- Hosztafi S. 2014. Recent advances in the chemistry of oripavine and its derivatives. Adv Biosci Biotechnol. 05(8):704–17. doi:10.4236/abb.2014.58084.
- Kastelic-Suhadolc T, Plemenitaš A, Žigon D. 1994. Isolation and identification of testosterone and androstenedione in the fungus Cochliobolus lunatus. Steroids. 59(6):357–61. doi:10.1016/0039-128X(94)90002-7.
- Kidby DK, Davidson DJ. 1973. A convenient ferricyanide estimation of reducing sugars in the nanomole range. Anal Biochem. 55(1):321–25. doi:10.1016/0003-2697(73)90323-0.
- Kim D, Langmead B, Salzberg SL. 2015. HISAT: a fast spliced aligner with low memory requirements. Nat Methods. 12(4):357–60. doi:10.1038/nmeth.3317.
- Kollerov VV, Lobastova TG, Monti D, Deshcherevskaya NO, Ferrandi EE, Fronza G, Riva S, Donova MV. 2016. Deoxycholic acid transformations catalyzed by selected filamentous fungi. Steroids. 107:20–29. doi:10.1016/j.steroids.2015.12.015.
- Kollerov V, Shutov A, Kazantsev A, Donova M. 2020. Biotransformation of androstenedione and androstadienedione by selected Ascomycota and Zygomycota fungal strains. Phytochemistry. 169:112160. doi:10.1016/j.phytochem.2019.112160.
- Koschmieder J, Wüst F, Schaub P, Álvarez D, Trautmann D, Krischke M, Rustenholz C, Mano J, Mueller MJ, Bartels D, et al. 2021. Plant apocarotenoid metabolism utilizes defense mechanisms against reactive carbonyl species and xenobiotics. Plant Physiol. 185(2):331–51. doi:10.1093/plphys/kiaa033.
- Kubicek CP, Kubicek EM. 2016. Enzymatic deconstruction of plant biomass by fungal enzymes. Curr Opin Chem Biol. 35:51–57. doi:10.1016/j.cbpa.2016.08.028.
- Kubicek CP, Starr TL, Glass NL. 2014. Plant cell wall–degrading enzymes and their secretion in plant-pathogenic Fungi. Annu Rev Phytopathol. 52(1):427–51. doi:10.1146/annurev-phyto-102313-045831.
- Kuramoto T, Furukawa Y, Nishina T, Sugimoto T, Mahara R, Tohma M, Kihira K, Hoshita T. 1990. Identification of short side chain bile acids in urine of patients with cerebrotendinous xanthomatosis. J Lipid Res. 31(10):1895–902. doi:10.1016/S0022-2275(20)42333-8.
- Lagace TA, Ridgway ND. 2013. The role of phospholipids in the biological activity and structure of the endoplasmic reticulum. Biochimica Et Biophysica Acta (BBA) - Molecular Cell Research. 1833(11):2499–510. doi:10.1016/j.bbamcr.2013.05.018.
- Larignon P, Fulchic R, Cere L, Dubos B. 2001. Observation on black dead arm in French vineyards. Phytopathol Mediterr. 40:S336–S342.
- Leonowicz A, Grzywnowicz K. 1981. Quantitative estimation of laccase forms in some white-rot fungi using syringaldazine as a substrate. Enzyme Microb Technol. 3(1):55–58. doi:10.1016/0141-0229(81)90036-3.
- Levasseur O, Stafford L, Gherardi N, Naudé N, Beche E, Esvan J, Blanchet P, Riedl B, Sarkissian A. 2013. Role of substrate outgassing on the formation dynamics of either hydrophilic or hydrophobic wood surfaces in atmospheric-pressure, organosilicon plasmas. Surf Coat Technol. 234:42–47. doi:10.1016/j.surfcoat.2013.05.045.
- Lin H, Travisano M, Kazlauskas RJ. 2016. The fungus Trichoderma regulates submerged conidiation using the steroid pregnenolone. ACS Chem Biol. 11(9):2568–75. doi:10.1021/acschembio.6b00376.
- Lombard V, Golaconda Ramulu H, Drula E, Coutinho PM, Henrissat B. 2014. The carbohydrate-active enzymes database (CAZy) in 2013. Nucleic Acids Res. 42(D1):D490–D495. doi:10.1093/nar/gkt1178.
- MacDonald J, Doering M, Canam T, Gong Y, Guttman DS, Campbell MM, Master ER. 2011. Transcriptomic responses of the softwood-degrading white-rot fungus Phanerochaete carnosa during growth on coniferous and deciduous wood. Appl Environ Microbiol. 77(10):3211–18. doi:10.1128/AEM.02490-10.
- Macías FA, Marín D, Oliveros-Bastidas A, Castellano D, Simonet AM, Molinillo JMG. 2005. Structure−Activity Relationships (SAR) studies of benzoxazinones, their degradation products and analogues. Phytotoxicity on Standard Target Species (STS). J Agric Food Chem. 53(3):538–48. doi:10.1021/jf0484071.
- Martos S, Andolfi A, Luque J, Mugnai L, Surico G, Evidente A. 2008. Production of phytotoxic metabolites by five species of Botryosphaeriaceae causing decline on grapevines, with special interest in the species Neofusicoccum luteum and N. parvum. Eur J Plant Pathol. 121(4):451–61. doi:10.1007/s10658-007-9263-0.
- Massonnet M, Morales-Cruz A, Figueroa-Balderas R, Lawrence DP, Baumgartner K, Cantu D. 2018. Condition-dependent co-regulation of genomic clusters of virulence factors in the grapevine trunk pathogen Neofusicoccum parvum. Mol Plant Pathol. 19(1):21–34. doi:10.1111/mpp.12491.
- Metreveli E, Khardziani T, Elisashvili V. 2021. The carbon source controls the secretion and yield of polysaccharide-hydrolyzing enzymes of Basidiomycetes. Biomolecules. 11(9):1341. doi:10.3390/biom11091341.
- Miedes E, Vanholme R, Boerjan W, Molina A. 2014. The role of the secondary cell wall in plant resistance to pathogens. Front Plant Sci. 5:5: 358. doi:10.3389/fpls.2014.00005.
- Minder AC, Kee DR, Narberhaus F, Fischer H-M, Hennecke H, Geiger O. 2001. Phosphatidylcholine levels in Bradyrhizobium japonicum membranes are critical for an efficient symbiosis with the soybean host plant. Mol Microbiol. 39(5):1186–98. doi:10.1111/j.1365-2958.2001.02325.x.
- Mondello V, Songy A, Battiston E, Pinto C, Coppin C, Trotel-Aziz P, Clément C, Mugnai L, Fontaine F. 2018. Grapevine trunk diseases: a review of fifteen years of trials for their control with chemicals and biocontrol agents. Plant Dis. 102(7):1189–217. doi:10.1094/PDIS-08-17-1181-FE.
- Morales-Cruz A, Amrine KCH, Blanco-Ulate B, Lawrence DP, Travadon R, Rolshausen PE, Baumgartner K, Cantu D. 2015. Distinctive expansion of gene families associated with plant cell wall degradation, secondary metabolism, and nutrient uptake in the genomes of grapevine trunk pathogens. BMC Genom. 16(1):469. doi:10.1186/s12864-015-1624-z.
- Mukherjee S, Khowala S. 2016. Unraveling the secretome of Termitomyces clypeatus grown on agroresidues as a potential source for bioethanol production. Process Biochem. 51(11):1793–807. doi:10.1016/j.procbio.2015.11.019.
- Nafisi M, Stranne M, Zhang L, van Kan JAL, Sakuragi Y. 2014. The endo-arabinanase bcara1 is a novel host-specific virulence factor of the necrotic fungal phytopathogen Botrytis cinerea. Mol Plant Microbe Interact. 27(8):781–92. doi:10.1094/MPMI-02-14-0036-R.
- Nagel JH, Wingfield MJ, Slippers B. 2021. Increased abundance of secreted hydrolytic enzymes and secondary metabolite gene clusters define the genomes of latent plant pathogens in the Botryosphaeriaceae. BMC Genom. 22(1):589. doi:10.1186/s12864-021-07902-w.
- Naya K, Hayashi M, Takagi I, Nakamura S, Kobayashi M. 1972. The structural elucidation of sesquiterpene lactones from Petasites japonicus Maxim. Bull Chem Soc Jpn. 45(12):3673–85. doi:10.1246/bcsj.45.3673.
- Nazar Pour F, Cobos R, Rubio Coque JJ, Serôdio J, Alves A, Félix C, Ferreira V, Esteves AC, Duarte AS. 2020. Toxicity of recombinant Necrosis and Ethylene-Inducing Proteins (NLPs) from Neofusicoccum parvum. Toxins. 12(4):235. doi:10.3390/toxins12040235.
- Nazar Pour F, Pedrosa B, Oliveira M, Fidalgo C, Devreese B, Driessche GV, Félix C, Rosa N, Alves A, Duarte AS, et al. 2022. Unveiling the secretome of the fungal plant pathogen Neofusicoccum parvum induced by in vitro host mimicry. J Fungi. 8(9):971. doi:10.3390/jof8090971.
- Nguyen QB, Itoh K, Van Vu B, Tosa Y, Nakayashiki H. 2011. Simultaneous silencing of endo-β-1,4 xylanase genes reveals their roles in the virulence of Magnaporthe oryzae. Mol Microbiol. 81(4):1008–19. doi:10.1111/j.1365-2958.2011.07746.x.
- Nicoletti R, Fiorentino A. 2015. Plant bioactive metabolites and drugs produced by endophytic fungi of Spermatophyta. Agriculture. 5(4):918–70. doi:10.3390/agriculture5040918.
- Ni M, Wu Q, Wang GS, Liu QQ, Yu MX, Tang J. 2019. Analysis of metabolic changes in Trichoderma asperellum TJ01 at different fermentation time-points by LC-QQQ-MS. J Environ Sci Health B. 54(1):20–26. doi:10.1080/03601234.2018.1507227.
- Ohashi K, Miyagawa Y, Nakamura Y, Shibuya H. 2008. Bioproduction of bile acids and the glycine conjugates by Penicillium fungus. J Nat Med. 62(1):83–86. doi:10.1007/s11418-007-0190-3.
- Oliveros JC. 2007. VENNY. An interactive tool for comparing lists with Venn diagrams. https://bioinfogp.cnb.csic.es/tools/venny/index.html.
- Ozawa H, Miyazawa T, Burdeos GC, Miyazawa T. 2022. Biological functions of antioxidant dipeptides. J Nutr Sci Vitaminol (Tokyo). 68(3):162–71. doi:10.3177/jnsv.68.162.
- Pang Z, Chong J, Zhou G, de Lima Morais DA, Chang L, Barrette M, Gauthier C, P-é J, Li S, Xia J. 2021. MetaboAnalyst 5.0: narrowing the gap between raw spectra and functional insights. Nucleic Acids Res. 49(W1):W388–W396. doi:10.1093/nar/gkab382.
- Pedras MSC, Yaya EE. 2015. Plant chemical defenses: are all constitutive antimicrobial metabolites phytoanticipins? Nat Prod Commun. 10(1):209–18. doi:10.1177/1934578X1501000142.
- Pertea M, Kim D, Pertea GM, Leek JT, Salzberg SL. 2016. Transcript-level expression analysis of RNA-seq experiments with HISAT, StringTie and Ballgown. Nat Protoc. 11(9):1650–67. doi:10.1038/nprot.2016.095.
- Phillips AJL, Alves A, Abdollahzadeh J, Slippers B, Wingfield MJ, Groenewald JZ, Crous PW. 2013. The Botryosphaeriaceae: genera and species known from culture. Stud Mycol. 76:51–167. doi:10.3114/sim0021.
- Plemenitaš A, Kastelic-Suhadolc T, Žigon D, Žakelj-Mavrič M. 1999. Steroidogenesis in the fungus Pleurotus ostreatus. Comp Biochem Physiol B Biochem Mol Biol. 123(2):175–79. doi:10.1016/S0305-0491(99)00053-X.
- Rahman MS, Fernando S, Ross B, Wu J, Qin W. 2018. Endoglucanase (EG) activity assays, and Lübeck M, editor. Cellulases: methods and protocols. New York (NY, USA): Humana Press. p. 169–83.
- Rajesh Banu J, Preethi KS, Tyagi VK, Gunasekaran M, Karthikeyan OP, Kumar G. 2021. Lignocellulosic biomass based biorefinery: a successful platform towards circular bioeconomy. Fuel. 302:121086. doi:10.1016/j.fuel.2021.121086.
- Ramírez-Suero M, Bénard-Gellon M, Chong J, Laloue H, Stempien E, Abou-Mansour E, Fontaine F, Larignon P, Mazet-Kieffer F, Farine S, et al. 2014. Extracellular compounds produced by fungi associated with Botryosphaeria dieback induce differential defence gene expression patterns and necrosis in Vitis vinifera cv. Chardonnay cells. Chardonnay cells. Protoplasma. 251(6):1417–26. doi:10.1007/s00709-014-0643-y.
- Reis P, Gaspar A, Alves A, Fontaine F, Lourenço I, Saramago J, Mota M, Rego C. 2020. Early season symptoms on stem, inflorescences and flowers of grapevine associated with Botryosphaeriaceae species. Plants. 9(11):1427. doi:10.3390/plants9111427.
- Reis P, Magnin-Robert M, Nascimento T, Spagnolo A, Abou-Mansour E, Fioretti C, Clément C, Rego C, Fontaine F. 2016. Reproducing Botryosphaeria dieback foliar symptoms in a simple model system. Plant Dis. 100(6):1071–79. doi:10.1094/PDIS-10-15-1194-RE.
- Reveglia P, Savocchia S, Billones-Baaijens R, Masi M, Cimmino A, Evidente A. 2019. Phytotoxic metabolites by nine species of Botryosphaeriaceae involved in grapevine dieback in Australia and identification of those produced by Diplodia mutila, Diplodia seriata, Neofusicoccum australe and Neofusicoccum luteum. Nat Prod Res. 33(15):2223–29. doi:10.1080/14786419.2018.1497631.
- Ross S, Giglione C, Pierre M, Espagne C, Meinnel T. 2005. Functional and developmental impact of cytosolic protein n-terminal methionine excision in Arabidopsis. Plant Physiol. 137(2):623–37. doi:10.1104/pp.104.056861.
- Rytioja J, Hildén K, Di Falco M, Zhou M, Aguilar-Pontes MV, O-M S, Tsang A, de Vries RP, MR M. 2017. The molecular response of the white-rot fungus Dichomitus squalens to wood and non-woody biomass as examined by transcriptome and exoproteome analyses. Environ Microbiol. 19(3):1237–50. doi:10.1111/1462-2920.13652.
- Sakalidis ML, Slippers B, Wingfield BD, GEStJ H, Burgess TI. 2013. The challenge of understanding the origin, pathways and extent of fungal invasions: global populations of the Neofusicoccum parvum-N. ribis species complex. Divers Distrib. 19(8):873–83. doi:10.1111/ddi.12030.
- Saldarriaga-Hernández S, Velasco-Ayala C, Leal-Isla Flores P. de Jesús Rostro-Alanis M, Parra-Saldivar R, Iqbal HMN, Carrillo-Nieves D. 2020. Biotransformation of lignocellulosic biomass into industrially relevant products with the aid of fungi-derived lignocellulolytic enzymes. Int J Biol Macromol. 161:1099–116. doi:10.1016/j.ijbiomac.2020.06.047.
- Salvatore MM, Alves A, Andolfi A. 2021. Secondary metabolites produced by Neofusicoccum species associated with plants: a review. Agriculture. 11(2):149. doi:10.3390/agriculture11020149.
- Sella L, Gazzetti K, Faoro F, Odorizzi S, D’Ovidio R, Schäfer W, Favaron F. 2013. A Fusarium graminearum xylanase expressed during wheat infection is a necrotizing factor but is not essential for virulence. Plant Physiol Biochem. 64:1–10. doi:10.1016/j.plaphy.2012.12.008.
- Shi J, Zeng Q, Liu Y, Pan Z. 2012. Alternaria sp. MG1, a resveratrol-producing fungus: isolation, identification, and optimal cultivation conditions for resveratrol production. Appl Microbiol Biotechnol. 95(2):369–79. doi:10.1007/s00253-012-4045-9.
- Siebers M, Brands M, Wewer V, Duan Y, Hölzl G, Dörmann P. 2016. Lipids in plant–microbe interactions. Biochimica Et Biophysica Acta (BBA) - Molecular and Cell Biology of Lipids. 1861(9):1379–95. doi:10.1016/j.bbalip.2016.02.021.
- Stempien E, Goddard M-L, Leva Y, Bénard-Gellon M, Laloue H, Farine S, Kieffer-Mazet F, Tarnus C, Bertsch C, Chong J. 2018. Secreted proteins produced by fungi associated with Botryosphaeria dieback trigger distinct defense responses in Vitis vinifera and Vitis rupestris cells. Protoplasma. 255(2):613–28. doi:10.1007/s00709-017-1175-z.
- Stempien E, Goddard M-L, Wilhelm K, Tarnus C, Bertsch C, Chong J. 2017. Grapevine Botryosphaeria dieback fungi have specific aggressiveness factor repertory involved in wood decay and stilbene metabolization. PLOS ONE. 12(12):e0188766. doi:10.1371/journal.pone.0188766.
- Teimoori-Boghsani Y, Ganjeali A, Cernava T, Müller H, Asili J, Berg G. 2020. Endophytic fungi of native Salvia abrotanoides plants reveal high taxonomic diversity and unique profiles of secondary metabolites. Front Microbiol. 11:10: 3013. doi:10.3389/fmicb.2020.00010.
- Thirumalaikumar VP, Wagner M, Balazadeh S, Skirycz A. 2021. Autophagy is responsible for the accumulation of proteogenic dipeptides in response to heat stress in Arabidopsis thaliana. FEBS J. 288(1):281–92. doi:10.1111/febs.15336.
- Travadon R, Rolshausen PE, Gubler WD, Cadle-Davidson L, Baumgartner K. 2013. Susceptibility of cultivated and Wild Vitis spp. to wood infection by fungal trunk pathogens. Plant Dis. 97(12):1529–36. doi:10.1094/PDIS-05-13-0525-RE.
- Trotel-Aziz P, Robert-Siegwald G, Fernandez O, Leal C, Villaume S, Guise J-F, Abou-Mansour E, Lebrun M-H,Fontaine F. 2022. Diversity of Neofusicoccum parvum for the production of the phytotoxic metabolites (-)-terremutin and (R)-mellein. J Fungi. 8(3):319. doi:10.3390/jof8030319.
- Turner GC, Du F, Varshavsky A. 2000. Peptides accelerate their uptake by activating a ubiquitin-dependent proteolytic pathway. Nature. 405(6786):579–83. doi:10.1038/35014629.
- Umezawa K, Niikura M, Kojima Y, Goodell B, Yoshida M. 2020. Transcriptome analysis of the brown rot fungus Gloeophyllum trabeum during lignocellulose degradation. PLOS ONE. 15(12):e0243984. doi:10.1371/journal.pone.0243984.
- Úrbez-Torres JR. 2011. The status of Botryosphaeriaceae species infecting grapevines. Phytopathol Mediterr. 50:S5–S45.
- Úrbez-Torres JR, Gubler WD. 2009. Pathogenicity of Botryosphaeriaceae species isolated from grapevine cankers in California. Plant Dis. 93(6):584–92. doi:10.1094/PDIS-93-6-0584.
- Van den Wymelenberg A, Gaskell J, Mozuch M, Splinter Bondurant S, Sabat G, Ralph J, Skyba O, Mansfield SD, Blanchette RA, Grigoriev IV, et al. 2011. Significant alteration of gene expression in wood decay fungi Postia placenta and Phanerochaete chrysosporium by plant species. Appl Environ Microbiol. 77(13):4499–507. doi:10.1128/AEM.00508-11.
- Van Soest PJ, Robertson JB. 1980. Systems of analysis for evaluating fibrous feeds. In: Pigden WJ, Balch CC, Graham M, editors. Standardization of analytical methodology for feeds: proceedings of a workshop held in Ottawa, Canada, 1979 Mar 12–14. Ottawa (Canada): International Development Research Centre. p. 49–60.
- Viel M, Collet F, Lanos C. 2018. Chemical and multi-physical characterization of agro-resources’ by-product as a possible raw building material. Ind Crops Prod. 120:214–37. doi:10.1016/j.indcrop.2018.04.025.
- Wang J, Wang H, Zhang C, Wu T, Ma Z, Chen Y. 2019. Phospholipid homeostasis plays an important role in fungal development, fungicide resistance and virulence in Fusarium graminearum. Phytopathology Res. 1(1):16. doi:10.1186/s42483-019-0023-9.
- Wegener S, Ransom RF, Walton J. 1999. A unique eukaryotic β-xylosidase gene from the phytopathogenic fungus Cochliobolus carbonum. Microbiology. 145(5):1089–95. doi:10.1099/13500872-145-5-1089.
- Wessel M, Klüsener S, Gödeke J, Fritz C, Hacker S, Narberhaus F. 2006. Virulence of Agrobacterium tumefaciens requires phosphatidylcholine in the bacterial membrane. Mol Microbiol. 62(3):906–15. doi:10.1111/j.1365-2958.2006.05425.x.
- Wewer V, Brands M, Dörmann P. 2014. Fatty acid synthesis and lipid metabolism in the obligate biotrophic fungus Rhizophagus irregularis during mycorrhization of Lotus japonicus. Plant J. 79(3):398–412. doi:10.1111/tpj.12566.
- Wu B, Gaskell J, Held BW, Toapanta C, Vuong TV, Ahrendt S, Lipzen A, Zhang J, Schilling JS, Master E, et al. 2021. Retracted and Republished from: “Substrate-Specific Differential Gene Expression and RNA Editing in the Brown Rot Fungus Fomitopsis pinicola”. Appl Environ Microbiol. 87(16):e00329–21. doi:10.1128/AEM.00329-21.
- Wu S-C, Halley JE, Luttig C, Fernekes LM, Gutiérrez-Sanchez G, Darvill AG, Albersheim P. 2006. Identification of an endo -β-1,4- d -Xylanase from Magnaporthe grisea by gene knockout analysis, purification, and heterologous expression. Appl Environ Microbiol. 72(2):986–93. doi:10.1128/AEM.72.2.986-993.2006.
- Xie W, Mirocha CJ, Wen Y, Pawlosky RJ. 1990. Isolation and structural identification of a new metabolite of Fusarium equiseti. Appl Environ Microbiol. 56(9):2946–48. doi:10.1128/aem.56.9.2946-2948.1990.
- Xu F, Yu J, Tesso T, Dowell F, Wang D. 2013. Qualitative and quantitative analysis of lignocellulosic biomass using infrared techniques: a mini-review. Appl Energy. 104:801–09. doi:10.1016/j.apenergy.2012.12.019.
- Yajima W, Liang Y, Kav NNV. 2009. Gene disruption of an arabinofuranosidase/β-xylosidase precursor decreases Sclerotinia sclerotiorum virulence on canola tissue. Mol Plant Microbe Interact. 22(7):783–89. doi:10.1094/MPMI-22-7-0783.
- Yuan L, Zhao P-J, Ma J, Lu C-H, Shen Y-M. 2009. Labdane and Tetranorlabdane Diterpenoids from Botryosphaeria sp. MHF, an Endophytic fungus of Maytenus hookeri. Helv Chim Acta. 92(6):1118–25. doi:10.1002/hlca.200800424.
- Yu C, Diao Y, Lu Q, Zhao J, Cui S, Xiong X, Lu A, Zhang X, Liu H. 2022. Comparative genomics reveals evolutionary traits, mating strategies, and pathogenicity-related genes variation of Botryosphaeriaceae. Front Microbiol. 13: 800981.
- Zaher AM, Moharram AM, Davis R, Panizzi P, Makboul MA, Calderón AI. 2015. Characterisation of the metabolites of an antibacterial endophyte Botryodiplodia theobromae Pat. of Dracaena draco L. by LC–MS/MS. Nat Prod Res. 29(24):2275–81. doi:10.1080/14786419.2015.1012715.
- Žakelj-Mavrič M, Kastelic-Suhadolc T, Plemenitaš A, Rižner TL, Belič I. 1995. Steroid hormone signalling system and fungi. Comp Biochem Physiol B Biochem Mol Biol. 112(4):637–42. doi:10.1016/0305-0491(95)00113-1.
- Zhang H, Yohe T, Huang L, Entwistle S, Wu P, Yang Z, Busk PK, Xu Y, Yin Y. 2018. dbCAN2: a meta server for automated carbohydrate-active enzyme annotation. Nucleic Acids Res. 46(W1):W95–W101. doi:10.1093/nar/gky418.