Abstract
Three marine inlets across the southern Hikurangi margin, New Zealand, are investigated for evidence of palaeocoseismic subsidence, a signal associated with great subduction earthquakes. Microfossil analyses and radiocarbon-dated shells from core samples show that none of the sites have subsided since 2000 cal yr BP. Pauatahanui Inlet has remained at the present elevation since 7000 cal yr BP, while Big Lagoon and south-eastern Lake Wairarapa both subsided c. 4 m between 6000 and 2000 cal yr BP. At Big Lagoon most or all of the subsidence was tectonic. At south-eastern Lake Wairarapa, however, sediment compaction caused some or all of the subsidence. Neither of the subsided sites contain sedimentary or palaeoenvironmental transitions suggestive of sudden, coseismic relative sea-level rise. One possible palaeotsunami deposit was found at Big Lagoon, coincident with a colluvial deposit, and two liquefaction layers were identified in south-eastern Lake Wairarapa.
Introduction
Beneath the southern North Island, New Zealand, convergence between the Australian and Pacific plates occurs along the northwest-dipping Hikurangi subduction zone plate interface (A, B, E). Rupture of the subduction interface potentially represents the greatest seismic hazard to the central New Zealand region. However, there have been no subduction earthquakes in historic times (<150 years) and no palaeoseismic records of subduction earthquakes have been found along the southern Hikurangi margin. The hazard of subduction earthquakes along the Hikurangi margin is therefore highly uncertain at present (e.g. Wallace et al. Citation2009).
Figure 1 Tectonic setting and locality maps of the southern Hikurangi margin. A, Tectonic setting of the southern Hikurangi margin. Pacific plate motion rates in mm a–1 from De Mets et al. (1994). Dashed grey lines show depth contours of the subducting plate interface at 15 km and 40 km. MFS: Marlborough fault system; NIDFB: North Island dextral fault belt. B, Distribution of interseismic coupling at the Hikurangi margin interpreted from campaign GPS measurements (Wallace et al. 2009). C, Localities and active faults of the southern Hikurangi margin, encompassing the lower North Island and north-western South Island. Onshore faults are from the New Zealand Active Faults Database (http://data.gns.cri.nz/af/) and offshore faults are from Pondard & Barnes (2010). The three study areas are shown: Big Lagoon complex, SE Lake Wairarapa and Pauatahanui Inlet. LK: Lake Kohangapiri; LW: Lake Wairarapa. D, Coastal tectonics of the southern Hikurangi margin with areas of uplifted and subsided Holocene deposits and uplifted Pleistocene marine terraces. The number refers to the following references: 1. Marlborough Sounds, Singh (1997, 1998) and Hayward et al. (2010a); 2. Lower Wairau Valley, Brown (1981) and Ota et al. (1995); 3. South Wellington coast, Begg & Johnston (2000); 4. Miramar Peninsula, Pillans & Huber (1995); 5. Petone, Stirling (Citation1992); 6. Turakirae Head, McSaveney et al. (2006); 7. South Wairarapa coast, Ghani (1978) and Palmer et al. (Citation1989); 8. Southeastern Lake Wairarapa, Leach & Anderson (1974) and L. J. Brown (Greater Wellington Regional Council unpublished records, accessed 2006.); 9. Wairarapa coast, Ota et al. (1988, 1990) and Berryman et al. (1989). E, Simplified cross-section across the lower North Island.

Globally, almost all palaeoseismic records of subduction earthquakes have been obtained from the coastal zone (e.g. Atwater Citation1987; Darienzo et al. Citation1994; Hemphill–Haley 1995; Nelson et al. Citation1996, 2006; Zachariasen et al. Citation1999; Sawai et al. Citation2002; Nanayama et al. Citation2003; Cisternas et al. Citation2005; Melnick et al. Citation2006; Rajendran et al. Citation2008). Within the coastal zone, sea level can be used as a benchmark against which uplift and subsidence of the upper plate due to subduction earthquake deformation can be measured. Evidence for past subduction earthquakes includes subsided tidal marshes and coral reefs and, elsewhere, uplifted beaches; both are often associated with synchronous tsunami deposits. In the central Hikurangi margin sudden subsidence of intertidal sediments and associated tsunamis are probable evidence of past subduction earthquakes at c. 5550 and c. 7000 years BP (Cochran et al. Citation2006; Wallace et al. Citation2009). Along the southern Hikurangi margin pervasive active upper plate faulting causes coastal deformation (B, E; Pillans & Huber Citation1995; Cochran et al. Citation2007) making the isolation of subduction earthquake deformation challenging. In this study we focus upon three coastal inlets along the southern Hikurangi margin. We obtain Holocene palaeoenvironmental histories and examine this in the context of subduction earthquakes, upper plate faulting and changing sea level.
Tectonic setting
Deformation of southern North Island is dominated by the effects of oblique convergence between the Australian and Pacific Plates. The north-eastern South Island lies within the transition zone between oblique subduction (to the northeast) and continental transpression (to the southwest, A). Both regions are dominated by transpressional northeast-striking dextral strike–slip faults, known as the North Island Dextral Fault Belt (NIDFB) and the Marlborough Fault System (MFS, Fig. 1A). The offshore accretionary wedge is >100 km wide adjacent to the lower North Island and narrows rapidly towards the south (Barnes & Mercier de Lepinay Citation1997; Barnes et al. Citation1998). The plate interface dips westward beneath the region and lies at approximately 25 km depth beneath Wellington and Blenheim (E).
There are a number of reasons to infer that the subduction interface beneath the southern North Island is capable of generating large to great earthquakes. GPS data shows the plate interface is presently strongly interseismically coupled (B; Wallace et al. Citation2004) and seismicity delineates a c. 70 km wide locked zone on the plate interface across the southern North Island (Reyners Citation1998). Long-term geologic data indicate permanent shortening in the upper plate over the past 5 Ma accounting for only 20% of the margin-normal plate convergence; the remaining 80% is assumed to be accommodated by slip on the subduction interface (Nicol & Beavan Citation2003).
The most significant regional historical earthquake is the 1855 MW 8.2 Wairarapa earthquake which ruptured 120 km of the Wairarapa Fault and produced uplift and subsidence along the southern North Island coastline (C; Grapes Citation1989; Grapes & Downes Citation1997; McSaveney et al. Citation2006; Rodgers & Little Citation2006). It is possible that the deeper portion of the subduction interface (18–30 km depth) also ruptured in this event (Darby & Beanland Citation1992; Beavan & Darby Citation2005).
Study locations
The primary criterion for site selection was a history of Holocene intertidal sedimentation. Secondly, forward elastic dislocation modelling of a theoretical subduction zone earthquake was used to guide site selection towards locations more likely to undergo deformation in subduction earthquakes (Appendix 1). The largest coseismic subsidence is predicted to occur in the northern South Island while the Wellington region contains a mixture of uplift and subsidence due to its location near the down-dip transition from interseismic coupling to aseismic creep.
In the upper South Island two regions, Marlborough Sounds and Big Lagoon, were considered to have potential subduction earthquake records. Hayward et al. (Citation2010a) studied a number of sediment cores from the Marlborough Sounds and confirmed a subsidence rate of 0.2–0.8 mm a−1 but found no evidence of coseismic events. The Big Lagoon area, located at the south-eastern side of the Wairau Valley in the northern South Island (C, 2A), was selected because it has a historic record of coseismic subsidence (Eiby Citation1980; Grapes & Downes Citation1997) and geologic evidence of Holocene subsidence (Brown Citation1981; Ota et al. Citation1995). Historical accounts following the 1855 Mw 8.2 Wairarapa earthquake indicate that 0.6–1.5 m of subsidence occurred in the lower Wairau Valley, but it is not known if the subsidence was tectonic or due to soft sediment compaction.
The lower North Island coastline is generally characterised by rocky shore platforms and long-term tectonic uplift (D). Within this context there is limited scope for sheltered marginal marine sedimentation. The two study sites of south-eastern Lake Wairarapa and Pauatahanui Inlet were selected due to their preserved records of marginal marine sedimentation and their location away from high rates of coastal uplift (C, 1D). Unpublished water well logs indicate that the south-eastern Lake Wairarapa area is underlain by up to 40 m of Holocene marine sediment and may have Holocene subsidence rates of 2–6 mm a−1 (dates obtained by L. J. Brown in 1980 shown in ; records kept by Greater Wellington Regional Council).
Table 1 Radiocarbon ages obtained from the cores used in this study and the radiocarbon ages from the Pouawha well obtained by L. J. Brown in 1980.
Pauatahanui Inlet has a modern environment of broad intertidal flats and high tidal salt marshes. The present ecological conditions are suitable for recording relatively small changes in sea level (SL), and may have done so in the past. Previous studies of Pauatahanui Inlet have indicated the area has been stable or slightly uplifted relative to modern mean SL in the Holocene (Gibb Citation1986; Cochran et al. Citation2007).
Methods
Between two and four cores were obtained from each study site and most cores were collected using a truck-mounted hydraulic drill rig. Cores BL1 at Big Lagoon and RPC at Pauatahanui Inlet were collected using a vibracorer. Surveying of all sites was undertaken using a real-time kinematic (RTK) global positioning system (GPS) with reference to modern SL markers, such as high tide lines, and geodetic benchmarks. There are variable uncertainties ranging from ±0.1 m (Big Lagoon and Pauatahanui) to ±1 m (Lake Wairarapa), depending on what calibration points were used. Sediment compaction was corrected for on the cores from Big Lagoon and Lake Wairarapa using an estimated compaction factor based on the Paul & Barras (Citation1998) model. Compaction increases towards the middle of the sedimentary sequence and reaches a maximum of 10%. Sediment compaction corrections were not applied to the Pauatahanui Inlet cores because the sequence contained thick gravel units, assumed to be incompressible.
The sedimentology of all cores was logged by visual assessment and macrofauna, pollen, spore, foraminifera and diatom samples were selected at regular intervals or from specific units of interest. Palynological samples were initially treated with hot dilute hydrochloric acid and then treated with hydrofluoric acid and oxidised for 2–3 minutes with nitric acid and filtered through a 6 µm filter. All samples were mounted in stained jelly and one exotic Lycopodium tablet was added to determine rates of pollen sedimentation (number of pollen per unit weight of sediment). For diatom samples, the sediment (c. 1 g per sample) was digested in H2O2 to remove organic matter and heated in HCl to remove carbonates. Sand was removed by settling through a water column. Clay was removed by washing with dilute sodium hexa-metaphosphate and repeated decanting of suspended fines. Diatom slides were prepared by drying small aliquots of suspension onto cover slips and mounting them in Naphrax diatom mountant. Diatom valves were identified for each sample using standard reference floras (e.g. Krammer & Lange–Bertalot 1991, 1999a, 1999b, 2000, Hartley Citation1996; Witkowski et al. Citation2000). Foraminifera samples of c. 10 cm3 were sieved over a 63 µm mesh with the portion > 63 µm examined for foraminiferal content. Where possible, approximately 100 benthic foraminifera were counted and identified with reference to Hayward et al. (Citation1999).
Radiocarbon samples were dated using the accelerator mass spectrometry (AMS) technique at the Rafter Radiocarbon Laboratory, Lower Hutt, New Zealand. All shell samples were calibrated using the Marine04 calibration curve of Hughen et al. (2004) with a ▵13C of −30±13. Samples are presented as calibrated radiocarbon years before present (cal yr BP) with a 2-sigma uncertainty age range.
Results and environmental interpretation
Big Lagoon stratigraphy
The Big Lagoon complex consists of three connected lagoons: Big Lagoon, Upper Lagoon and Chandlers Lagoon (A). One core was collected from the southern margin of Big Lagoon (BL6) and three were collected from the margins of Chandlers Lagoon (BL1, 8 and 12); the cores reached depths of 6–10 m (). Seven radiocarbon ages have been obtained from the Big Lagoon complex cores (, ). Ages range from c. 1500 to 8500 cal yr BP (). A detailed modern analogue study of the Big Lagoon complex, along with the foraminiferal and diatom analyses of the Big Lagoon cores, has been described in Hayward et al. (Citation2010b); the core stratigraphy is therefore only briefly described here.
Figure 2 Shaded digital elevation maps of the three study sites. A, Map of the Big Lagoon complex in the lower Wairau Valley, see C for location. The four drill locations are shown along with the topography and onshore location of the Vernon Fault. B, Core locations within the lower Wairarapa Valley. Topography and active faults are shown (WF: Wairarapa Fault). The dotted white line indicates the course of the Ruamahanga River (note the locations of the natural and artificial river courses). Stippled light grey areas indicate Pleistocene marine terrace surfaces. PW: Pouawha Well. C, Map of the Pauatahanui Inlet area with the four drillcore locations. Also shown in light grey are the modern salt marsh areas and the Ohariu Fault trace. PO: Pauatahanui Orchard core.
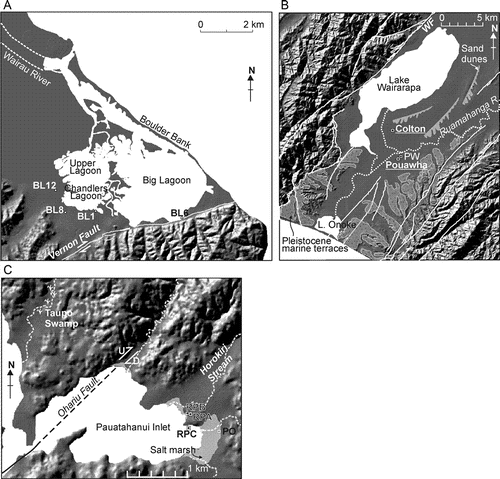
Figure 3 Stratigraphy and palaeoenvironmental interpretations of cores from the Big Lagoon complex (see Fig. 2 for the core locations). The first column for each core shows the grain size and presence of wood or shells along with the locations of radiocarbon ages. Columns 1–6 refer to different palaeoenvironmental data (see legends for further information); note not all cores have the same data available. In the middle of the figure the generalised palaeoenvironmental interpretation is shown; light grey dashed lines indicate correlations between the cores. provides radiocarbon sample details.
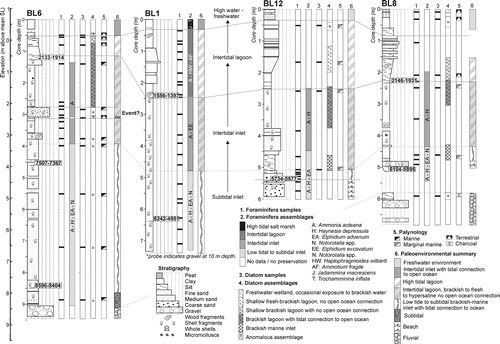
Gravels and coarse sands are at the base of most Big Lagoon cores. In BL6 and BL8 the gravels are non-fossiliferous, and in BL12 shell fragments occur. The BL1 core ends within silt but deeper probing indicates there are gravels at 10 m depth. Overlying the coarse basal sediments, and up to c. 2.5 m depth, are blue-grey silt and silty sand units with scattered shells; the shells are typically estuarine species such as Austrovenus stutchburyi and Cyclomactra tristi. Foraminifera assemblages containing Elphidium advenum and Haynesina depressula indicate a low-tide to subtidal environment, while diatoms suggest there was an open connection to the ocean (Fig. 3). At 5–3 m depth in all cores the foraminifera assemblages become dominated by Ammonia aoteana and H. depressula, indicating an intertidal environment; diatoms indicate that an open tidal connection to the ocean was maintained.
At 3.1 m depth in BL6, a silty clay unit and a coarse sand unit occur within the intertidal silt sequence; both units are bounded by sharp contacts. The silty clay has an anomalous diatom assemblage that represents a fluctuating environment or a mixed source influx event and the pollen assemblage is indicative of deposition in a terrestrial environment. The clay is barren of foraminifera, in contrast to surrounding units that have intertidal foraminifera. The overlying coarse sand at 3.09–2.75 m depth contains shell hash and has a clay matrix.
Within the upper 2.5 m of all cores is a transition to more variable, slightly coarser, orange-brown silt and sand units that do not have shells or foraminifera (except in BL1). Diatoms indicate limited to no connection to the open ocean and pollen is consistent with a decreasing marine influence. At 2.5–0 m depth in BL1 agglutinated foraminifera assemblages are dominated by Ammotium fragile and Haplophragmoides wilberti, typical of intertidal to high tidal environments with fluctuating salinities (Hayward et al. 2010b). In BL6 an influx of brackish-marine diatoms at 0.5 m depth occurs within a unit otherwise dominated by brackish, indifferent and soil diatom taxa. The top 0.2–0.3 m of each core in the Big Lagoon complex consists of soil A horizon. At the top of cores BL12 and BL8 are two A-horizons separated by a thin (2–5 cm) silty clay unit. The lower soil in BL8 at 0.28–0.10 m depth has pollen of a freshwater environment nearby, while the overlying silt contains diatoms and pollen indicative of a salt marsh environment with occasional exposure to brackish water.
Big Lagoon palaeoenvironments
The basal gravels in all cores are at variable elevations reflecting pre-existing topography (). The non-fossiliferous gravels of BL6 and BL8 are probably of fluvial origin, representing an early Holocene alluvial plain. The shelly gravels of BL12 probably represent a transgressive shoreline. Rising early Holocene SL saw inundation of the Big Lagoon area. This occurred at the earliest at BL6 at c. 8500 cal yr BP and later at BL12 and BL8 where the deepest dated marine sediments are 5600 and 6000 cal yr BP, respectively. The overlying silts and silty sands represent the subtidal environments of a sheltered inlet with a tidal connection to the open ocean. At depths between 3 and 5 m, all cores show a transition to an intertidal brackish inlet. This is dated at c. 5000 cal yr BP at Chandlers Lagoon and c. 7000 cal yr BP at Big Lagoon.
Within the intertidal sequence of BL6 is the anomalous silty clay unit at 3.1 m and the overlying coarse sand and shell hash. The silty clay, with its unusual (perhaps terrestrial) pollen and diatom assemblages, may be colluvium from the nearby hillslopes, perhaps shed during an earthquake. The chaotic and poorly sorted sand could be a palaeotsunami deposit. Neither the anomalous silty clay or the chaotic coarse sand and shell hash have unique and unambiguous signs of colluvium and tsunami deposition. However, their coupling suggests that a single event produced them. An earthquake explains slope collapse and marine inundation at the same time; the possible earthquake is not associated with a detectable relative SL change.
The transition at c. 2.5 m depth in all cores, associated with a change to coarser, non-fossiliferous silty sands and dated at c. 2000 cal yr BP, probably represents a change from an open to a closed lagoon. In BL1 this is recorded by the change to agglutinated foraminifera. Hayward et al. (Citation2010b) suggest the Wairau River changed course: instead of flowing into Big Lagoon it cut though the gravel barrier to the north thus diverting the strong currents of the Wairau River away from the water body of Big Lagoon.
The double A-horizons separated by clays at the top of BL12 and BL8 may represent subsidence associated with the 1855 earthquake (). The brackish silt between the soils contains introduced Pinus pollen, indicating deposition after the time of European occupation (i.e. post-1840). Alternative explanations for the buried soils include alteration of the drainage pattern in the area or recent SL rise (Gehrels et al. Citation2008). This same event may be associated with the anomalous diatom assemblage at 0.5 m depth in BL6.
Lake Wairarapa stratigraphy
Two cores were collected from the south-eastern side of Lake Wairarapa at Colton and Pouawha (B); both cores reached 18.3 m depth (). At 18.3–4 m depth, the Pouawha core consists of silt, clay and silty fine sand with scattered layers of articulated A. stutchburyi and Limnoperna securis shells (). L. securis is a small mussel species that typically lives attached to hard surfaces at low tide level, although it has been recorded as living at 2 m depth in a sandy lagoon (Hayward & Morley Citation2004). Two horizons c. 0.2 m thick of pervasively deformed sediments occur within the Pouawha core at 15.5 and 17.5 m, but otherwise the sediments are horizontally bedded (). The foraminiferal samples from Pouawha show generally either poor fossil occurrence or low preservation (), but at two intervals of c. 7–9 m depth and 15–16.5 m depth are clusters A. aoteana. A diatom sample at 18.1 m is dominated by Cyclotella menghiniana, Cocconeis placentula and Achnanthes pusilla, species associated with fluvial to fresh-brackish environments. Up core, the diatom assemblages at 4, 7.6 and 10.9 m are dominated by brackish-marine species such as Catenula adhaerens, Cocconeis spp. and Melosira spp. These taxa indicate a brackish marine estuarine environment with limited marine connectivity. From 18.3 m to 5.5 m depth, the Colton core consists of laminated to massive blue-grey clay with scattered to rare shells. Foraminifera preservation is poor; only three samples between 9 m and 13 m depth yielded intertidal foraminifera (A. aoteana and E. excavatum).
Figure 4 Stratigraphy and palaeoenvironmental interpretations of cores from south-eastern Wairarapa. See Fig. 3 for the stratigraphy legend and Fig. 2 for the core locations.
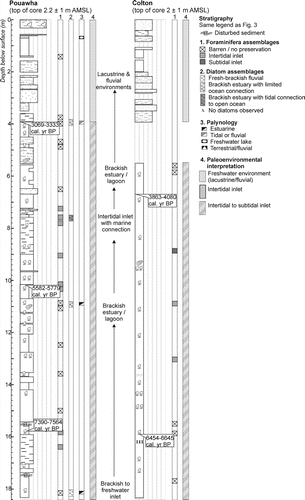
Figure 5 Core photos and sketches of the disturbed sedimentary horizons within the Pouawha core from south-eastern Lake Wairarapa. The deformation in the core on the left is likely to be related to seismic shaking, the deformation in the core on the right has some features that could be related to bioturbation but it may also be seismic shaking-induced.
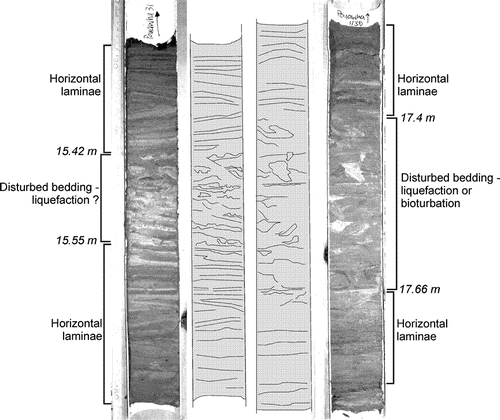
At approximately 4 m depth in both cores is a transition to more variable and slightly coarser sediments: alternating clay, silt and fine sand units with no shells or foraminifera. A pollen sample from Pouawha at 0.68 m depth indicates a freshwater environment with abundant sedge pollen, consistent with a non-marine floodplain environment.
Lake Wairarapa palaeoenvironments
The silts and silty fine sand below 4 m depth in the Pouawha core are similar to modern sedimentary environments found in Lake Onoke (see facies descriptions in Woolfe Citation1993). Lake Onoke, located on the coast 14 km south of Lake Wairarapa (), is separated from the sea by a gravel barrier. The water level, tidal exposure and salinity of the lake fluctuate depending on the interplay between storm waves, sediment supply and river input. We suggest the Pouawha palaeoenvironment from 18.3–4 m was of an estuary or sheltered inlet similar to Lake Onoke; this would explain the patchy occurrence of marine microfossils as salinity levels probably varied. The deformed sediment units at 15.5 m and 17.5 m in the Pouawha core probably represent some type of disturbance event such as liquefaction, submarine landsliding or intense bioturbation (). The Colton core is finer grained and more homogenous than Pouawha; we suggest the Colton sediments represent a deeper or more sheltered part of an inlet or estuary relative to Pouahwa. Radiocarbon ages show the marine-influenced sediments of Pouawha 1 and Colton 1 span the mid-Holocene from c. 7400 to 3200 cal yr BP.
The coarser, more variable sediments above 4 m depth in both cores are probably from a fluvial or overbank flooding environment. In the Pouawha core the transition occurs at c. 3200 cal yr BP; in the Colton core it is after c. 3950 cal yr BP (). Leach & Anderson (Citation1974) also found evidence for a palaeoenvironmental change from marine to non-marine in the Lake Wairarapa area at approximately 3400 cal yr BP. Our data are consistent with Leach & Anderson (Citation1974) although they suggest the transition may have occurred later at 3200 cal yr BP; however, the transition from marine inlet to fluvial may have been diachronous across the region.
Pauatahanui Inlet stratigraphy
Four cores, referred to as Pauatahanui Orchard, Ration Point A (RPA), Ration Point B (RPB) and Ration Point C (RPC), were obtained from the north-eastern margins of Pauatahanui Inlet (C, 6). The Pauatahanui Orchard core, reaching a depth of 5.4 m, was obtained from the margins of the salt marsh. Consisting of very stiff, weathered silt and silty sand units, the Pauatahanui Orchard core sediments are interpreted as representing last glacial fluvial and/or loess deposits. No marine sediments were evident so there were no prospects of obtaining a relative SL record.
Both the RPA and RPB cores were located at the seaward edge of a relict sand ridge. The RPA core reached a depth of 5.6 m; however, the core was not collected from 2.9 to 4.8 m because it was gravel (). The RPB core was collected approximately 10 m away from RPA and reached a depth of 4.2 m; the upper 1.1 m of anthropogenic fill was not collected. Sediment from the base of the cores up to 2 m depth in RPA and 1.8 m depth in RPB consists of alternating decimetre-scale peat, silty sand and poorly sorted gravel units; there are sharp contacts between the units and unit thicknesses are variable (most gravel units cannot be correlated between the cores). Foraminifera are absent but rare diatoms species such as Tryblionella levidensis and Cocconeis placentula in RPA suggest a fresh to fresh-brackish environment. The broken and etched nature of many of the pollen grains indicates an active depositional environment with either tidal or fluviatile currents. In cores RPA and RPB, silty fine sand with scattered A. stutchburyi and Paphies australis shell fragments occur at 2–1.5 m and 1.8–1.1 m depth, respectively. Intertidal foraminifera such as Ammonia spp. are present, as are rare tidal flat diatom species ().
Figure 6 Stratigraphy and palaeoenvironmental interpretations of cores from Pauatahanui Inlet (see for the core locations). Note that the scale for Ration Point C is different from the other two cores. The Pauatahanui Orchard core log is not shown as it was not used for palaeoenvironmental studies. See for the legend.
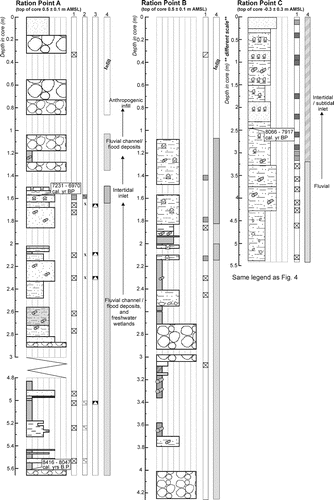
The RPC core was collected from mid-low tide elevation at the south-eastern side of Ration Point and reached 5.4 m depth (). The basal part of the core (and up to 3.4 m) is mud and sand with occasional wood fragments and pebbles; foraminifera are absent. From 3.4 m depth to the top of the core are sand and sandy mud units with scattered to concentrated beds of estuarine shells such as A. stutchburyi, P. australis and Mactra ovata. Ten foraminifera samples from 3.2 m depth to the top of the core indicate a marine history of initially intertidal sedimentation deepening to subtidal and then shallowing to intertidal ().
Pauatahanui Inlet palaeoenvironments
We suggest the alternating peat, silt and gravel units in the lower parts of core RPA and RPB represent marshlands (peat) subject to periodic flooding of the nearby Horokiri Stream, which deposited the sands and gravels (). Peat at the base of RPA has an age of 8047–8416 cal yr BP, a time when SL was 10±5 m below present mean SL (, ). The silty shelly sediments in the middle sections of RPA and RPB represent an intertidal inlet palaeoenvironment. A shell radiocarbon age of 7231–6970 cal yr BP within the intertidal sediments of RPA coincides with the culmination of SL rise in the New Zealand region. The upper part of both cores consists of flood gravel and anthropogenic fill. The lower part of the RPA core up to 3.4 m depth is interpreted to be fluvial sediment because it contains wood fragments and no foraminifera. An age of 8066–7917 cal yr BP was obtained from 2.48 m at the boundary between the intertidal and subtidal facies (, ); this sample pre-dates the SL rise culmination by approximately 500–1000 years. The overlying shell and foraminifera-bearing sands and muds represent a deepening then shallowing inlet environment, reflecting sediment infilling under rising and stabilising SL.
Discussion
Evidence of subsidence
In this section we compare the ages and elevations of the marine sediment to the regional SL curve to determine if there has been any net subsidence at the study sites in the Holocene. Some amount of relative SL rise should be recorded in the stratigraphic record if there is either (1) coseismic subsidence and not all the subsidence is recovered during the intersesimic period, or (2) rising SL (i.e. submergence), creating accommodation space (A–C).
Figure 7 Simplified illustrations showing how event stratigraphy is preserved or not preserved within an intertidal inlet under different amounts of subsidence or SL change. A, If a sequence of intertidal sediment is undergoing sedimentation near the top of the intertidal depth range, then >1.3 m of subsidence is required to change the environment. B, If a sequence of intertidal sediment is undergoing sedimentation near the top of the intertidal depth range and <1.3 m of subsidence occurs then the environment may not be noticeably changed, i.e. no evidence of an event preserved. C, If a sequence of intertidal sediment is undergoing sedimentation near the base of the intertidal depth range then an event of <1.3 m can change the environment. D, This scenario illustrates how evidence of a subsidence event may be eroded from the sedimentary sequence under conditions of complete interseismic recovery and stable SL. E, This scenario shows how under stable SL the stratigraphic evidence of a subsidence event may be preserved if there is incomplete interseismic recovery. F, This scenario shows that even with complete interseismic recovery of coseismic subsidence, rising SL will help to preserve evidence of the event.
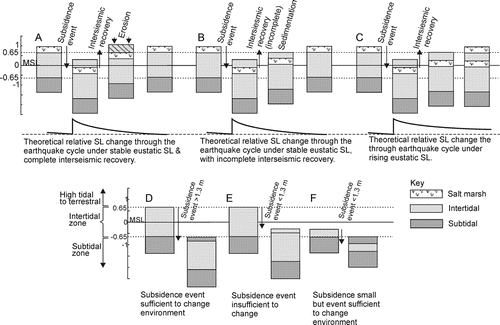
In the New Zealand region, SL reached present levels at approximately 7500 to 7000 cal yr BP (Gibb Citation1986; Clement et al. Citation2008, 2010). Prior to that time, SL had been rising rapidly at rates of around 9–10 mm a–1 (). From 7000 cal yr BP to present, the Gibb (Citation1986) SL curve suggests SL has remained approximately stable. However, a recent review of New Zealand SL data by Clement et al. (Citation2008, Citation2010) suggests that SL stabilised at 7550 cal yr BP and was 0.5–1 m higher than modern mean SL from 7550 to 2000 cal yr BP. Further studies also suggest there may have been a 1.2–1.5 m mid-Holocene highstand in New Zealand (Schofield Citation1960; Hicks & Nichol Citation2007; Kennedy Citation2008), consistent with other studies from the southwest Pacific (e.g. Sloss et al. Citation2007; Switzer et al. Citation2010). There has also been a SL rise of c. 0.1 m since 1500 AD and a c. 0.25 m rise in SL during the past 100 years in New Zealand (Gehrels et al. Citation2008). We present the SL curves of Clement et al. (Citation2010) and Gibb (Citation1986) in Fig. 7 to compare with the results of this study.
Figure 8 Age and elevation (plus uncertainties) of radiocarbon samples from the Big Lagoon complex, south-eastern Lake Wairarapa and Pauatahanui Inlet cores relative to the New Zealand SL curve. The grey shaded area represents the calibrated ages from Gibb (1986) and the dashed grey curve is from Clement et al. (2008); also shown is the Gehrels et al. (2008) SL curve for the past 2000 years. Radiocarbon ages are in calibrated years BP with a 2-sigma uncertainty. Samples that plot near the grey shaded area indicate no vertical movement since deposition; samples that plot below the curve indicate subsidence since deposition. See for the parameters used to calculate sample elevation. The lower figures shows a summary of the vertical tectonic motions of the three southern Hikurangi margin study sites relative to the New Zealand region SL curve (Gibb 1986; Clement et al. 2008). Dark grey arrow indicates approximate tectonic stability.
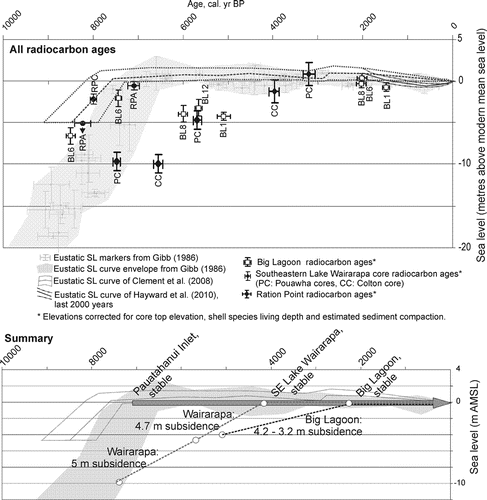
Subsidence was calculated as:
Subsidence=[present elevation of C14 sample + preferred living depth of dated mollusc + sediment compaction] – [SL at time of mollusc death].
The parameters used to calculate net subsidence are listed in and the elevation of the samples relative to past SL is shown in . We assume the dated molluscs are preserved in their living positions. In most cases the molluscs are well preserved and consistent with the depositional depth of the surrounding sediment as determined by foraminifera (e.g. A. stutchburyi living within sand containing intertidal foraminifera assemblages) so we are confident there is no reworking. An exception to this is a Maoricolpus roseus sample from BL8, a species that typically lives subtidally. The surrounding sediment is more consistent with a beach environment, so we assign this sample a ‘living’ depth of 0±1 m.
Table 2 Parameters used to calculate the elevation of all radiocarbon samples relative to modern mean sea level (used in ).
At Big Lagoon, the two>7000 cal yr BP samples from the BL6 core do not indicate subsidence (). Given the considerable uncertainty in the SL curve prior to 7000 cal yr BP, however, we do not draw any conclusions regarding tectonic uplift or subsidence from the older ages. Elevation and age relations of samples obtained from the Big Lagoon cores show (3.2±1)–(4.2±0.5) m of subsidence occurred between approximately 6000 and 2000 cal yr BP (). If there was a 1 m highstand during this period then the total subsidence may have been up to 5.2±0.5 m ().
There has been no tectonic subsidence in the past 2000 cal yr BP except for the effects of 1855 Wairarapa earthquake, discussed below ().
The three radiocarbon ages from Pauatahanui Inlet indicate there has been no tectonic subsidence of this area during the Holocene. An estuarine shell in RPC at 2.3±0.6 m depth was deposited during a period of rising SL at c. 8000 cal yr BP; it is therefore consistent with Holocene stability (). An estuarine shell in the RPA core dated c. 7000 cal yr BP is presently located near mean SL, indicating no vertical tectonic movement since SL stabilisation ().
In the south-eastern Lake Wairarapa area, the oldest sample (7564–7390 cal yr BP) comes from the Pouawha core. It has an elevation of −9.9±1.1 m a.m.s.l.; this falls within the SL curve envelope of Gibb (Citation1986) but is approximately 10 m below the SL curve envelope of Clement et al. (Citation2008); ). Without better clarification of SL at this time, this sample has an ambiguous subsidence signal. Mid-Holocene radiocarbon samples suggest there may have been up to 10±1.1 m of subsidence (). A shell dated at 5799–5582 cal yr BP from Pouawha 1 has a corrected elevation of –4.9±1.1 m a.m.s.l. and a shell dated at 6645–6454 cal yr BP in the Colton 1 core has a corrected elevation of −10.2±1.1 m a.m.s.l. Both dated specimens were intertidal shells (A. stutchburyi) and, in the case of the Pouawha 1 core, the sample was surrounded by intertidal foraminifera. These data suggest that 4.9±1.1 m of tectonic subsidence occurred at the Pouawha site during c. 5600–3200 cal yr BP, and that 10.2 ± 1.1 m of tectonic subsidence occurred at the Colton site during 6500–4000 cal yr BP. However, particularly for the Colton 1 core, the palaeoenvironmental control on the dated samples is weak. There is a possibility that the samples were from a subtidal environment, in which case an accurate estimate of the amount of tectonic subsidence cannot be made. Samples younger than 4000 cal yr BP indicate no late Holocene tectonic subsidence at south-eastern Lake Wairarapa ().
In the Big lagoon area, the mid-Holocene subsidence is most likely of tectonic origin; we have good palaeoenvironmental control and have made reasonable assumptions about the amount of compaction. In the Lake Wairarapa area, the mid-Holocene subsidence signal could be entirely due to sediment compaction. Water well data shows there is approximately 40 m of silty sandy sediment overlying gravels at the Pouawha and Colton core site; the gravels are approximately 8 m thick and are then underlain by another 40 m of silty sandy sediment. While our calculations of subsidence in the Colton and Pouawha cores take into account up to 10% sediment compaction in the upper 40 m of silt, we have no method of estimating the ongoing compaction of the deeper, pre-Holocene sediment. This compaction may be responsible for the apparent subsidence of the Holocene sediments.
Evidence of coseismic events
Evidence of coseismic events may be recorded by sudden changes in relative SL and/or palaeotsunami deposits (e.g. Nelson et al. Citation1996; Clague Citation1997; Cisternas et al. Citation2005). Supporting evidence may include liquefaction of sediments and landsliding. There has been Holocene tectonic subsidence at Big Lagoon but there is no evidence of coseismic subsidence, except for in the upper 0.5 m of cores BL6, BL8 and BL12 where subsidence of <0.2 m is probably associated with the 1855 Wairarapa earthquake. The deeper sections of the Big Lagoon cores contain sediment deposited between 6000 and 2000 cal yr BP when the radiocarbon ages and palaeoecology imply there was (3.2±1)–(4.2±0.5) m of subsidence. If this subsidence occurred coseismically we would expect a palaeoenvironmental signal of SL sudden rise. The core data actually suggest a general trend of gradual relative SL lowering as the palaeoenvironments progress from subtidal to intertidal to high tidal and terrestrial ().
Two possible interpretations regarding the mid-Holocene (6000 and 2000 cal yr BP) tectonic subsidence in the Big Lagoon complex are: (1) the tectonic subsidence of Big Lagoon occurred gradually and at slower rates than sediment infilling, thus relative SL rise is not detectable; or (2) tectonic subsidence of Big Lagoon, by events on upper plate faults or the subduction interface, occurred coseismically but our core locations did not capture suitable palaeoenvironments to record the changes. The temporal variability in subsidence (i.e. no subsidence in the past 2000 years but high rates from 2000–6000 cal yr BP) suggests it does not have a gradual and, by inference, continuous cause. The insensitivity of our palaeoenvironments is a more likely reason for the lack of events. For the purpose of this type of study we would prefer to have captured palaeoenvironments containing fauna and flora with narrower depth and salinity tolerances and greater sensitivity to SL changes (e.g. high water to extreme high water environments), but our cores mostly captured intertidal environments.
At Big Lagoon we can use palaeoenvironmental control to place constraints on the maximum amount of displacement by past earthquakes. Most of the Big Lagoon cores consist of intertidal deposits that have a depth range of 0.65 to –0.65 m (based on a spring tidal range of c. 1.3 m). Therefore a coseismic subsidence event would have to cause>1.3 m of subsidence to alter the environment to a degree that we could reliably detect in our palaeoenvironmental analysis (D–F). This assumes that post-event sediment mixing has not erased or blurred the event horizon. As a result, we can be confident that no coseismic subsidence events causing greater than >1.3 m occurred at Big Lagoon in the past c. 7000 years. Elastic dislocation models of a subduction rupture (Appendix) estimate that c. 1.3 m of subsidence may occur given a 1300 year recurrence earthquake. Our results suggest subduction earthquakes may have a recurrence interval <1300 years along the southern Hikurangi margin, although this is contingent on the dislocation modelling being a true reflection of past upper plate deformation and relies on negative evidence of no large subsidence events.
The single probable coseismic subsidence event seen in this study is from the upper parts of the Big Lagoon cores. The event is marked in BL8 and BL12 by a 2 cm silt layer overlying a palaeosol (). Pollen evidence of introduced tree species confirms it happened in historic times (post-1830s in the Wairau Valley region). The amount of subsidence at BL12 was <0.15 m and <0.1 m at BL8 (the depth between the present ground surface and the upper contact of the buried soil; ). These measurements are significantly less than the historical accounts of 0.6–1.5 m of subsidence around the Big Lagoon area following the 1855 earthquake (Grapes & Downes Citation1997). Hayward et al. (2010b) observed a subsided peat soil near the north-western margin of Upper Lagoon that attests to 0.5 m of subsidence in a very localised area; they suggest this was liquefaction-related subsidence in the 1855 earthquake. Our results of <0.15 m of subsidence over the wider area add support to a locally variable mode of subsidence in 1855 such as sediment compaction or liquefaction, rather than a more regional tectonic subsidence signal.
Within the BL6 core at Big Lagoon there is evidence of an event at c. 3 m depth, but it is not associated with a relative SL change. At 3.1 m depth is a silty clay unit with anomalous diatom, pollen and foraminifera assemblages, overlain by a coarse sand and shell hash (). We suggest this may be a colluvial layer overlain by a tsunami deposit. If correct, the coseismic unit may be associated with a subduction earthquake that produced shaking and a tsunami but no significant upper plate subsidence, or it could have been caused by an event on a nearby upper plate fault. Both the Wairau and Vernon Faults have been active during the Holocene so are candidates for causing strong shaking at Big Lagoon (Zachariasen et al. Citation2006; Pondard & Barnes Citation2010).
A similar result is obtained from the south-eastern Lake Wairarapa cores where Holocene subsidence has occurred, but we cannot determine if the subsidence was gradual or coseismic because our palaeoenvironmental data cannot resolve small changes in relative SL. We do however observe that the stratigraphy of the Lake Wairarapa cores are remarkably homogenous, with the exception of two horizons of deformed sediment in the Pouawha core at 15.4 and 17.5 m depth (, ). An explanation for deformed sediment is disturbance due to seismic shaking, although the horizon at 17.5 m displays some features also characteristic of bioturbation (). A radiocarbon age of 7390–7564 cal yr BP at 15.85 m depth suggests the deformed horizon at 15.4 m may be close to 7100 cal yr BP, coinciding with the timing of a probable subduction earthquake along the central Hikurangi margin (Cochran et al. Citation2006). It also coincides with the time range of a likely seismic event recorded in Lake Kohangapiripiri, 70 km east-southeast of Lake Wairarapa (C, Cochran et al. Citation2007). An equivalent deformed horizon at c. 7100–7400 cal yr BP is not seen in the Colton core, possibly because the core does not cover that age range or due to its generally higher clay content making it more cohesive.
There is no evidence of coseismic events within the Pauatahanui Inlet cores. No net subsidence is recorded and the thin sequence of intertidal sediments within RPA and RPB do not contain sufficient microfossils to undertake high-resolution SL studies. In RPC the sequence of intertidal-subtidal-intertidal is consistent with valley infilling under SL transgression and stabilisation.
The results presented here are the first advances towards understanding the plate interface palaeoseismicity along the southern Hikurangi margin. While no unequivocal subduction earthquakes have been identified, a number of interesting events in the palaeoenevironmental history of the intertidal inlets have been identified; each of these are worthy of further investigations. With the positive identification of metres of mid-Holocene subsidence at Big Lagoon and at south-eastern Lake Wairarapa, these two areas have the greatest potential for preserving evidence of subduction earthquakes. This is particularly true if more marginal marine palaeoenvironments can be located in the geologic record. The greatest contrast between the southern Hikurangi margin and other subduction margins with long palaeoseismic records such as Cascadia, southern Chile and Sumatra is the complexity of upper plate faulting. The best method to overcome this will be to obtain widespread evidence of subduction earthquakes that can be correlated across the various upper plate fault blocks. If only localised evidence of tectonic subsidence is recognised (i.e. within one fault block) then the timing of the subsidence event could be compared to nearby upper plate faults to determine if they are coincident (e.g. see the comparisons in Cochran et al. Citation2007). Subsidence events anti-correlated to upper plate fault ruptures could be an indicator of a subduction zone event.
Conclusions
We have investigated the Holocene palaeoenvironmental histories of three intertidal inlets across the southern Hikurangi margin. Evidence of subsidence which occurred between c. 6000 and 2000 cal yr BP was found at Big Lagoon in the lower Wairau Valley and the south-eastern Lake Wairarapa area. There have been no elevation changes at all three sites during the past 2000 cal yr BP. At Big Lagoon, the mid-Holocene subsidence was tectonic in origin but we cannot determine if it was gradual or coseismic. If it was coseismic, however, palaeoenvironmental constraints imply there was probably <1.3 m of subsidence per event. The occurrence of anomalous sediments at 3 m depth in one Big Lagoon core (BL6) may be related to a local earthquake that caused a tsunami and groundshaking, but no subsidence. In the south-eastern Lake Wairarapa area, the mid-Holocene subsidence could be tectonic or related to sediment compaction. A deformed sediment horizon dated c. 7100 years may be related to widespread seismic shaking, possibly correlated to a subduction earthquake along the central Hikurangi margin. No net Holocene tectonic deformation was found at Pauatatahanui Inlet.Footnote1
Supplementary data: Tables of all microfossil data are available in Supplementary Files 1–3.
tnzg_a_562903_sup_19430558.doc
Download MS Word (172.5 KB)tnzg_a_562903_sup_19925874.zip
Download Zip (69 KB)tnzg_a_562903_sup_19430531.doc
Download MS Word (517.5 KB)tnzg_a_562903_sup_19430580.doc
Download MS Word (83.5 KB)Acknowledgements
This work was funded by the Foundation of Research Science and Technology and by the Earthquake Commission as part of the ‘It's Our Fault’ project. Landowners Ted Colton, Frazer Blazek, Judy Preston, Janet and Phillip Reidy and Paul and Leigh Leedom are thanked for allowing access to their land for drilling. The Greater Wellington Regional Council, particularly Doug McAllister, is thanked for providing well data from the Lake Wairarapa area. We are especially appreciative of Peter Barker and William Ries for operating the drill truck and ensuring superb core retrieval. Reviews of an earlier version of this work by Russ Van Dissen, Nicola Litchfield and Kelvin Berryman, and reviews of this manuscript by Scott Nichol and Brian Sherrod, were appreciated.
Notes
Supplementary data available at www.tandfonline.com/10.1080/00288306.2011.562903
Supplemental File 1: Microfossil data from Big Lagoon. (A) Foraminifera census, (B) summary of pollen results, (C) diatom census and summary of results.
Supplemental File 2: Microfossil data from south-eastern Lake Wairarapa. (A) Foraminifera census, (B) summary of pollen results, (C) diatom census and summary of results.
Supplemental File 3: Microfossil data from Pauatahanui Inlet. (A) Foraminifera census, (B) summary of pollen results, (C) summary of diaton results.
References
- Atwater , BF . 1987 . Evidence for great Holocene earthquakes along the outer coast of Washington State . Science , 236 : 942 – 944 .
- Barnes , PM and Mercier de Lepinay , B . 1997 . Rates and mechanics of rapid frontal accretion along the very obliquely convergent southern Hikurangi margin, New Zealand . Journal of Geophysical Research , 102 ( B11 ) : 24931 – 24952 .
- Barnes PM , Mercier de Lepinay B , Collot J–Y , Delteil J , Audru J–C 1998 . Strain partitioning in the transition area between oblique subduction and continental collision, Hikurangi margin, New Zealand . Tectonics 17 ( 4 ): 534 – 557 .
- Beavan J , Darby D 2005 . Fault slip in the 1855 Wairarapa earthquake beased on new and reassessed vertical motion observations: did slip occur on the subduction interface? In : Townend J The 1855 Wairarapa Earthquake Symposium . Pp. 31 – 41 .
- Begg J , Johnston M 2000 . Geology of the Wellington area . Institute of Geological and Nuclear Sciences 1:250000 Geological Map 10: 1 sheet+64 pp .
- Berryman , KR , Ota , Y and Hull , AG . 1989 . Holocene palaeoseismicity in the fold and thrust belt of the Hikurangi subduction zone, eastern North Island, New Zealand . Tectonophysics , 163 : 185 – 195 .
- Brown , LJ . 1981 . Late Quaternary geology of the Wairau Plain, Marlborough, New Zealand . New Zealand Journal of Geology and Geophysics , 24 : 477 – 490 .
- Cisternas M , Atwater B , Torrejon F , Sawai Y , Machuca G , Lagos M , Eipert A , Toulton C , Saldago I , Kamataki T and others 2005 . Predecessors of the giant 1960 Chile earthquake . Nature 437(doi:10.1038/nature03943) : 404 – 407 .
- Clague , JJ . 1997 . Evidence for large earthquakes at the Cascadia subduction zone . Reviews of Geophysics , 35 : 439 – 460 .
- Clement AJ , Sloss CR , Fuller IC 2008 . Holocene sea–level change in New Zealand: a review . IGCP #495 Annual Conference . Pp. 46 – 47 .
- Clement , AJ , Sloss , CR and Fuller , C . 2010 . Late Quaternary geomorphology of the Manawatu coastal plain, North Island, New Zealand . Quaternary International , 221 : 36 – 45 .
- Cochran , U , Berryman , K , Mildenhall , D , Hayward , B , Southall , K , Hollis , C , Barker , P , Wallace , L , Alloway , B and Wilson , K . 2006 . Palaeoecological insights into subduction zone earthquake occurrence, eastern North Island, New Zealand . Geological Society of America Bulletin , 118 ( 9/10 ) : 1051 – 1074 .
- Cochran , UA , Hannah , M , Harper , MA , Van Dissen , R , Berryman , K and Begg , J . 2007 . Detection of large, Holocene earthquakes using diatom analysis of coastal sedimentary sequences, Wellington . New Zealand Quaternary Science Reviews , 26 ( 7–8 ) : 1129 – 1147 .
- Darby , D and Beanland , S . 1992 . Possible source models for the 1855 Wairarapa earthquake, New Zealand . Journal of Geophysical Research , 97 : 12375 – 12389 .
- Darienzo , ME , Peterson , CD and Clough , C . 1994 . Stratigraphic evidence for great subduction-zone earthquakes at four estuaries in Northern Oregon, U.S.A . Journal of Coastal Research , 10 : 820 – 876 .
- De Mets , C , Gordon , RG , Argus , DF and Stein , S . 1994 . Effect of recent revisions to the geomagnetic reversal timescale on estimates of current plate motions . Geophysical Research Letters , 21 : 2191 – 2194 .
- Eiby GA 1980 . The Marlborough earthquakes of 1848 . DSIR Bulletin 225: 82p .
- Gehrels R , Hayward B , Newnham R , Southall K 2008 . A 20th century acceleration of sea-level rise in New Zealand . Geophysical Research Letters 35: LO2717 , doi: doi:10.1029/2007GL032632 .
- Ghani , MA . 1978 . Late cenozoic vertical crustal movements in the southern North Island, New Zealand . New Zealand Journal of Geology and Geophysics , 21 ( 1 ) : 117 – 25 .
- Gibb JG 1986 . A New Zealand regional Holocene eustatic sea-level curve and its application to determination of vertical tectonic movements, a contribution to IGCP Project 200 . Recent Crustal Movements of the Pacific Region. Bulletin of the Royal Society of New Zealand . 24: 377 – 395 .
- Grapes , R . 1989 . The Wairarapa Fault: displacements and palaeoseismicity . Bulletin of the New Zealand National Society for Earthquake Engineering , 22 ( 1 ) : 13 – 16 .
- Grapes , R and Downes , G . 1997 . The 1855 Wairarapa, New Zealand, Earthquake-Analysis of Historical Data . Bulletin of the New Zealand National Society for Earthquake Engineering , 30 : 271 – 368 .
- Hartley B 1996 . An Atlas of British Diatoms. Biopress Ltd, Bristol .
- Hayward B , Morley M 2004 . Intertidal life around the coast of the Waitakere Ranges, Auckland . Auckland Regional Council Technical Publication No. 298 .
- Hayward B , Grenfell HR , Sabaa A , Kay J , Daymond–King R , Cochran U 2010a . Holocene subsidence at the transition between strike-slip and subduction on the Pacific–Australian plate boundary, Marlborough Sounds, New Zealand . Quaternary Science Reviews 29 : 648 – 661 , doi: doi:10.1016/j.quascirev.2009.11.021 .
- Hayward , B , Wilson , KJ , Morley , M , Cochran , U , Grenfell , HR , Sabaa , A and Daymond–King , R . 2010b . Microfossil record of the Holocene evolution of coastal wetlands in a tectonically active region of New Zealand . The Holocene , 30 ( 3 ) : 405 – 421 .
- Hayward BW , Grenfell HR , Reid C , Hayward K 1999 . Recent New Zealand shallow-water benthic foraminifera: taxonomy, ecologic distribution, biogeography, and use in palaeoenvironmental assessment., Institute of Geological and Nuclear Sciences Monograph 21 . 264 p. Lower Hutt, New Zealand: Institute of Geological and Nuclear Sciences Limited .
- Hemphill–Haley , E . 1995 . Diatom evidence for earthquake-induced subsidence and tsunami 300 yr ago in southern coastal Washington . Geological Society of America Bulletin , 107 : 367 – 378 .
- Hicks , H and Nichol , SL . 2007 . A marine to freshwater sediment succession from Kowhai Beach wetland, Northland: implications for Holocene sea level . Journal of the Royal Society of New Zealand , 37 ( 3 ) : 91 – 107 .
- Hughen KA, Baillie MGL, Bard E, Beck JW, Bertrand CJH, Blackwell PG, Buck CE, Burr GS, Cutler KB, Damon PE, Edwards RL, Fairbanks RG, Friedrich M, Guilderson TP, Kromer B, McCormac G, Manning S, Ramsey BB, Reimer PJ, Reimer RW, Remmele SS Jr, Stuiver M, Talamo S, Taylor FW, van der Plicht J, Weyhenmeyer CE 2004 . Marine04 marine radiocarbon age calibration, 0–26 Cal Kyr BP. Radiocarbon 46: 1059–1086.
- Kennedy , DM . 2008 . Recent and future higher sea levels in New Zealand: a review . New Zealand Geographer , 64 : 105 – 116 .
- Krammer K , Lange–Bertalot H 1991 . Bacillariophyceae: Achnanthaceae . Gustav Fischer Verlag, Stuttgart .
- Krammer K , Lange–Bertalot H 1999a . Bacillariophyceae: Naviculaceae. Specktrum Akademischer Verlag, Heidelberg .
- Krammer K , Lange–Bertalot H 1999b . Bacillariophyceae: Bacillariaceae, Epithemiaceae, Surirellaceae . Specktrum Akademischer Verlag, Heidelberg .
- Krammer K , Lange–Bertalot H 2000 . Bacillariophyceae: Centrales, Fragilariaceae, Eunotiaceae . Spektrum Akademischer Verlag, Heidelberg .
- Leach , BF and Anderson , AJ . 1974 . The transformation from an estuarine to lacustrine environment in the lower Wairarapa . Journal of the Royal Society of New Zealand , 4 ( 3 ) : 267 – 275 .
- McSaveney , M , Graham , I , Begg , J , Beu , A , Hull , A , Kim , K and Zondervan , A . 2006 . Late Holocene uplift of beach ridges at Turakirae Head, south Wellington coast . New Zealand New Zealand Journal of Geology and Geophysics , 49 : 337 – 358 .
- Melnick D , Bookhagen B , Echtler H , Strecker M 2006 . Coastal deformation and great subduction earthquakes, Isla Santa Maria, Chile (37S) . Geological Society of America Bulletin 118 ( 11/12 ): 1463 – 1480 ; doi: doi: 10.1130/B25865.1 .
- Nanayama , F , Satake , K , Furukawa , R , Shimokawa , K , Atwater , BF , Shigeno , K and Yamaki , S . 2003 . Unusually large earthquakes inferred from tsunami deposits along the Kuril trench . Nature , 424 : 660 – 663 .
- Nelson AR , Kelsey HM , Witter RC 2006 . Great earthquakes of variable magnitude at the Cascadia subduction zone. Quaternary Research 65 (3) : 354–365 ; doi: doi: 10.1016/j.yqres.2006.02.009 .
- Nelson , AR , Shennan , I and Long , AJ . 1996 . Identifying coseismic subsidence in tidal-wetland stratigraphic sequences at the Cascadia subduction zone of western North America . Journal of Geophysical Research , 101 : 6115 – 6135 .
- Nicol A , Beavan J 2003 . Shortening of an overriding plate and its implications for slip on a subduction thrust, central Hikurangi margin, New Zealand . Tectonics 22 ( 6 ): 1070 , doi: doi:10.1029/2003TC001521 .
- Ota , Y , Berryman , KR , Hull , AG , Miyauchi , T and Iso , N . 1988 . Age and height distribution of Holocene transgressive deposits in eastern North Island, New Zealand. Palaeogeography, Palaeoclimatology . Palaeoecology , 68 : 135 – 151 .
- Ota Y , Berryman K , Fellows D , Hull A , Ishibashi K , Iso N , Miyauchi T , Miyoshi M , Yamasina K 1990 . Sections and profiles for the study of Holocene coastal tectonics, Gisborne–Cape Palliser, North Island, New Zealand . New Zealand Geologocal Survey Record 42, DSIR Geology and Geophysics, Lower Hutt, New Zealand .
- Ota , Y , Brown , LJ , Berryman , KR , Fujimori , T , Miyauchi , T , Beu , AG , Kashima , K and Taguchi , K . 1995 . Vertical tectonic movement in northeastern Marlborough: stratigraphic, radiocarbon, and palaeoecological data from Holocene estuaries . New Zealand Journal of Geology and Geophysics , 38 : 269 – 282 .
- Palmer , A , Vucetich , CG , McGlone , MS and Harper , MA . 1989 . Late Glacial loess and early Last Glacial vegetation history of Wairarapa valley, New Zealand . New Zealand Journal of Geology and Geophysics , 32 : 499 – 513 .
- Paul , MA and Barras , BF . 1998 . A geotechnical correction for post–depositional sediment compression: examples from the Forth valley, Scotland . Journal of Quaternary Science , 13 ( 2 ) : 171 – 176 .
- Pillans , B and Huber , P . 1995 . Interpreting coseismic deformation using Holocene coastal deposits, Wellington, New Zealand . Quaternary International , 26 : 87 – 95 .
- Pondard N , Barnes PM 2010 . Structure and palaeoearthquake records of active submarine faults, Cook Strait, New Zealand: Implications for fault interactions, stress loading, and seismic hazard . J of Geophysical Research 115 ( B12 ): B12320 .
- Rajendran , K , Rajendran , C , Earnest , A , Ravi Prasad , GV , Dutta , K , Ray , DK and Anu , R . 2008 . Age estimates of coastal terraces in the Andaman and Nicobar Islands and their tectonic implications . Tectonophysics , 455 : 53 – 60 .
- Reyners , M . 1998 . Plate coupling and the hazard of large subduction thrust earthquakes at the Hikurangi subduction zone, New Zealand . New Zealand Journal of Geology and Geophysics , 41 : 343 – 354 .
- Rodgers D , Little T 2006 . World's largest coseismic strike-slip offset: The 1855 rupture of the Wairarapa Fault, New Zealand, and implications for displacement/length scaling of continental earthquakes . Journal of Geophysical Research 111 ( B1212408 ): doi: doi:10.1029/2005JB004065 .
- Sawai Y , Nasu H , Yasuda Y 2002 . Fluctuations in relative sea-level during the past 3000 yr in the Onnetoh estuary, Hokkaido, northern Japan . Journal of Quaternary Science 17 ( 5–6 ): doi: doi:10.1002/jqs.708 .
- Schofield , JC . 1960 . Sea level fluctuations during the last 4,000 years as recorded by a chenier plain, Firth of Thames, New Zealand . New Zealand Journal of Geology and Geophysics , 3 : 467 – 485 .
- Singh L 1997 . Late Quaternary ages from terrestrial deposits, Marlborough Sounds, New Zealand; implications for dating the subsidence . Geological Society of New Zealand Miscellaneous Publication 95A : 149 .
- Singh L 1998 . Submerged terraces of Marlborough Sounds . Geological Society of New Zealand Miscellaneous Publication 101A : 206 .
- Sloss , CR , Murray–Wallace , CV and Jones , BG . 2007 . Holocene sea-level change on the southeast coast of Australia: a review . The Holocene , 17 ( 7 ) : 999 – 1014 .
- Stirling , M . 1992 . Late Holocene beach ridges displaced by the Wellington Fault in the Lower Hutt area, New Zealand . New Zealand Journal of Geology and Geophysics , 35 : 447 – 453 .
- Switzer , AD , Sloss , CR , Jones , BG and Bristow , CS . 2010 . Geomorphic evidence for mid–late Holocene higher sea level from southeastern Australia . Quaternary International , 221 : 13 – 22 .
- Wallace L , Pondard N , Barnes P , Beavan J , Van Dissen R , Litchfield N , Lamarche G , Little T 2008 . Kinematic model of the transition from subduction to strike-slip using GPS and active fault data . Geological Society of New Zealand Miscellaneous Publication 124A : 47 .
- Wallace L , Reyners M , Cochran U , Bannister S , Barnes P , Berryman K , Downes G , Eberhart–Philips D , Fagereng A , Ellis S and others 2009 . Characterizing the seismogenic zone of a major plate boundary subduction thrust: the Hikurangi Margin, New Zealand . Geochemistry Geophysics Geosystems 10 ( 10 ): doi: doi:10.1029/2009GC002610
- Wallace LM , Beavan J , McCaffrey R , Darby D 2004 . Subduction zone coupling and tectonic block rotations in the North Island, New Zealand . Journal of Geophysical Research 109 ( B12406 ): doi: doi:10.1029/2004JB003241 .
- Witkowski A , Lange–Bertalot H , Metzeltin D 2000 . Diatom Flora of Marine Coasts I. Iconographia Diatomologica Vol. 7. Liechtenstein, A.R.G. Gantner Verlag K.G., Ruggell .
- Woolfe , KJ . 1993 . Lakes Onoke and Wairarapa as modern analogues for the Hautotara and Te Muna Formations (Mid-Pleistocene), southern Wairarapa, New Zealand . Sedimentary Geology , 84 : 123 – 137 .
- Zachariasen , J , Sieh , K , Taylor , FW , Edwards , RL and Hantoro , WS . 1999 . Submergence and uplift associated with the giant 1833 Sumatran subduction zone earthquake: evidence from coral microatolls . Journal of Geophysical Research , 104 ( B1 ) : 895 – 919 .
- Zachariasen , J , Berryman , K , Langridge , R , Prentice , C , Rymer , M , Stirling , M and Villamor , P . 2006 . Timing of late Holocene surface rupture of the Wairau Fault, Marlborough, New Zealand . Journal of Geology and Geophysics , 49 : 159 – 174 .
Appendix 1
Dislocation modelling showing the coseismic vertical deformation (in metres) predicted for a subduction interface rupture event along the southern Hikurangi margin. This model is based upon current GPS-derived plate motion deficits on the plate interface and models a 500-year recurrence interval. If the recurrence of such events was longer (e.g. 1000 years), then the vertical displacements would be proportionally greater. Two models are depicted in Figure A1.1 due to the uncertainty in the degree of coupling in the offshore region beneath Cook Strait and offshore of the Wellington region. Figure A1.1A is based on a coupling model from Wallace et al. (Citation2008) and Fig. A1.1B is based on a coupling model from Wallace et al. (2009). Both of the couplings behind these two vertical deformation models fit the onshore GPS velocities equally well. Each of these events would involve as much as 8–10 m of slip on the interface and would be of magnitude>Mw 8.3, depending on the total slip and area ruptured in the event.