Abstract
Laser ablation inductively coupled plasma mass spectrometry (LA-ICP-MS) U–Pb zircon dating and whole-rock geochemistry of plutonic rocks from coastal exposures of the Paparoa Metamorphic Core Complex (PMCC) lower-plate has revealed their suite affinity and magmatic and metamorphic ages. Emplacement and metamorphism of the Charleston Orthogneiss occurred at 118±2 Ma and 107±2 Ma, respectively. Metamorphism of the Charleston Orthogneiss was coeval with Buckland Granite intrusion, suggesting a link between the two. Field relations between the post-metamorphic Doctor Bay dike (DBD) and Charleston Orthogneiss indicate intrusion of the dike at 105±2 Ma was syn-tectonic. Deformation of the Charleston Orthogneiss occurred during mid-crustal continental extension and PMCC formation in the New Zealand region of the Pacific Gondwana margin. Fabric development in the Charleston Orthogneiss may therefore be related to mid-crustal deformation associated with extension. The Charleston Orthogneiss and DBD are assigned to the I-/S-type Rahu Suite on the basis of their geochemistry, mid-Cretaceous ages, inherited zircon populations and inboard location.
Introduction
Extensive outcrops of the Paparoa Metamorphic Core Complex (PMCC) lower-plate are exposed along the coast between Charleston and Belfast Creek, Buller, New Zealand (Kimbrough & Tulloch Citation1989; Nathan Citation1975; Tulloch & Kimbrough Citation1989; White Citation1994). The PMCC formed during mid-Cretaceous continental extension in the New Zealand segment of Gondwana, which preceded fragmentation of the supercontinent (Tulloch & Kimbrough Citation1989; Spell et al. Citation2000). A small granitic pluton and numerous dikes cut the PMCC lower-plate gneisses in the Charleston area (Nathan Citation1975; Kimbrough & Tulloch Citation1989; White Citation1994). The timing of magmatism, metamorphism and fabric development in the footwall gneisses of the PMCC in relation to the continental extension that occurred immediately prior to separation of New Zealand from Gondwana is poorly constrained. This study reports on zircon geochronological and whole-rock geochemical investigations of granitoid orthogneisses from the lower-plate in order to provide constraints on the timing of their emplacement, metamorphism and deformation and their affiliation with previously described Cretaceous plutonic suites in New Zealand (Tulloch Citation1983, Citation1988; Tulloch & Kimbrough Citation2003).
Geological background
New Zealand basement geology
The geology of New Zealand is subdivided into pre-Cenozoic basement rocks and the unconformably overlying Late Cretaceous–Cenozoic sedimentary plus volcanic rocks (Mortimer Citation2004; Wandres & Bradshaw Citation2005). The basement rocks of New Zealand are further subdivided into the Western and Eastern Provinces, which contain a number of tectonostratigraphic terranes, and the Median Batholith (Mortimer Citation2004; Wandres & Bradshaw Citation2005). The Cenozoic Alpine Fault, which is the on-land expression of the Pacific–Australian plate boundary, has dextrally offset the basement rocks by c. 480 km (Cooper et al. Citation1987).
Western Province
The Western Province contains the Takaka and Buller Terranes, which together formed the Pacific margin of Gondwana from the Devonian onwards (Cooper Citation1989; Cooper & Tulloch Citation1992; Wandres & Bradshaw Citation2005; Mortimer Citation2004; Tulloch et al. Citation2009a). The Takaka Terrane is structurally complex, comprising numerous fault-bound slices of rock which have experienced multiple episodes of metamorphism and deformation of varying degrees. It contains a wide variety of rock types and is dominated by Middle–Late Cambrian intra-oceanic arc volcanics and interbedded arc-derived sediments deposited in a back-arc basin (Mortimer Citation2004; Wandres & Bradshaw Citation2005). In contrast, the adjacent Buller Terrane (the most inboard of the New Zealand terranes) consists largely of Ordovician quartzose greywacke turbidites of continental Gondwana provenance (Greenland Group) and pelagic argillite deposited in continental margin submarine fans, affected by Late Ordovician lower greenschist facies metamorphism and folded into upright folds with axial planar cleavage (Cooper Citation1974, Citation1989; Adams et al. Citation1975; Roser & Korsch Citation1988; Cooper & Tulloch Citation1992; Ireland Citation1992; Roser et al. Citation1996; Adams Citation2004).
Median Batholith
Subduction along the Pacific margin of Gondwana from the Devonian through the Cretaceous produced the Median Batholith, some of which occupies a position between the Western and Eastern Provinces, and includes contiguous plutons which intrude both provinces (Allibone et al. Citation2009a). Plutons that make up the Median Batholith and intrude the Western Province are subdivided on the basis of their age and composition into nine suites: the Karamea, Paringa, Tobin, Ridge, Foulwind, Darran, Western Fiordland Orthogneiss (WFO), Separation Point (SPS) and Rahu Suites (Tulloch Citation1983, Citation1988; Cooper & Tulloch Citation1992; Tulloch & Kimbrough Citation2003; Allibone et al. Citation2009a, Citationb; Tulloch et al. Citation2009a). Key characteristics of the relevant suites are summarised in .
Table 1 Key characteristics of relevant granitoid suites (Kimbrough et al. Citation1994; Muir et al. 1994, 1995, 1997, Citation1998; Waight et al. 1997, 1998; Ireland & Gibson 1998; Tulloch & Kimbrough 2003; Bolhar et al. Citation2008; Scott & Palin 2008). Bracketed ages indicate single plutons that are older or younger than most of those comprising a particular suite.
Eastern Province
The Eastern Province contains six variably metamorphosed Permian–Cretaceous volcanic-sedimentary terranes, which were accreted to the Pacific Gondwana margin due to transform and convergent tectonics (Mortimer Citation2004; Wandres & Bradshaw Citation2005). The latest stage of magmatism in the Median Batholith (SPS) ‘stitched’ the Takaka Terrane to the Eastern Province (Mortimer et al. Citation1999).
Paparoa Metamorphic Core Complex
The NE–SW trending Paparoa Range (A) is situated in the Buller Terrane. The lower-plate exposed in the southern and central portions of the Paparoa Range consists of Pecksniff Metasedimentary Gneiss, Okari Granite-Gneiss and Awakiri Tonalite Gneiss of the Charleston Metamorphic Group (CMG) (Nathan Citation1978; Tulloch & Kimbrough Citation1989; White Citation1994). Geothermobarometry indicates metamorphism of the gneisses occurred under amphibolite facies conditions of 600±50 °C and 4±1 kb (White Citation1994). Deformed Carboniferous (Windy Point and Foulwind Granites) and mid-Cretaceous Rahu Suite (Buckland Granite, Railway Quartz Diorite, Steele Granodiorite and Blackwater Granite) granitoid plutons comprise the northern PMCC lower-plate (Nathan Citation1975, Citation1978; Tulloch & Kimbrough Citation1989; Graham & White Citation1990; Muir et al. Citation1994; White Citation1994). The largest of the lower-plate plutons is the c. 180 km2 Buckland Granite, which is a leucocratic medium-grained weakly foliated biotite + muscovite±garnet monzogranite (Graham & White Citation1990; White Citation1994; Spell et al. Citation2000). Mid-Cretaceous crystallisation ages of 110±2 Ma have been obtained via sensitive high-resolution ion microprobe (SHRIMP) U–Th–Pb dating of zircon and monazite from the Buckland Granite (Muir et al. Citation1994; Ireland & Gibson Citation1998). Xenoliths of Pecksniff Metasedimentary Gneiss and adjacent granitoids, chilled margins and cross-cutting relations indicate the Buckland Granite is also the youngest lower-plate pluton (Nathan Citation1978; Graham & White Citation1990). Comparison of 40Ar–39Ar cooling ages for upper-plate and lower-plate rocks indicate relatively rapid cooling of the middle crust during the interval 111–90 Ma, interpreted to reflect extensional exhumation (Spell et al. Citation2000). Syn-tectonic emplacement of the Buckland Granite is implied by the weak tectonic foliation, clasts in the Hawks Crag Breccia member of the Pororari Group and overlap between radiometric dates of intrusion and PMCC unroofing (Tulloch & Kimbrough Citation1989; Spell et al. Citation2000; Klepeis et al. Citation2007).
Figure 1 A, Simplified geological map of the Charleston area. B, Photo and digitised field sketch illustrating the intrusive relations between the Doctor Bay dike and Charleston Orthogneiss (this study; Nathan 1975; Kimbrough & Tulloch 1989; Nathan et al. 2002). The diagonal dashed lines on the digitised sketch (B) represent the foliation.
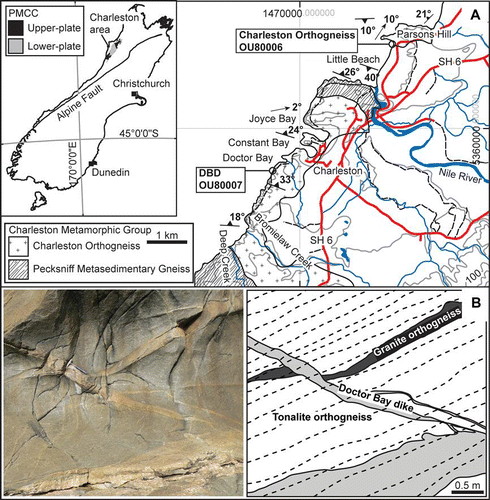
The Ohika and Pike Detachment Faults are oppositely dipping, low-angle (10–25° dip) normal faults displaying opposite senses of shear that separate the lower- and upper-plates in the northern and southern Paparoa Range, respectively (Tulloch & Kimbrough Citation1989; Tulloch & Palmer Citation1990). Shear zones accommodated extension in the middle crust beneath the detachment faults (Tulloch & Kimbrough Citation1989; Klepeis et al. Citation2007). Extension is thought to have first started in the lower crust (now exposed in Fiordland) at c. 114 Ma (Klepeis et al. Citation2007). Due to cooling and compositional contrasts the lower crust became strong relative to the mid-crust by c. 111 Ma. This led to partitioning of extension into narrow mid-crustal shear zones, some of which penetrated the lower crust to maintain kinematic compatibility between the deforming layers of crust (Klepeis et al. Citation2007). Motion along the Ohika and Pike Detachment Faults and their underlying shear zones accommodated mid-Cretaceous extensional exhumation of the PMCC mid-crustal lower-plate (Tulloch & Kimbrough Citation1989; Spell et al. Citation2000).
The Greenland Group and intruding granitic plutons (e.g. Berlins Porphyry and Punakaiki River pluton) constitute the upper-plate of the PMCC (Tulloch & Kimbrough Citation1989; Tulloch & Palmer Citation1990). Terrestrial Late Cretaceous (NZ Motuan stage, 100–103 Ma) Pororari Group sediments were derived from the PMCC upper- and lower-plates during unroofing of the metamorphic core. The Pororari Group unconformably overlies the basement units of both the upper- and lower-plates at the northern and southern extremities of the Paparoa Range (Nathan Citation1978; Raine Citation1984; Laird Citation1988; Tulloch & Palmer Citation1990; Muir et al. Citation1997; Tulloch et al. Citation2009b).
Charleston area
Two samples from coastal exposures of the PMCC lower-plate near Charleston are reported in this paper: (1) Charleston Orthogneiss granite (OU80006), and (2) a syn-tectonic granitic dike (OU80007) emplaced into the Charleston Orthogneiss near Doctor Bay. Location details of these samples are provided in .
Table 2 Details of the samples reported in this paper. Eastings and northings are in the NZTM coordinate system. Sample number prefix OU indicates the samples are held in the University of Otago, Department of Geology collection.
Charleston Orthogneiss
The Charleston Orthogneiss intruded the Devonian (Ireland & Gibson Citation1998) Pecksniff Metasedimentary Gneiss, and is made up of dark-grey plagioclase-porphyroblastic biotite tonalite which in turn has been intruded by two-mica granite. A gneissic foliation, defined by aligned micas, traverses intrusive contacts between the two orthogneisses, indicating that metamorphism and deformation occurred subsequent to emplacement of both lithologies (Fig. 1B; Kimbrough & Tulloch Citation1989). Along most of the Charleston coastal section, the complex, small-scale intrusive relation of granitic into tonalite orthogneisses prevents the distribution of these two rocks to be shown on maps at reasonable scales (1:10 000 or smaller). Hence, neither the tonalite nor granitic orthogneiss should be awarded formation status. However, exposures consisting of both orthogneiss lithologies are mappable; it is therefore suggested the tonalite and granitic orthogneisses in the Charleston area be collected under a formal formation name: the Charleston Orthogneiss.
An outcrop at Little Beach of coarse-grained, inequigranular, weakly foliated granitic orthogneiss was sampled for zircon geochronology and geochemistry (A). The texturally equilibrated mineral assemblage of this specimen consists of plagioclase+K-feldspar+quartz+biotite+ muscovite±garnet(spessartine–almandine)±sillimanite, which indicates the Charleston Orthogneiss underwent upper amphibolite facies metamorphism at temperatures ≥500 °C. Biotite displays dark-brown pleochroism, shows evidence of minor retrogressive alteration to chlorite and, along with muscovite, is euhedral–subhedral. Myrmekite is common, especially at the margins of K-feldspar grains, which are subhedral–anhedral. Plagioclase is subhedral–anhedral, multiple twins are often deformed and grains occasionally exhibit magmatic zoning. Quartz is anhedral and displays undulose extinction.
Doctor Bay dike (DBD)
In the sea cliffs south of Doctor Bay, a granitic dike intrudes the Charleston Orthogneiss oblique to the host rock foliation (A, 1B). This dike here is informally named the Doctor Bay dike (DBD) after the small keyhole-shaped bay to the north known as Doctor Bay (A). The DBD has a weak tectonic foliation defined by aligned micas and quartz and feldspar crystals, which parallels that in the Charleston Orthogneiss (B). The field relations suggest syn-tectonic emplacement of the DBD into the Charleston Orthogneiss.
The DBD is an inequigranular, coarse-grained muscovite + biotite granite rooted in a sub-horizontal coarse-grained–pegmatitic two-mica granite parent dike (B). Metamorphic mineralogy developed in the Charleston Orthogneiss, such as garnet and sillimanite, are absent. The entire assemblage is therefore considered to be magmatic; the DBD is post-metamorphic. Biotite exhibits dark-brown pleochroism, is euhedral–subhedral and minor retrogression to chlorite is evident. Myrmekite is ubiquitous at the margins of K-feldspar, which along with plagioclase is subhedral–anhedral and forms the majority of the groundmass. Quartz is anhedral and displays undulose extinction. Magmatic muscovite (euhedral) indicates the DBD was emplaced at ≥ 3–4 kb (Speer Citation1984; Anderson Citation1996), consistent with metamorphic mineral assemblages of the Charleston Orthogneiss (described above; Kimbrough & Tulloch Citation1989) and geothermobarometry of the CMG in the Paparoa Range (White Citation1994).
U–Th–Pb zircon data and cathodoluminescence
Zircon was extracted by standard crushing and heavy liquid methods. Grains were hand-picked and mounted in epoxy resin, analysed under cathodoluminescence using the JEOL JXA-6800 Superprobe at the Department of Geology, University of Otago, and dated by laser ablation inductively coupled plasma mass spectrometry (LA-ICP-MS) at the Research School of Earth Sciences (RSES), Australian National University (ANU) following the methods described in Scott & Palin (Citation2008). TEMORA-2 zircon and NIST SRM 610 glass were used as reference standards (Pearce et al. Citation1997; Black et al. Citation2004). Processing of raw data was achieved with a series of offline Excel workbooks. See Appendix 1 for a comprehensive description of the methodology.
Charleston Orthogneiss
Cathodoluminescence (CL) images of Charleston Orthogneiss zircon crystals exhibit a wide range of morphologies including rounded-anhedral pale cores mantled by thin irregular overgrowths, subhedral stubby prismatic pale cores with dull overgrowths exhibiting weak oscillatory zoning and euhedral grains with well-developed terminations and oscillatory zoning (A). Cores yielded ages ranging from Neoproterozoic to Early Cretaceous, although the majority record Devonian–Carboniferous dates (A). Mid-Cretaceous ages are restricted to dull rims and oscillatory zoned sectors (A).
Figure 2 Charleston Orthogneiss granite (OU80006) zircons. A, CL images. B, C, Probability density plots. D, E, Tera-Wasserburg concordia age diagrams of 238U/206Pb* (* indicates correction for common Pb). The laser pits visible in the CL image are located by the white and black circles (I & G '98: Ireland & Gibson 1998).
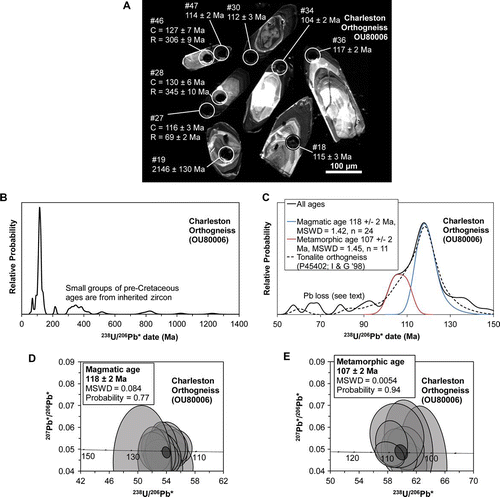
Forty-five of 71 analyses of Charleston Orthogneiss zircons yielded Cretaceous 238U/206Pb* ages (where * indicates correction for common-Pb, see Appendix 1; ). Distinguishable clusters of ages fall at c. 200 Ma, 300–400 Ma, 500–600 Ma, 700–900 Ma, 1000–1200 Ma and c. 2000 Ma (B; ). On a probability density plot, the Cretaceous dates comprise two prominent peaks centred at 118 Ma (major peak) and 107 Ma (lesser peak) and a series of minor peaks between c. 90 Ma and c. 50 Ma (C). The analyses comprising the major and lesser Cretaceous peaks constitute two normally distributed populations, with identical Tera-Wasserburg concordia and error-weighted mean ages of 118±2 Ma (mean squared weighted deviates [MSWD]=1.42, n = 24) and 107±2 Ma (MSWD = 1.45, n = 11), respectively (C–E).
Table 3 U-Th-Pb isotope data for Charleston Orthogneiss (granite) zircon analyses. Pb*: radiogenic component only; italicised dates are those pooled for the metamorphic age; bold italicised dates are those giving the emplacement age.
The two mid-Cretaceous populations of ages from Charleston Orthogneiss zircons are not distinguishable by their Th/U ratios (A). However, some dates contained in the major population are associated with higher Th/U values relative to those comprising the lesser population (A). Zones with exceptionally high U concentrations (>3000 ppm) yielded the youngest (<90 Ma) dates (B).
Doctor Bay dike
Most DBD zircons are stubby prismatic subhedral–euhedral grains with pale un-zoned cores enveloped by overgrowths with well-developed oscillatory zoning (A). However, some grains lack cores while others are anhedral (A). Late Cretaceous ages were obtained from oscillatory zoned overgrowths, dull rims and grains that lacked inherited cores (Fig. 4A).
Figure 4 Doctor Bay dike (OU80007) zircons. A, CL images. B, D, Probability density plots. C, Tera-Wasserburg concordia age diagram (* indicates correction for common Pb). The laser pits visible in the CL image are located by the white and black circles.
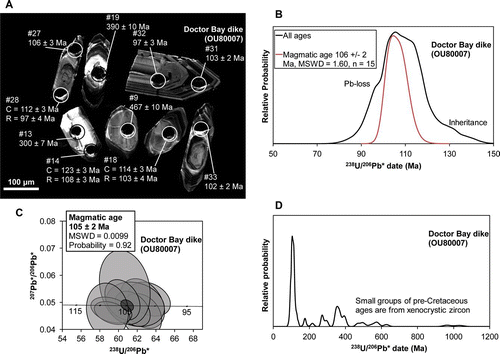
Thirty-three of the 64 238U/206Pb* ages obtained from DBD zircons comprise a slightly left-skewed mid-Cretaceous distribution, with a peak at c. 105 Ma (B; ). Of these, 15 form an approximately normally distributed population with an error-weighted mean age of 106±2 Ma (MSWD = 1.60) (B). Concordant analyses within this population yield a Tera-Wasserburg concordia age of 105±2 Ma (C). The older dates in the mid-Cretaceous population were excluded in this age determination (B) on the basis they are probably from zircon inherited from the Charleston Orthogneiss, while Pb-loss likely accounts for the few younger dates. The remaining ages are distributed between smaller Jurassic, Permotriassic, Devonian–Carboniferous, Cambrian–Ordovician and Proterozoic groupings (D; ). Cretaceous ages show a range in Th/U ratios from c. 0.85–0.02, but most are less than the ‘typical’ magmatic value of 0.5 (A) (Hoskin & Schaltegger Citation2003). There is also a large scatter in U concentrations from c. 250–4200 ppm (B).
Figure 5 Atomic Th/U and present-day U content against 238U/206Pb* age of DBD (OU80007) zircon. A, Atomic Th/U. B, present-day U. There is a large range in both Th/U ratios and U content (* indicates correction for common Pb).
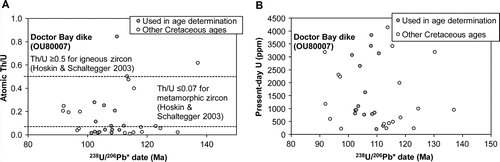
Table 4 U-Th-Pb isotope data for DBD zircon analyses. Pb*: radiogenic component only; bold italicised dates are those considered for the age.
Timing of magmatism, metamorphism and extension
Charleston Orthogneiss
The probability density plot of ages from the Charleston Orthogneiss granite, particularly the Cretaceous portion, bears a striking resemblance to that of SHRIMP U–Pb zircon ages from the tonalite member of the Charleston Orthogneiss (C) (fig. 7 of Ireland & Gibson Citation1998). Most importantly, the mid-Cretaceous ages on both these plots exhibit two populations with pooled mean ages of c. 120 Ma and c. 110 Ma (C), previously interpreted to record Separation Point Suite granitoid protolith emplacement and metamorphism coeval with syn-tectonic intrusion of the Buckland Granite (110±2 Ma), respectively (Ireland & Gibson Citation1998). Furthermore, the ages of Charleston Orthogneiss intrusion (119±2 Ma) and metamorphism (109±5 Ma) of Ireland and Gibson (Citation1998) are the same within error as those presented here (118±2 Ma and 107±2 Ma). Due to this similarity, Ireland and Gibson's (1998) interpretation of the major and less Cretaceous peaks on the probability density plot (C) representing emplacement and metamorphism of the Charleston Orthogneiss, respectively, is retained here. Conventional multi-grain thermal ionisation mass spectrometry (TIMS) U–Pb zircon dating of the Charleston Orthogneiss yielded discordant data with a lower intercept of 114±18 Ma interpreted as the magmatic crystallisation age (Kimbrough & Tulloch Citation1989), which also encompasses the ages reported here. The timing of metamorphism is further supported by SHRIMP U–Pb (103–113 Ma) and Th–Pb (105–114 Ma) monazite ages from the Charleston Orthogneiss tonalite (Ireland & Gibson Citation1998; Hiess et al. Citation2010), which are the same within error as our age of metamorphism (105–109 Ma) (C–2E). Overlap of the metamorphic age of the Charleston Orthogneiss and the age of Buckland Granite suggests heat from intrusion of the latter was responsible for metamorphism of the Charleston Orthogneiss.
Ages obtained from dull, featureless rims characteristic of metamorphic zircon comprise the lesser Cretaceous population, proposed to record metamorphism, while ages obtained from oscillatory zoned sectors (typical of igneous zircon; Corfu et al. Citation2003) make up the major Cretaceous population, considered to record emplacement of the Charleston Orthogneiss granite (A). Thus, cathodoluminescence of the Charleston Orthogneiss granite zircons also supports the interpretation that they record ages of both intrusion and metamorphism.
Metamorphic zircon often possesses low Th/U values relative to igneous zircon (Hoskin & Schaltegger Citation2003). The two Cretaceous populations of dates cannot be distinguished by their Th/U ratios alone, although the older population possesses a higher average Th content (Figs. 3A, 3C). SHRIMP analyses of zircon from the tonalite member of the Charleston Orthogneiss also reveal Cretaceous populations cannot be identified solely from their Th/U ratios (Ireland & Gibson Citation1998). However, the composition of metamorphic zircon is determined by whether it forms via re-equilibration of pre-existing zircon (closed or partially closed system behaviour) or crystallisation or precipitation of new zircon overgrowths (open system behaviour; Geisler et al. Citation2007; Martin et al. Citation2008). Metamorphic zircon formed by re-equilibration of pre-existing zircon retains textural and geochemical characteristics of the parent zircon (Martin et al. Citation2008). Conversely, new zircon crystallised in an open system does not preserve textural or geochemical characteristics of its precursor, but the composition of the rims is controlled by that of the fluid phase/melt present and surrounding minerals (Martin et al. Citation2008). The Th/U ratios of zircon therefore do not conclusively elucidate its origin. Hence, despite not possessing uniformly low Th/U values (A), a metamorphic origin for zircon rims from the Charleston Orthogneiss granite cannot be ruled out.
The high U content of the youngest dates yielded by Charleston Orthogneiss zircons (Fig. 3B) opens the possibility that they may not record metamorphic or igneous events; rather, they are the result of Pb loss from Cretaceous grains facilitated by radiation damage of the crystal lattice (Palenik et al. Citation2003). Alternatively, Pb loss may have been aided by crystal-plastic deformation of zircon, which has been suggested to create high diffusivity pathways for migration of elements through the zircon lattice (Reddy et al. Citation2006). Regardless of the mechanism, the progressive trailing of young dates from the Cretaceous populations (Fig. 2C) supports Pb loss as the cause. Groups of ages falling at c. 200 Ma, 400–300 Ma, 600–500 Ma, 900–700 Ma, 1000–1200 Ma and c. 2000 Ma (B) are interpreted to be from xenocrystic zircon.
Doctor Bay dike
The ages of magmatic zircon populations are thought to be characteristically normally distributed, with MSWD values approaching 1 (Bryan et al. Citation2004). An intrusive age of 106±2 Ma (MSWD = 1.60) for the DBD (B) is justified for this reason. Younger ages were excluded on the basis they are the result of Pb loss. Despite mostly showing Th/U ratios lower than typical magmatic zircon (A) (Hoskin & Schaltegger Citation2003), the DBD zircon ages used in the age determination are considered to be magmatic in origin. This is because the composition of zircon is dependent on that of melt from which it crystallised (Geisler et al. Citation2007; Martin et al. Citation2008), as outlined in more detail for the Charleston Orthogneiss above. Small clusters of Jurassic, Permo-Triassic, Devonian–Carboniferous, Cambrian–Ordovician and Proterozoic ages are interpreted as inherited (D). However, the slightly younger Tera-Wasserburg concordia age of 105±2 Ma (C) is preferred because only grains that are concordant and mutually overlapping within error are considered.
This age is the same within error as that of an undeformed muscovite pegmatite from the nearby Nile River dated at 108±3 Ma by Rb–Sr (recalculated from Aronson Citation1965), but marginally younger than a syn-tectonic granitic dike intruding biotite schist of the CMG in the central Paparoa Range that was dated at 113±4 Ma via the LA-ICP-MS U-Pb zircon method (Klepeis et al. Citation2007); this suggests lower-plate deformation was diachronous. Given the intrusive relations between the DBD and Charleston Orthogneiss (B), the age of the dike is also consistent with the newly reported and existing U–Pb zircon and monazite dating of the host rock (see above; Kimbrough & Tulloch Citation1989; Ireland & Gibson Citation1998).
Due to its syn-tectonic relations with the host rock and lack of metamorphic mineralogy, the age of the DBD constrains the timing of deformation of the Charleston Orthogneiss to 105±2 Ma. Although this age is indistinguishable from the metamorphic age of the Charleston Orthogneiss (107±2 Ma), the lack of metamorphic minerals in the DBD implies that fabric development post-dated metamorphism of the Charleston Orthogneiss by up to 4 Ma. The Charleston Orthogneiss was therefore metamorphosed at c. 107 Ma, and deformed and intruded by the DBD at c. 105 Ma.
Possible link between Charleston Orthogneiss deformation and continental extension
Syn-tectonic emplacement of the Buckland Granite occurred at 110±2 Ma, which may have facilitated the onset of extensional exhumation of the PMCC lower-plate (Tulloch & Kimbrough Citation1989; Muir et al. Citation1994; Ireland & Gibson Citation1998; Klepeis et al. Citation2007). Whole-rock, feldspar and muscovite Rb–Sr dating of ultramylonite from the Paparoa Shear Zone (PSZ) at White Horse Creek define an isochron age of 116±6 Ma, which is interpreted to date early-stage ductile deformation associated with continental extension and development of the PMCC (Ring et al. Citation2006). 40Ar–39Ar, K–Ar and Rb–Sr dating of the PMCC indicates extensional exhumation of the middle crust in the interval 111–90 Ma (Kimbrough & Tulloch Citation1989; Spell et al. Citation2000). Continental extension was therefore underway by 122–111 Ma. Development of the PMCC between 111 Ma and 90 Ma during continental extension, which may have started as early as 122 Ma, was therefore coeval with deformation of the Charleston Orthogneiss at c. 105 Ma.
Lineation measurements taken on Charleston Orthogneiss foliation surfaces trend c. NE–SE and dip at shallow angles to the north (A). These are sub-parallel to those from the PSZ, which accommodated most of the extension in the middle crust (Tulloch & Kimbrough Citation1989; Tulloch & Palmer Citation1990; White Citation1994; Spell et al. Citation2000; Klepeis et al. Citation2007). It is therefore suggested that fabric development in the Charleston Orthogneiss at c. 105 Ma was related to mid-crustal deformation associated with extension that led to unroofing of the PMCC lower-plate.
Whole-rock geochemistry and granitoid suite affiliation
Previously, the Charleston Orthogneiss has been correlated with either the Cretaceous Rahu or Separation Point Suites on the basis of geochemistry (Kimbrough & Tulloch Citation1989) and on the basis of emplacement age alone with the SPS (Ireland & Gibson Citation1998). More recently, the Charleston Orthogneiss has been mapped as Rahu Suite at the regional scale (Nathan et al. Citation2002).
Like the Rahu Suite (Tulloch Citation1983; Graham & White Citation1990; Muir et al. Citation1997; Waight et al. Citation1998; Tulloch & Kimbrough Citation2003), the Charleston Orthogneiss granite and DBD contain low Na2O and Al2O3 and high SiO2 and K2O (intermediate between I- and S-type granitoid compositions; Chappell & White Citation1992) relative to the Separation Point Suite (; A, 6B). The peraluminous composition (alumina saturation index or ASI >1.0) of the Charleston Orthogneiss granite and DBD is also typical of the Rahu Suite (; ) (Tulloch Citation1983; Waight et al. Citation1998). The moderate-SY (Sr/Y ratio) character of the two Cretaceous rocks also typifies the Rahu Suite; the Separation Point Suite is distinctly high Sr/Y (HiSY) (; C) (Muir et al. Citation1995; Tulloch & Kimbrough Citation2003). Furthermore, the rare earth element (REE) patterns of the Charleston Orthogneiss granite and DBD exhibit Eu anomalies and heavy rare earth elements (HREE) abundances akin to the Rahu Suite; the Separation Point Suite is relatively depleted in HREE and lacks Eu anomalies (; D) (Muir et al. Citation1995).
Figure 6 Whole-rock Harker and REE diagrams for the Charleston Orthogneiss (OU80006) and DBD (OU80007) compared to Cretaceous granitoids west of the Alpine Fault. A, Whole-rock Al2O3-SiO2. B, Whole-rock Na2O-K2O. C, Whole-rock Sr/Y-Y. D, REE diagram (K & T '89: Kimbrough & Tulloch 1989). (Data sources: White Citation1987; Kimbrough & Tulloch 1989; Graham & White 1990; Muir et al. 1995, 1997; Waight et al. 1998; Tulloch & Kimbrough 2003).
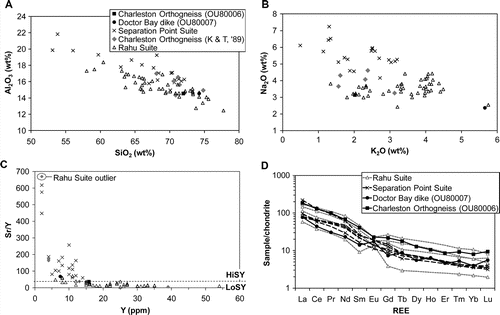
Table 5 Typical major (wt%) and trace (ppm) element composition of the Charleston Orthogneiss (granite) at Little Beach (OU80006) and DBD (OU80007) (ND: not determined). Subscript average indicates the value given is an average of multiple isotopes of that species.
Additionally, the presence of inherited zircon, their mid-Cretaceous ages (C–2E, 4B, 4C) and inboard location are typical of the Rahu Suite (Tulloch Citation1983; Muir et al. Citation1994, Citation1995, Citation1997; Waight et al. Citation1997, Citation1998; Tulloch & Kimbrough Citation2003). In combination, therefore, their geochemistry, zircon inheritance, age and inboard location indicate the Charleston Orthogneiss and DBD are of Rahu Suite affinity.
Conclusion
• | Intrusion and amphibolite facies metamorphism (≥ 500 °C) of the granitic Charleston Orthogneiss protolith occurred at 118±2 Ma and 107±2 Ma, respectively (C–2E). Metamorphism of the Charleston Orthogneiss occurred at the same time within error as intrusion of the Buckland Granite, which suggests they may be related. | ||||
• | Emplacement of the post-metamorphic, syn-tectonic Doctor Bay dike constrains deformation of the Charleston Orthogneiss (the host rock) to 105±2 Ma (C). | ||||
• | Fabric development (105±2 Ma) in the Charleston Orthogneiss was related to deformation of the middle crust associated with extension in the New Zealand portion of Gondwana. | ||||
• | In combination, the whole-rock major and trace element geochemistry (), age, presence of inherited zircon (C–2E, 4B, 4C) and inboard location of the Charleston Orthogneiss granite and DBD suggest correlation with the Rahu Suite, rather than the Separation Point Suite. |
Acknowledgements
The majority of original content presented here formed the honours dissertation of MWS written in 2008. Brent Pooley, Kat Lilly, Damian Walls, Charlotte Allen, Katherine Cambridge and Dushan Jugum are thanked for their help with sample preparation and analyses. Liam Heath and David Sagar accompanied MWS during reconnaissance fieldwork, for which the author is very grateful. Trevor Ireland generously provided raw U–Pb–Th zircon data from the Charleston Orthogneiss. Gratitude is also extended to Andy Tulloch for insightful discussions during this project. James Scott critically reviewed the manuscript on several occasions, which greatly improved the document. The comments and suggestions of reviewers Uwe Ring and Joseph Kula and editor Nick Mortimer were most helpful in improving the final manuscript. The Department of Geology Benson Fund provided MWS with financial aid for fieldwork. A University of Otago Research Grant to JMP supported LA-ICP-MS U–Pb zircon dating at the Research School of Earth Sciences (RSES), Australian National University (ANU), Canberra.
References
- Adams , C , Harper , C and Laird , M . 1975 . K-Ar ages of low-grade metasediments of the Greenland and Waiuta Groups in Westland and Buller, New Zealand . New Zealand Journal of Geology and Geophysics , 18 : 39 – 48 .
- Adams , C . 2004 . Rb-Sr age and strontium isotope characteristics of the Greenland Group, Buller Terrane, New Zealand, and correlations at the East Gondwana margin . New Zealand Journal of Geology and Geophysics , 47 : 189 – 200 .
- Allibone , A , Jongens , R , Scott , J , Tulloch , A , Turnbull , I , Cooper , A , Powell , N , Ladley , E , King , R and Rattenbury , M . 2009a . Plutonic rocks of the Median Batholith in eastern and central Fiordland, New Zealand: field relations, geochemistry, correlation, and nomenclature . New Zealand Journal of Geology and Geophysics , 52 : 101 – 148 .
- Allibone , A , Jongens , R , Turnbull , I , Milan , I , Daczko , N , De Paulo , M and Tulloch , A . 2009b . Plutonic rocks of western Fiordland, New Zealand: field relations, geochemistry, correlation, and nomenclature . New Zealand Journal of Geology and Geophysics , 52 : 379 – 415 .
- Anderson , J . 1996 . Status of thermobarometry in granitic batholiths . Transactions of the Royal Society of Edinburgh: Earth Sciences , 87 : 125 – 138 .
- Aronson 1965 . Reconnaissance Rubidium-Strontium geochronology of New Zealand plutonic and metamorphic rocks . New Zealand Journal of Geology and Geophysics 8 : 401 – 423 .
- Black L , Kamo S , Allen C , Davis D , Aleinikoff J , Valley J , Mundil R , Campbell I , Korsch R , Williams I and others 2004 . Improved 206Pb/238U microprobe geochronology by the monitoring of a trace-element-related matrix effect; SHRIMP, ID–TIMS, ELA–ICP–MS and oxygen isotope documentation for a series of zircon standards . Chemical Geology 205 : 115 – 140 .
- Bolhar , R , Weaver , S , Palin , J , Cole , J and Paterson , L . 2008 . Systematics of zircon crystallisation in the Cretaceous Separation Point Suite, New Zealand, using U/Pb isotopes, REE and Ti geothermometry . Contributions to Mineralogy and Petrology , 156 : 133 – 160 .
- Bryan , S , Allen , C , Holcombe , R and Fielding , C . 2004 . U-Pb zircon geochronology of Late Devonian to Early Carboniferous extension-related silicic volcanism in the northern New England Fold Belt . Australian Journal of Earth Sciences , 51 : 645 – 664 .
- Chappell , B and White , A . 1992 . I- and S-type granites of the Lachlan Fold Belt . Transactions of the Royal Society of Edinburgh: Earth Sciences , 83 : 1–26
- Cooper , A , Barreiro , B , Kimbrough , D and Mattinson , J . 1987 . Lamprophyre dike intrusion and the age of the Alpine fault, New Zealand . Geology , 15 : 941 – 944 .
- Cooper , R . 1974 . Age of the Greenland and Waiuta Groups, South Island, New Zealand . New Zealand Journal of Geology and Geophysics , 17 : 955 – 962 .
- Cooper , R . 1989 . Early Paleozoic Terranes of New Zealand . Journal of the Royal Society of New Zealand , 19 : 73 – 112 .
- Cooper , R and Tulloch , A . 1992 . Early Paleozoic terranes in New Zealand and their relationship to the Lachlan Fold Belt . Tectonophysics , 214 : 129 – 144 .
- Corfu , F , Hanchar , J , Hoskin , P and Kinny , P . 2003 . Atlas of zircon textures . Reviews in Mineralogy and Geochemistry , 53 : 468 – 500 .
- Cumming , G and Richards , J . 1975 . Ore lead ratios in a continuously changing Earth . Earth and Planetary Science Letters , 28 : 155 – 171 .
- Geisler , T , Schaltegger , U and Tomaschek , F . 2007 . Re-equilibration of zircon in aqueous fluids and melts . Elements , 3 : 43 – 50 .
- Graham , I and White , P . 1990 . Rb-Sr dating of Rahu Suite granitoids from the Paparoa Range, North Westland, New Zealand . New Zealand Journal of Geology and Geophysics , 33 : 11 – 22 .
- Hiess , J , Ireland , T and Rattenbury , R . 2010 . U-Th-Pb zircon and monazite geochronology of Western Province gneissic rocks, central-south Westland, New Zealand . New Zealand Journal of Geology and Geophysics , 53 : 241 – 269 .
- Hoskin , P and Schaltegger , U . 2003 . The composition of zircon and igneous and metamorphic petrogenesis . Reviews in Mineralogy and Geochemistry , 53 : 27 – 62 .
- Ireland , T . 1992 . Crustal evolution of New Zealand: Evidence from age distributions of detrital zircons in Western Province paragneisses and Torlesse greywacke . Geochemica et Cosmochimica Acta , 56 : 911 – 920 .
- Ireland , T and Gibson , G . 1998 . SHRIMP monazite and zircon geochronology of high-grade metamorphism in New Zealand . Journal of Metamorphic Geology , 16 : 149 – 167 .
- Kimbrough , D and Tulloch , A . 1989 . Early Cretaceous age of orthogneiss from the Charleston Metamorphic Group, New Zealand . Earth and Planetary Science Letters , 95 : 130 – 140 .
- Kimbrough , D , Tulloch , A , Coombs , D , Landis , C , Johnston , M and Mattinson , J . 1994 . Uranium-lead zircon ages from the Median Tectonic Zone, New Zealand . New Zealand Journal of Geology and Geophysics , 37 : 393 – 419 .
- Klepeis K , King D , De Paoli M , Clarke G , Gehrels G 2007 . Interaction of strong lower and weak middle crust during lithospheric extension in western New Zealand . Tectonics 26 , 10.1029/2006TC002003: TC4017 .
- Laird , M . 1988 . Sheet S37 Punakaiki , Wellington, , New Zealand : DSIR Publishing .
- Martin , L , Duchene , S , Deloule , E and Vanderhaeghe , O . 2008 . Mobility of trace elements and oxygen in zircon during metamorphism: Consequences for geochemical tracing . Earth and Planetary Science Letters , 267 : 161 – 174 .
- Mortimer , N . 2004 . New Zealand's Geological Foundations . Gondwana Research , 7 : 261 – 272 .
- Mortimer , N , Tulloch , A , Spark , R , Walker , N , Ladley , E , Allibone , A and Kimbrough , D . 1999 . Overview of the Median Batholith, New Zealand: a new interpretation of the geology of the Median Tectonic Zone and adjacent rocks . Journal of African Earth Sciences , 29 : 257 – 268 .
- Muir , R , Ireland , T , Weaver , S and Bradshaw , J . 1994 . Ion microprobe U–Pb zircon geochronology of granitic magmatism in the Western Province of the South Island, New Zealand . Chemical Geology , 113 : 171 – 189 .
- Muir , R , Weaver , S , Bradshaw , J , Eby , G and Evans , J . 1995 . The Cretaceous Separation Point Batholith, New Zealand: granitoid magmas formed by melting of mafic lithosphere . Journal of the Geological Society of London , 152 : 689 – 701 .
- Muir , R , Ireland , T , Weaver , S , Bradshaw , J , Waight , T , Jongens , R and Eby , G . 1997 . SHRIMP U-Pb geochronology of Cretaceous magmatism in northwest Nelson–Westland, South Island, New Zealand . New Zealand Geology and Geophysics , 40 : 453 – 463 .
- Muir , R , Ireland , T , Weaver , S , Bradshaw , J , Evans , J , Eby , G and Shelley , D . 1998 . Geochronology and geochemistry of a Mesozoic magmatic arc system, Fiordland, New Zealand . Journal of the Geological Society of London , 155 : 1037 – 1053 .
- Nathan , S . 1975 . Sheets S23 & S30 Foulwind and Charleston , Wellington : NZ Department of Scientific and Industrial Research .
- Nathan , S . 1978 . Sheets S31 & part S32 Buller-Lyell , Wellington : Department of Scientific and Industrial Research .
- Nathan , S , Rattenbury , M and Suggate , R . 2002 . Geology of the Greymouth area. Institute of Geological & Nuclear Sciences 1:250,000 geological map 12 , Lower Hutt, , New Zealand : Institute of Geological & Nuclear Sciences Limited .
- Palenik , C , Nasdala , L and Ewing , R . 2003 . Radiation damage in zircon . American Mineralogist , 88 : 770 – 781 .
- Pearce , N , Perkins , W , Westgate , J , Gorton , M , Jackson , S , Neal , C and Chenery , S . 1997 . A compilation of new and published major and trace element data for NIST SRM 610 and NIST SRM 612 glass reference materials . Geostandards Newsletter , 21 : 115 – 144 .
- Raine J 1984 . Outline of palynological zonation of Cretaceous to Paleogene terrestrial sediments in West Coast region, South Island, New Zealand . New Zealand Geological Survey Report 109. Lower Hutt, New Zealand, Department of Scientific and Industrial Research . Pp. 82.
- Reddy , S , Timms , N , Trimby , P , Kinny , P , Buchan , C and Blake , K . 2006 . Crystal-plastic deformation of zircon: A defect in the assumption of chemical robustness . Geology , 34 : 257 – 260 .
- Ring U , Herd M , Bolhar R , Glodny J , Palin J 2006 . Dating mylonitisation in the Paparoa Core Complex, Westland, GSNZ Misc . Pub. 122A . 116 p.
- Roser , B and Korsch , R . 1988 . Provenance signatures of sandstone-mudstone suites determined using discriminant function analysis of major-element data . Chemical Geology , 67 : 119 – 139 .
- Roser , B , Cooper , R , Nathan , S and Tulloch , A . 1996 . Reconnaissance sandstone geochemistry, provenance, and tectonic setting of the lower Paleozoic terranes of the West Coast and Nelson, New Zealand . New Zealand Journal of Geology and Geophysics , 39 : 1 – 16 .
- Scott , J and Palin , J . 2008 . LA-ICP-MS U-Pb zircon ages from Mesozoic plutonic rocks in eastern Fiordland, New Zealand . New Zealand Journal of Geology and Geophysics , 51 : 105 – 113 .
- Speer , J . 1984 . Micas in igneous rocks . Reviews in Mineralogy , 13 : 299 – 356 .
- Spell , T , McDougall , I and Tulloch , A . 2000 . Thermochronologic constraints on the breakup of the Pacific Gondwana margin: The Paparoa metamorphic core complex, South Island, New Zealand . Tectonics , 19 : 433 – 451 .
- Tulloch , A . 1983 . Granitoid rocks of New Zealand – A brief review . Geological Society of America Memoir , 159 : 5 – 20 .
- Tulloch , A . 1988 . Batholiths, plutons, and suites: nomenclature for granitoid rocks of Westland–Nelson, New Zealand . New Zealand Journal of Geology and Geophysics , 31 : 505 – 509 .
- Tulloch , A and Kimbrough , D . 1989 . The Paparoa Metamorphic Core Complex, New Zealand: Cretaceous continental extension associated with fragmentation of the Pacific margin of Gondwana . Tectonics , 8 : 1217 – 1234 .
- Tulloch , A and Palmer , K . 1990 . Tectonic implications of granite cobbles from the mid-Cretaceous Pororari Group, southwest Nelson, New Zealand . New Zealand Journal of Geology and Geophysics , 33 : 205 – 217 .
- Tulloch A , Kimbrough D 2003 . Paired plutonic belts in convergent margins and the development of high Sr/Y magmatism: Peninsular Ranges batholith of Baja-California and Median batholith of New Zealand . In : Johnson S , Patterson S , Fletcher J , Girty G , Kimbrough D , Martín-Barajas A . Tectonic evolution of northwestern Mexico and the southwestern USA . Boulder, Colorado, Geological Society of America Special Paper . Pp. 275 – 295 .
- Tulloch , A , Ramezani , J , Kimbrough , D , Faure , K and Allibone , A . 2009a . U-Pb geochronology of mid-Paleozoic plutonism in western New Zealand: Implications for S-type granite generation and growth of the east Gondwana margin . Geological Society of America Bulletin , 121 : 1236 – 1261 .
- Tulloch , A , Ramezani , J , Mortimer , N , Mortensen , J , Van Den Bogaard , P and Maas , R . 2009b . Cretaceous felsic volcanism in New Zealand and Lord Howe Rise (Zealandia) as a precursor to final Gondwana break-up . The Geological Society of London Special Publication , 321 : 89 – 118 .
- Waight , T , Weaver , S , Ireland , T , Mass , R , Muir , R and Shelley , D . 1997 . Field characteristics, petrography, and geochronology of the Hohonu Batholith and the adjacent Granite Hill Complex, North Westland, New Zealand . New Zealand Journal of Geology and Geophysics , 40 : 1 – 17 .
- Waight , T , Weaver , S , Muir , J , Mass , R and Eby , G . 1998 . The Hohonu Batholith of North Westland, New Zealand: granitoid compositions controlled by source H2O contents and generated during tectonic transition . Contributions to Mineralogy and Petrology , 130 : 225 – 239 .
- Wandres A , Bradshaw J 2005 . New Zealand tectonostratigraphy and implications from conglomeratic rocks for the configuration of the SW Pacific margin of Gondwana . In : Vaughan A , Leat P , Pankhurst R Terrane Processes at the Margins of Gondwana . The Geological Society of London , London . Pp. 179 – 216 .
- White P 1987 . The petrology, geochemistry and petrogenesis of igneous and metamorphic rocks from the Paparoa Range, southwest Nelson . Unpublished PhD thesis, Victoria University of Wellington, Wellington, New Zealand . 115 p.
- White , P . 1994 . Thermobarometry of the Charleston Metamorphic Group and implications for the evolution of the Paparoa Metamorphic Core Complex, New Zealand . New Zealand Journal of Geology and Geophysics , 37 : 201 – 209 .
Appendix
LA-ICP-MS U-Pb Zircon Dating
Standard techniques of rock crushing (to 250 µm), plus heavy liquid (lithium-polytungstate or LST ρ = 2.8 g/cm3 & methylene-iodide or MEI ρ = 3.3 g/cm3) and magnetic separation (using the Frantz L-1 Isodynamic Separator at GNS Science, Dunedin) were employed to liberate and concentrate zircons. Subsequently, zircon separates were dry hand-picked using a probe and mounted on double-sided tape. These zircon mounts were encased in epoxy resin. The upper surface of the briquettes containing the zircon samples was first ground with fine (1000 µm) sandpaper to expose the grains, and then polished with an automated polisher using a combination of aluminium-oxide solution, diamond paste and diamond mats.
LA-ICP-MS U-Pb zircon dating was carried out at the Research School of Earth Sciences (RSES), Australian National University (ANU), Canberra. Samples were housed in a HelEx sample cell. Laser ablation was performed with a LambdaPhysik LaserTechnik LPX 120I UV ArF pulsed excimer laser operated at 23 kV producing a 70 mJ 5 Hz beam. Laser spot diameter remained constant throughout an analytical session at either 22 µm or 28 µm. An Agilent 7500 s ICP-MS received ablated matter directed through a flow homogeniser from the sample chamber with He-Ar gas. Abundances of 18 isotopes were recorded with varying integration times according to their importance in U-Pb dating and relative abundances in zircon: 40 ms for 206Pb, 207Pb and 208Pb; 25 ms for 232Th, 235U and 238U; 20 ms for 49Ti; 10 ms for 31P, 89Y, 139La, 140Ce, 147Sm, 153Eu 163Dy, 175Lu and 177Hf; 5 ms for 29Si and 91Zr giving ∼396 ms per analysis. Background levels of the selected isotopes were collected for 20 s immediately prior to each spot analysis, which lasted 40 s allowing ∼100 mass scans (40 s/0.396 s) and the laser to penetrate ∼20 µm.
The TEMORA 2 zircon (413 ± 3 Ma; Black et al. 2004) and NIST SRM 610 glass (Pearce et al. 1997) standards were analysed at the beginning and conclusion, plus at regular intervals throughout each analytical session. The sequence in which the samples were analysed remained constant within an analytical session. Thus, corrections made with reference to the standards are applicable to all analyses within an analytical session. If the laser bored through a grain into the underlying epoxy, as recognised via real-time monitoring of isotope readings, ablation was halted for the remainder of the spot analysis.
Raw data was processed using a series of offline Microsoft Office Excel spreadsheets. The first 10 s of all spot analyses were discarded, because following activation of the laser a number of seconds passed before the signal stabilised. In addition, background levels of the measured isotopes were deducted, and outlier data >3σ from mean values were excluded from all analyses. Instrument drift and penetration depth fractionation were corrected via reference to data collected for the standards averaged for each analytical session. Standard 207Pb/206Pb or 206Pb/238U ratios that differed by greater than ∼10% from mean values were excluded. Direct measurement of common Pb was not undertaken because 204Hg, which interferes with the measurement of stable 204Pb, is present in background concentrations well above those of non-radiogenic Pb in zircons. Consequently, a 208Pb-based common Pb correction was implemented, which estimated the amount of non-radiogenic Pb through comparison of expected and measured 208Pb/206Pb ratios according to actual 232Th/238U values (Cumming & Richards 1975).
Uncertainties implicit in individual spot analyses were calculated as the standard error of the mean (SEM) for the corrected U, Pb and Th isotopic ratios across the interval selected for dating. Comparison of these internal errors with those estimated via statistics for each mass scan allowed calculation of the mean squared weighted deviates (MSWD) values. When calculation of the MSWD for common Pb-corrected ages failed, a conservative value was assumed for subsequent processing. Selection of the appropriate interval of unknown zircon analyses was based on age zonation (see below), achieving MSWD values approaching one if reasonably possible, and the presence or absence of inclusions indicated by spikes in trace element content. Cathodoluminescence images of the analysed zircons, captured with the JEOL JXA-6800 Superprobe at the University of Otago, aided in recognition of age zones indicated by trends in Pb/U ratios and trace element abundances with penetration depth in spot analyses. The unique advantage of LA-ICP-MS U-Pb zircon dating over alternative dating methods is the depth profiling of individual grains; multiple growth zones may be analysed within a single grain with each spot analysis (e.g., Fig. A1). Total uncertainties in the ratios of U, Pb and Th for each spot analysis were calculated as a combination of their internal uncertainty and the standard deviation in the same ratios measured in TEMORA 2 for the analytical session.
All dates <1000 Ma were calculated using the ratio of radiogenic (*) 206Pb to 238U, because uncertainty in the averaged 207Pb/206Pb ratio of TEMORA 2 was relatively poor. Pooled ages are error-weighted averages, whose uncertainty represents two SEM, do not include dates greater than 10% discordant or with MSWD values >10, and exhibit Gaussian distributions on probability density plots (generated with IsoPlot 2 add-in for Excel), which was assumed indicative of a single natural zircon population (Bryan et al. 2004). Ages were also calculated using a Tera-Wasserburg (207Pb/206Pb-238U/206Pb) concordia generated with IsoPlot 3.
XRF Whole-rock Major Element Geochemistry
Representative sub-samples of each rock were placed in an oven maintained at a constant temperature of 105 °C overnight to remove any free H2O. Once dry, ∼0.64 g of each sub-sample was combined with ∼6.8 g of lithium borate flux and ∼1.0 g of NH4NO3 in a plastic jar of known weight. Utilising an Automated Fusion Technology Ltd. Phoenix 4000 unit, these cocktails were heated to 1100 °C in Pt-Au crucibles, mixed thoroughly once molten to ensure homogeneity, and cast into silicate glass discs of 40 mm diameter in pre-heated Pt-Au plates cooled by compressed oxygen. Discs of two sub-samples of each rock were prepared and analysed twice to assess the reproducibility of XRF whole-rock major element geochemistry results and compositional variation within each sample. Loss on ignition (LOI) values were not determined because the required furnace was nonoperational at the time of sample preparation. However, the total oxide weight percent for all analyses were within 1% of 100% indicating LOI was minimal, typical of granitic rocks (e.g., Kimbrough & Tulloch 1989). The Philips PW2400 sequential wavelength dispersive x-ray fluorescence spectrometer fitted with a 3 kW Rh tube, in the Department of Geology, University of Otago was employed to determine major element compositions.
LA-ICP-MS Whole-rock Trace Element Geochemistry
The same silicate glass discs prepared for XRF whole-rock geochemistry were analysed via LA-ICP-MS at the Otago Community Trust Centre for Trace Element Analysis, University of Otago, and RSES, ANU, Canberra. To reduce the duration of the analytical session, the need to exchange samples was eliminated by cutting small (∼0.5 cm long) sections from each disc, which were then mounted in a single epoxy resin briquette. The briquette was polished with a 1 µm diamond mat.
At the University of Otago, a NewWave 213 nm pulsed laser operated at a half-power and 10 Hz was used for laser ablation. Ablated material was transported by He gas from the sample cell, and mixed with gaseous Ar before being passed into an Agilent 7500 ICP-MS. Data for 45 mass peaks were collected in time-resolved mode with one point per peak. Integration times were 10 ms for all the isotopes analysed (24Mg, 27Al, 29Si, 43Ca, 39K, 43Ca, 45Sc, 47Ti, 48Ti, 49Ti, 51V, 52Cr, 53Cr, 57Fe, 69Co, 60Ni, 71Ga, 85Rb, 88Sr, 89Y, 90Zr, 93Nb, 133Cs, 137Ba, 139La, 140Ce, 141Pr, 146Nd, 147Sm, 153Eu, 157Gd, 159Tb, 163Dy, 165Ho, 166Er, 169Tm, 174Yb, 175Lu, 178Hf, 181Ta, 206Pb, 207Pb, 208Pb, 232Th, 238U), giving a total scan time of 539 ms. Background levels were collected for the first 20 s of each analysis prior to triggering of the laser, followed by 40 s of data acquisition for each sub-sample. The laser was set to migrate at 10 µm/s and a spot diameter of 100 µm. This gave ∼100 mass scans from a 400 µm long ∼5 µm deep track across each disc. The effects of depth fractionation are negligible when employing laser-migration, because laser penetration is relatively shallow. A stationary spot, of 78 µm diameter was utilised at the RSES. However, depth fractionation was minimal due to the relatively large spot diameter employed. At the beginning and conclusion of the analytical session, two analyses of NIST SRM 610 glass standard were taken. Between each spot analysis, a purge time ≥120 s was allowed for a return to background levels.
Reduction of the raw trace element data utilised an offline Excel spreadsheet. The raw mass peak data were corrected for background levels and mass bias drift with reference to the NIST SRM 610 silicate glass standard. Trace element concentrations (ppm) in the sub-samples were obtained by normalising the corrected count rates to the known Si and trace element content of the standard and Si concentration in the unknowns, which was determined previously as whole-rock SiO2 via XRF. The two NIST SRM 610 standard analyses directly bounding the analyses of the samples were used data for correction and conversion (i.e., the second and first NIST SRM 610 standard analyses taken at the beginning and end of the analytical session, respectively). The interval selection of data from the sub-samples excluded the first 10–15 s acquired following activation of the laser, because the signal only stabilised after this amount of time following the commencement of ablation.