Abstract
Melt rates on glaciers are strongly influenced by the presence of supraglacial debris, which can either enhance or reduce ablation relative to bare ice. Most recently, Franz Josef Glacier has entered into a phase of strong retreat and downwasting, with the increasing emergence of debris on the surface in the ablation zone. Previously at Franz Josef Glacier, melt has only been measured on bare ice. During February 2012, a network of 11 ablation stakes was drilled into locations of varying supraglacial debris thickness on the lower glacier. Mean ablation rates over 9 days varied over the range 1.2–10.1 cm d−1, and were closely related to debris thickness. Concomitant observations of air temperature allowed the application of a degree-day approach to the calculation of melt rates, with air temperature providing a strong indicator of melt. Degree-day factors (d f) varied over the range 1.1–8.1 mm d−1 °C−1 (mean of 4.4 mm d−1 °C−1), comparable with rates reported in other studies. Mapping of the current debris cover revealed 0.7 km2 of the 4.9 km2 ablation zone surface was debris-covered, with thicknesses ranging 1–50 cm. Based on measured debris thicknesses and d f, ablation on debris-covered areas of the glacier is reduced by a total of 41% which equates to a 6% reduction in melt overall across the entire ablation zone. This study highlights the usefulness of a short-term survey to gather representative ablation data, consistent with numerous overseas ablation studies on debris-covered glaciers.
Introduction
Glacier retreat in many regions is associated with the development of debris-covered ablation zones. Debris cover extent may range from completely debris-covered tongues (e.g. Miage Glacier, Italy; Smiraglia et al. Citation2000), to partly covered tongues (e.g. glaciers in Chenab basin, Himalaya; Shukla et al. Citation2010) and finally to discrete areas of fine dust and pebbles as observed on many alpine glaciers (Mayer et al. Citation2011). Based on several small-scale field studies since Østrem's (Citation1959) observations, it is well known that supraglacial debris cover modifies glacier behaviour, and this work has been complemented by theoretical studies based on heat flux calculations (e.g. Nakawo & Young Citation1981). Empirical relationships between supraglacial debris thickness and ice-melt rates were initially established by Østrem (Citation1959). Ablation under supraglacial debris is a balance between melt enhancement due to increased absorption of shortwave radiation, which dominates under thin debris covers, and melt reduction through insulation from atmospheric heat and insolation, which dominates under greater debris thicknesses (Nicholson & Benn Citation2006). Once a ‘critical’ thickness (debris thickness at which sub-debris ablation equals that under bare snow or ice) of debris is reached, the shielding effect dominates which insulates the underlying ice and reduces melt rates (Østrem Citation1959). This critical thickness depends on physical properties of the debris and on meteorological conditions, with values reported varying between 2 cm (Mattson Citation2000) and 7–8 cm (Popovnin & Rozova Citation2002). Ablation rates progressively decline under thicker debris covers, a pattern confirmed by several studies (e.g. Loomis Citation1970; Mattson et al. Citation1993; Nicholson & Benn Citation2006).
The degree to which debris alters ablation at any given glacier, including the critical thickness at which ablation is retarded, is however difficult to quantify under real conditions (Scherler et al. Citation2011). This is due to the typical highly heterogeneous nature of supraglacial debris cover on glaciers, local climatic conditions (which may vary with latitude and altitude or include local effects such as topographic shading etc.) and lithology (Reznichenko et al. Citation2010). Indeed, ice ablation is controlled by the amount of energy supplied to ice (Cuffey & Paterson Citation2010), which is influenced by the interplay between the surface energy balance and heat transfer processes through the debris layer. Reznichenko et al. (Citation2010) found that diurnal radiation cycles were critical in generating the insulating ability of debris cover, highlighting that such cycles vary with latitude and altitude. Furthermore, the degree of climate ‘continentality’ may also be an important effect on ice melt with continental climates characterised by less cloudiness and greater shortwave radiation receipts than maritime glaciers (Oerlemans Citation2001), and lower annual precipitation leading to drier, more thermally resistant debris covers.
While debris-covered glaciers are common in high-mountain environments such as the Himalaya, the Peruvian Andes and the European Alps, there have been very few studies of the effect of debris cover on ice surface ablation in the New Zealand Southern Alps to date (Brook & Paine Citation2012). Nevertheless, studies of the impact of debris cover on glacier ablation are important for two reasons.
First, if mass balance data are unavailable, glacier advances and retreats are typically used as indicators of their response to climate change (Hooker & Fitzharris Citation1999; Oerlemans Citation2005), even though terminus changes are not unambiguous indicators of glacier response to climate. Indeed, when supraglacial debris covers a significant proportion of the ablation zone, the glacier mass balance may become significantly less negative, influencing the behaviour of the terminus. This may, in turn, generate a terminal moraine that only has a partial climate-related cause (Reznichenko et al. Citation2011).
Second, of more immediate importance is the response of glaciers to future climate change since the current trend of glacier retreat has been widely accompanied by an increasing extent of debris cover on glacier tongues (Scherler et al. Citation2011). Long periods of negative mass balance typically increase the extent of debris cover on glaciers and, along with reduced ice velocities, accumulated debris over extended time periods leads to insulation of the ice surface and reduced local melt. The role of supraglacial debris cover in controlling ice melt is therefore likely to become more significant with the prolonged negative glacier mass balances in the future. The evolving terminus position at Franz Josef Glacier, and glacier access, is of particular importance to the tourism industry (the glacier terminus of Franz Josef Glacier receives c. 200 000 visitors annually; Bogie Citation2007). The effects of debris cover on insulating the ablation zone, the resulting evolution of the terminus and the impact on tourist accessibility over the coming decade are therefore of substantial economic importance to the area.
Ablation studies on New Zealand glaciers have typically been limited to debris-free glacier surfaces, although a small number of studies have taken place on debris-covered ice. These include an analysis of the effect of tephra on ablation on the Summit Plateau icefield, Mt Ruapehu, on the North Island (Richardson & Brook Citation2010). In the Southern Alps, large parts of some glaciers are covered with a varying thickness of debris totalling 92 km2 or 8% of the total glacier area (Anderson and Mackintosh Citation2012). The debris is largely derived by rock fall from the steep alpine slopes. Tasman Glacier has the best record of debris cover and ablation data; Kirkbride and Warren (Citation1999: p. 21) reported the effect of down-glacier increases in debris cover to be ‘dramatic’, with debris cover reversing and increasing ablation gradients. Purdie and Fitzharris (Citation1999) reported that ablation reduction relative to clean ice under 1.1 m of debris was estimated at 93%. Reznichenko et al. (Citation2011) reported clear evidence of ablation reduction under debris cover from geophysical data and surveys of debris-covered ice and topographic development. At Fox Glacier, Purdie et al. (Citation2008) found clear contrasts between ablation rates on clean ice surfaces compared with debris-covered ice, which was particularly significant during summer when ablation rates under debris cover were suppressed by up to 50%. A short-term study of ablation of ice-cored moraine in the foreland of Fox Glacier (Brook & Paine Citation2012) reported melt rates of 1.3–6.7 cm d−1 from debris covers of varying thicknesses, with enhancement of melt rate under thin debris covers.
The effect of debris cover on glacier activity in New Zealand is also of palaeoclimatic signficance, because evidence is emerging that some terminal moraine ridge formation in the past (e.g. the Waiho Loop moraine of Franz Josef Glacier) may be caused or at least largely influenced by an increased supraglacial debris cover in the ablation zone, rather than by pure climatic forcing (e.g. Tovar et al. Citation2008; Shulmeister et al. Citation2009). Modelling shows that a climate-driven advance to the Waiho Loop requires mean temperatures of c. 4–5 °C (Alexander et al. Citation2011) or 3–4 °C (Anderson & Mackintosh Citation2006) less than the present day. Both studies contrast with proxy data, which suggest temperatures no more than c. 2.5 °C cooler than present day during the last glacial–interglacial transition (LGIT) about 12–13 ka BP (Carter et al. Citation2003; Turney et al. Citation2003; Barrows et al. Citation2007). Cosmogenic dating of the Waiho Loop moraine by Barrows et al. (Citation2007) yielded an age of c. 10.5 ka BP, equating to warmer temperatures than during the LGIT and making a climate-driven advance less likely. Tovar et al. (Citation2008) suggested that the ablation zone of Franz Josef Glacier was covered with debris by a rock avalanche, and Alexander et al. (Citation2011) showed that a 65% reduction in total ablation would have been required to cause the required 3 km of advance from the mountain front to the Waiho Loop. Vacco et al. (Citation2010) recently presented a numerical model of Franz Josef Glacier, confirming that a debris-cover-driven advance could reach the Waiho Loop under certain circumstances.
In summary, the precise origin of the Waiho Loop moraine remains equivocal; however, there is substantial evidence that a rock avalanche deposit covering a sufficient proportion of the ablation zone and the effect of an insulating debris cover could cause mass balance fluctuations and a non-climatically driven advance. Hence, an investigation into the effect of debris cover on ablation at one of New Zealand's most active and well-studied glaciers (Franz Josef Glacier) is timely. We present results of a field study of the effect of varying debris thickness on surface melt and, having mapped the debris cover and thickness, estimate the degree to which emerging debris cover on the lower part of Franz Josef Glacier reduces surface melt across the ablation zone.
Study area
Franz Josef Glacier is one of the best-studied Southern Hemisphere glaciers, and is regarded as a key indicator of Southern Hemisphere climate change (Anderson et al. Citation2008). The c. 11 km long glacier occupies 35 km2 on the western flank of the Southern Alps, is c. 6% of Southern Alps total ice volume (; Chinn Citation2001), and descends from a maximum elevation of 2900 m a.s.l. (Alexander et al. Citation2011) to a terminus at c. 280 m a.s.l. The broad accumulation area consists of gently sloping névé basins, which converge into a heavily crevassed steep and narrow tongue. The average equilibrium line altitude (ELA) is currently at c. 1860 m a.s.l. (Anderson et al. Citation2006). Precipitation increases from c. 7 m a−1 at the terminus to c. 12 m a−1 at 600 m a.s.l. (Anderson et al. Citation2006), decreasing to 11 m a−1 at the névé (Stuart Citation2011). Mean annual temperature varies from c. 9 °C at the terminus to approximately −4 °C at the névé (Anderson et al. Citation2008). The corollary is a glacier system with both high accumulation and ablation rates, leading to velocities of c. 1000 m a−1 (Herman et al. Citation2011) and a response time of 9–20 years (Oerlemans Citation1997).
Figure 1 Location map of Franz Josef Glacier, including debris-covered zones at the terminus and the medial moraine (coordinate system: NZTM). Numbers refer to ablation stakes in .
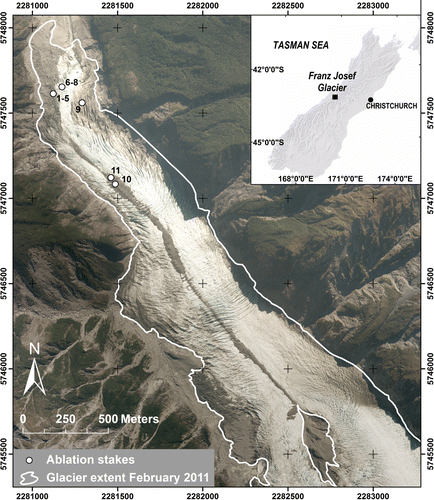
While Franz Josef Glacier has retreated substantially from its Little Ice Age maximum over the 20th century, it advanced >1 km from the mid-1980s until the late 1990s due to cool, wet conditions linked to the El Niño–Southern Oscillation and Interdecadal Pacific Oscillation (Hooker & Fitzharris Citation1999; Chinn et al. Citation2005). Over the last decade, the glacier first retreated and then advanced c. 350 m during mid-2005–2009; it is now undergoing fast retreat and downwastage, leading to the formation of features typical of debris-covered glaciers (). This has been accompanied by an emergence of debris at the snout of the glacier and development of a medial moraine (). The focus of this study is both the debris-covered snout and the medial moraine, previous ablation work at Franz Josef Glacier having solely focused on abundant clean ice surfaces (Marcus et al. Citation1985; Ishikawa et al. Citation1992; Anderson et al. Citation2008) when debris cover was negligible.
Figure 2 Debris cover and landforms on the lower Franz Josef Glacier: A, Differential ablation at the terminus of Franz Josef Glacier, as shown by the contrast in surface elevations of both the debris-covered terminus and medial moraine compared with bare ice surfaces; B, AD (Ablation Dominant-2) type medial moraine (sensu Eyles & Rogerson Citation1978) formed by emergence of englacial debris that has been entrained within the glacier above the equilibrium line altitude (ELA); C, ‘glacier table’ formed by insolation shielding of ice pedestal; D, the debris-covered terminus, with clean ice surfaces visible beneath the <0.5 m thick debris; E, ablation cones forming at the point of emergence of englacial debris at the upper end of the medial moraine under very thin debris (c. 2 cm).
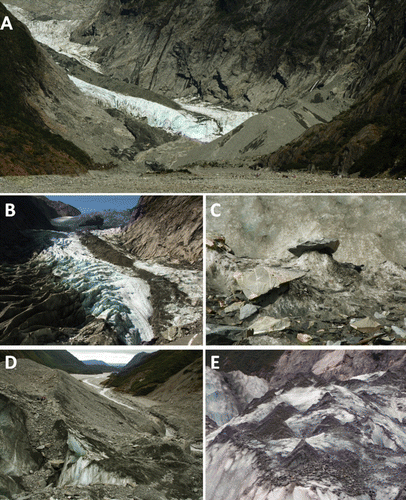
Methods
To determine the effect of a varying supraglacial debris thickness on ice melt, ablation was measured during 7–16 February 2012 using a network of 11 light-grey low-conductivity PVC ablation stakes. These were placed into hand-drilled holes in the debris-covered ice through debris of varying thickness (0–45 cm), covering the entire variability of debris thicknesses observed on the lower tongue of Franz Josef Glacier. Stakes were installed on flat surfaces on areas of continuous, stable debris, with debris thickness measured at the beginning of the study period and at each stake daily over the following 9 days. The altitude of the stake locations ranged 360–480 m a.s.l. Disturbance of the debris cover was minimised as much as possible. This included the use of a steel measuring tape that was extended down the side of each ablation pole to the debris-ice interface, rather than excavating away large areas of debris around stakes each day. For the thinnest debris covers, debris thickness was maintained using a trowel to make sure debris thickness remained consistent.
For air temperature measurements, a Campbell HC2S3 temperature probe inside a radiation shield was attached to a Campbell CR1000 logger, logging at 5 minute intervals, installed on a stanchion 1.5 m above the debris-covered ice at the snout. Rainfall intensity (in mm h−1) was obtained from the NIWA Franz Josef Climate Station (Agent Number 24926). Although variability of daily lapse rates can be high in glacial valleys (e.g. Fujita & Sakai Citation2000), we assumed an elevation gradient of 4.8 °C/1000 m based on Anderson et al. (Citation2006), and this allowed the positive-degree-day (PDD) factor to be calculated for each stake. The PDD method assumes that the amount of ice melt during a period of study is directly proportional to the sum of the mean daily temperatures (calculated from the daily maximum and minimum temperatures) above 0 °C over that period, and enables comparison of ablation between different regions (Braithwaite Citation1995). This allows the calculation of the PDD factor (d f), which is derived from the sum of ablation divided by the sum of positive-degree-days over the period of study. Despite simplifying complex energy fluxes, the PDD factor (in mm d–1 °C−1) has proven to be a very useful parameter where detailed energy balance measurements are lacking (Braithwaite Citation1995).
The relation between PDD and debris thickness can later be used to calculate melt for any given debris thickness and degree-day sum. In order to estimate the influence of debris cover on ice melt across the ablation zone, thickness and spatial distribution of debris was mapped in the field using a hand-held global positioning system (GPS). Areas of similar debris thickness were mapped and then added, in ArcGIS, as shape files onto a georegistered aerial photograph (0.4 m ground resolution) of Franz Josef Glacier () acquired by New Zealand Aerial Mapping in February 2011 (Survey Number: 50850X, Run and Frame: 16/1885). This subdivided the debris-covered areas of the glacier into 79 polygons of homogeneous appearance and known thickness (). For each polygon, the relative deviations from clean ice ablation were calculated. For this purpose, the best-fit regression of an exponential relation between PDD and debris thickness was used. By considering the areas of the polygons, the relative impact of supraglacial debris on surface melt could be estimated.
Results
Cumulative ablation for each of the 11 stakes is reported in along with temperature, which showed typical diurnal variability of c. 9–19 °C. Rainfall, mostly limited to the 14 February, is also reported on and appears to have had little impact on ablation. At selected stakes readings were taken twice daily, and the inflections on the cumulative ablation curves for those stakes () indicate that melt also progressed during night time. This confirms the effect of positive night-time air temperatures that are quite typical for maritime mid-latitudinal glaciers such as Franz Josef Glacier (Ishikawa et al. Citation1992).
Figure 5 Top graph shows the full record of daily ablation measurements at each stake. The lower graphs show temperature logged at 5 minute intervals at an automatic weather station located on the debris-covered ice at the terminus (grey line), and rainfall intensity logged hourly (black bars) at the NIWA Franz Josef climate station (Agent Number 24926).
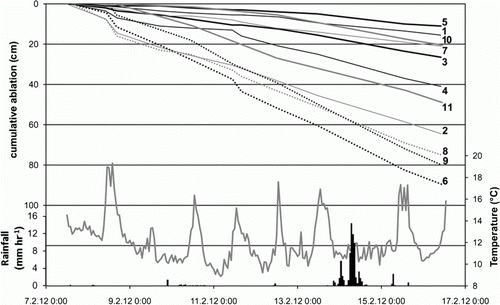
The mean ablation rate of clean ice was 10.1 cm d−1 (). Daily melt rates under debris-covered ice varied from 1.2 to 10.1 cm d−1, under debris cover of 43 cm and 0 cm thickness, respectively, with a mean of 5.3 cm d−1 (, ). The relationship between debris cover and melt rate is shown in A, with the relationship of Østrem (Citation1959) superimposed on the plot. Also included on the plot is an exponential regression, which provided the most representative fit from a series of models that were attempted (linear, polynomial, logarithmic). As can be seen from the plot, ablation decreases asymptotically with increasing debris thickness and, following the exponential regression model reported on the graph, would be close to zero with a debris thickness of c. 85 cm. Nevertheless, there is no evidence for increased ablation relative to clean ice under a very fine debris cover. Indeed, the average ablation rate for bare ice (10.1 cm d−1) was not exceeded at any of our observed locations, which agrees with the findings of Mihalcea et al. (Citation2008).
Figure 6 A, Relationship between ablation and debris cover as measured from the ablation stakes; B, relationship between ablation rate and degree-day factor d f. Grey dots on each plot represent every stake reading (note that stakes under thicker debris covers were not measured every day) to provide an indication of variability. Exponential regression curves have been fitted to the mean daily values (black dots). The curve of Østrem (Citation1959) is also shown for reference.
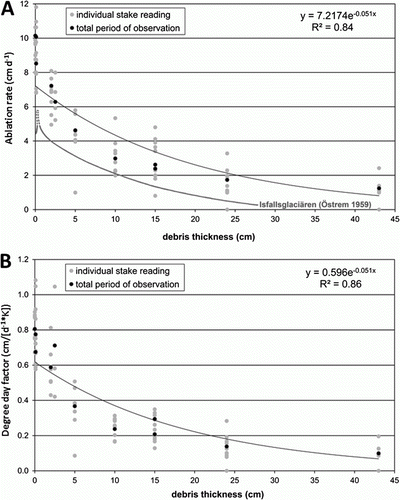
Table 1 Ablation stake location, ablation and temperature (calculated from lapse rates; see text for explanation) data.
To allow direct comparison with other studies, a degree-day factor d f was calculated from air temperature, which can be extrapolated to all of the stakes due to rather constant lapse rates. This temperature index method based on mean air temperature is beneficial because it is strongly connected to the most important energy fluxes in alpine environments, solar radiation and sensible heat flux (Ohmura Citation2001). The air temperature record allowed calculation of d f for all the stakes in this study, with d f varying from 1.1 mm d−1 °C−1 under 43 cm of debris to a maximum of 8.1 mm d−1 °C−1 under bare ice (B), with a mean value of 4.4 mm d−1 °C−1.
The close fit (r 2=0.86) of the exponential regression (y=0.595−0.051x ) to the debris thickness and degree-day melt rates indicates that it should be possible to estimate ablation rates under supraglacial debris if temperature and debris thickness are known. This was undertaken for the ablation zone using the high-resolution aerial photograph in and field mapping of debris thicknesses (). Results show that 0.7 km2 of the 4.9 km2 ablation zone surface is currently debris covered, with thicknesses 1–50 mm. Based on the measured debris thicknesses and d f within the debris-covered area of the glacier, ablation is reduced by a total of 41% in those areas at the present time. Considering the whole ablation area, the current debris cover reduces total ice melt here by 6%.
Discussion
Ablation of clean ice (10.1 cm d−1) closely corresponds to results reported in previous melt studies on the lower Franz Josef Glacier and across the Southern Alps. Owens et al. (Citation1992) reported a rate of 13.7 cm d−1, Gunn (Citation1964) reported a rate of 8.2 cm d−1 and Evans (Citation2003) reported an ablation rate of 7.7 cm d−1. Results from close to the terminus of nearby Fox Glacier are also consistent with our clean ice ablation rates, with Purdie et al. (Citation2008) reporting mean ablation on clean ice surfaces in summer of 12.9 cm d−1, diminishing to c. 2.2 cm d−1 in winter. East of the Main Divide of the Southern Alps at Tasman Glacier, clean ice ablation rates closely correspond to clean ice ablation rates on the western flank of the Southern Alps. Indeed, Kirkbride (Citation1989) recorded mean ablation rates of 8.0 cm d−1 and Purdie and Fitzharris (Citation1999) reported mean clean ice ablation rates of 9.6 cm d−1 at the same location of the ablation area at Tasman Glacier. Although there is a highly seasonal pattern to ablation in the Southern Alps (Purdie et al. Citation2008), clean ice melt rates measured during different ablation seasons at a range of glacier tongues are remarkably similar.
Ablation of debris-covered ice at Franz Josef Glacier appears to follow the general trends outlined in previous studies globally, with ablation being reduced under thicker debris layers (e.g. Østrem Citation1959; Loomis Citation1970; Brock et al. Citation2007). At the same altitude, an increase in debris cover from 2.5 cm to 10 cm is accompanied by a 32% decrease in melt (), showing that a small change in debris thickness causes a marked change in energy available for ice melt. Nevertheless, although our study provides a good approximate match to the general form of the Østrem (Citation1959) curve (showing an asymptotic decline in melt with increasing debris thickness), it does not mimic the ‘rising limb’ of increasing ablation rates under very thin (<2 cm) debris covers, as found in many previous studies (e.g. Mattson & Gardner Citation1991; Mattson et al. Citation1993; Tangborn & Rana Citation2000). By contrast, our results show falling melt rates with increasing debris thickness even for miniscule debris layers. This is consistent with findings of several studies that have emerged over the last decade (Nicholson & Benn Citation2006; Mihalcea et al. Citation2006; Brock et al. Citation2007; Hagg et al. Citation2008; Lambrecht et al. Citation2011).
The lack of an increase in ablation for very thin debris cover is intriguing. Adhikary et al. (Citation2000) found that, rather than being a continuous thickness, very thin debris cover tends to be discontinuous either due to aggregation of particles or by ‘diffusion-loss’ of particles with melt water flow (Adhikary et al. Citation2000). The effect of this is to reduce solar radiation absorption; this limits the ablation rates and may account for the ‘rising limb’ reported in many previous studies. Thus, ‘bare ice’ locations may not actually be absolutely debris free, with albedo lowered by the presence of small amounts of dust and cryoconite, increasing absorption of solar energy.
Such issues regarding accurate characterisation of debris thicknesses and measurements of ablation under thin debris covers are not new. Indeed, in his seminal study, Østrem (Citation1959, p. 228) reported that under thin debris covers (<1 cm) exact measurements were difficult to obtain because of melt water erosion. Close inspection of Østrem's (Citation1959) ablation rate/debris thickness graph (his fig. 1) reveals he actually estimated the ‘rising limb’ section of the ablation rate curve by drawing a dotted line on the plot. Hence, the ‘rising limb’ of the Østrem curve (e.g. Nicholson & Benn Citation2006) was not actually based on empirical observations, highlighting the difficulty of meaningful ablation measurements under very thin debris covers.
While the degree-day factor (d f) for ‘bare’ ice for the present study of 8.1 mm d−1 °C−1 is close to the upper end of the range in d f (5.5–7.7 mm d−1 °C−1) reported for a selection of glaciers globally by Braithwaite (Citation1995), it is higher than the d f of 5.1 mm d−1 °C−1 reported for nearby Fox Glacier by Brook and Paine (Citation2012). The range in d f in this study (1.1–8.1 mm d−1 °C−1) is close to, and in some cases overlaps, ranges of d f values reported from a host of other debris-covered glaciers from different continents. Indeed, d f values of 1.6–10.6 mm d−1 °C−1 for 24K Glacier, southeast Tibet were reported by Wei et al. (Citation2010); Hagg et al. (Citation2008) reported d f of 4.1–9.3 mm d−1 °C−1 for Inylchek Glacier, central Tian Shan; and Brook and Paine (Citation2012) reported a range in d f of 1.2–5.9 mm d−1 °C−1 at Fox Glacier. To allow a closer evaluation with other studies, comparisons of d f for debris thicknesses of 10 cm can be made. Values of d f of 15.6, 14.0, 5.5 and 3.5 mm d−1 °C−1 were reported for the Himalayan AX010, Khumbu, Rakhiot and Lirung glaciers, respectively (Kayastha et al. Citation2000). Rates of 3.8 and 3.5 mm d−1 °C−1 for Fox and Inylchek glaciers, respectively, were reported by Hagg et al. (Citation2008) and Brook & Paine (Citation2012).
The variations in d f between these studies is intriguing, and is mainly due to geographical conditions (elevation, latitude, aspect, shading); local geology and debris layer porosity and permeability (controlling debris surface albedo and thermal resistance); and meteorological conditions (cloudiness and rainfall). This determines the amount of energy reaching the ice–debris interface. Marcus et al. (Citation1985) highlighted the possible effects of rainfall events on ablation, arguing that surface runoff due to rainfall may intercept and advect a substantial portion of incoming energy fluxes, with percolating rainfall advecting heat from the warm debris to the ice (e.g. Reznichenko et al. Citation2010). Ishikawa et al. (Citation1992) found that rainfall contributes very little (2%) to surface melt at Franz Josef Glacier, with this melt being due to the ‘sensible’ heat content of rain.
Indeed, our results indicate that temperature alone as defined by d f is a strong predictor of sub-debris melt. This indicates that heat flux supplied by rain (e.g. ) has little effect on the energy balance at the ice–debris interface, unlike on clean ice surfaces (Ishikawa et al. Citation1992), at Franz Josef Glacier. This is in accord with conclusions from previous studies in the Italian Alps (Brock et al. Citation2010), the Himalaya (Kayastha et al. Citation2000; Takeuchi et al. Citation2000), the Karakorum (Mattson & Gardner Citation1991) and Svalbard (Nicholson & Benn Citation2006). Moreover, statistical modelling by Reid and Brock (Citation2010) on the effect of precipitation on melt beneath a debris layer found that the heat flux from precipitation could be omitted without significant loss in model performance.
Our study confirms that debris cover significantly reduces ablation rates at Franz Josef Glacier, with ablation beneath the debris-covered part of the ablation zone reduced by 41%. The implication of this is that the behaviour of the glacier will to some extent become less sensitive to climate with increased debris cover (e.g. Anderson & Mackintosh Citation2012). The extensive area of debris-covered ice at the terminus of Franz Josef Glacier () is therefore likely to persist for some decades, gradually lowering over time as downwastage proceeds. A similar phenomenon was observed at nearby Fox Glacier by Brook and Paine (Citation2012), although that dead-ice complex was buried by a rather thick debris-flow deposit and did exist for several decades before finally melting completely during the past 10 years.
This study has implications for several recent modelling studies of Franz Josef Glacier (e.g. Shulmeister et al. Citation2009; Vacco et al. Citation2010; Alexander et al. Citation2011; Anderson & Mackintosh Citation2012). The first two models predict that the effect of a late glacial rock avalanche onto the surface of Franz Josef Glacier would have led to an advance independent of any climate forcing, followed by stagnation. Shulmeister et al. (Citation2009) proposed that a realistic rock avalanche would produce a debris cover of 5 m across the ablation zone, leading to a <3 km glacier advance to the Waiho Loop from a position outboard of the present mountain front. In contrast, Vacco et al. (Citation2010) incorporated a debris thickness value of 92 m into their numerical model, which produced a 10 km advance of Franz Josef Glacier from a terminus position within the confining valley (cf. Anderson and Mackintosh, Citation2006). Both models propose that, if a rock avalanche caused an advance of Franz Josef Glacier leading to formation of the Waiho Loop terminal moraine, a significant carpet of ‘hummocky’ moraine would have formed behind the rather well-defined moraine ridge of the Waiho Loop. However, such landforms are not visible on today's surface.
Using the relationship between debris cover and ablation in B, even under a debris thickness of 5 m (as suggested by Shulmeister et al. Citation2009), ablation would be negligible with a theoretical d f of 5.02−12 mm d−1 °C−1. Given the annual positive degree-day values typically recorded at the NIWA Franz Josef Glacier automatic weather station (c. 3600 °C a−1), an extended Franz Josef Glacier tongue under a debris cover of 5 m would exist for many centuries, presumably forming substantial hummocky moraines as downwastage proceeded (e.g. Shulmeister et al. Citation2009) which have since been covered by aggradational fans. Likewise, the estimated 6% present-day debris-cover-driven reduction in ablation across the whole ablation zone is intriguing.
Indeed, a more recent model of late-glacial advance of Franz Josef Glacier by Alexander et al. (Citation2011) proposed that, based on a temperature 2 °C lower than present day, ablation reduction of 65–80% would be required to advance the terminus to the Waiho Loop. The likely corollary is therefore that the spatial extent of current debris cover would need to expand by several orders of magnitude, concomitant with a temperature decrease and/or precipitation increase, before any advance of the Franz Josef terminus can be expected. In reality, the effects of supraglacial rock avalanche debris cover are much more complex than simple ablation reduction. Reduced ablation also leads to less melt water being available for efficient basal sliding, thus ice velocities will likely decrease. The additional weight of supraglacial debris would modify force balances, and basal sliding resistance would be altered due to the transfer of large quantities of rock debris in the subglacial drainage system (as summarised by Alexander et al. Citation2011).
A major challenge of glacier modelling at a regional scale (e.g. Anderson and Mackintosh Citation2012) is in acquiring information on the extent, thickness and thermal properties of supraglacial debris covers for accurate parameterisation of ablation. Most recently, Anderson and Mackintosh (Citation2012) reported that around 92 km2 of glacier area in the Southern Alps (equating to 8% of the total glacier area) is covered by a variable thickness of supraglacial debris. They applied an energy balance model on a regional scale and found that the central Southern Alps glaciers are very sensitive to temperature change. However, they neglected to incorporate a realistic parameterisation of debris thickness, acknowledging that insufficient data existed on the distribution of debris thickness or the relationship between debris thickness and ablation suppression (Anderson & Mackintosh Citation2012). Hence, they simply assumed that ablation calculated by their energy balance model would be reduced by 90% where any debris cover existed. This neglects the pronounced inhomogeneous debris distribution and the varying effects of debris thickness on ablation rates, as identified in the present study. The implication is that such models could be improved by more accurate parameterisation of debris thickness variability, which could be achieved by a combination of ground-surveyed debris thicknesses and satellite-derived debris layer thermal resistances, which have been shown to correlate strongly over entire ablation areas (e.g. Zhang et al. Citation2011).
Conclusion
The results described here represent the first step in research aimed at describing and interpreting the complex relationship between supraglacial debris conditions and climate at this steep mid-latitude maritime glacier. At 11 ablation stakes under different thicknesses of debris, mean melt rates varied over the range 1.2–10.1 cm d−1. Melt rate decreased exponentially with increasing debris thickness, following the general pattern observed in previous investigations. Nevertheless, the enhancement of melt rates under very thin debris caused by increased absorption of shortwave radiation (e.g. Østrem Citation1959) was not detected, possibly because ‘clean’ ice surfaces were not entirely debris free. Temperature measurements from an automatic weather station on the glacier surface allowed the application of a positive degree-day approach to calculate ablation, and allow comparison with other studies globally. Air temperature alone proved to be a strong index of surface melt, and degree-day factors (d f) ranged from 1.1 to 8.1 mm d−1 °C−1 (mean d f=4.4). Field mapping of debris thickness in combination with analysis of an aerial photograph allowed the calculation of melt reduction due to debris cover. Melt reduction is estimated to be 41% under debris-covered ice, which equates to a 6% reduction in melt for the ablation zone as a whole.
The present study has shown that a meaningful relationship between air temperature, melt and debris thickness can be identified at point locations over a short timescale. Furthermore, this kind of study enables a reasonable determination of sub-debris melt, without knowing the thermal properties of the debris layer. Hence, once the relation between debris thickness and degree-day factor is determined, melt can be calculated at all locations of known debris thickness. Thus, the future application of remote sensing data (e.g. Advanced Spaceborne Thermal Emission and Reflection Radiometer or ASTER) for regional-scale detection of debris thickness and energy balance patterns across the Southern Alps, combined with suitable field validation at point locations, would be advantageous. Such work would lead to improved estimates of glacier retreat due to climate change, and help in refining the effect on glacier dynamics of a large rock avalanche onto a glacier surface.
We have shown that, even under a modest supraglacial debris thickness formed from emergent englacial ‘melt-out’ debris, significant ablation reduction can occur. Rock avalanche debris cover, which would likely be of 1–2 orders of magnitude greater thickness than the present debris cover and of greater spatial extent, would therefore have a significant effect on terminus dynamics. This implies that it is important that the influence of supraglacial debris cover is properly assessed in the context of regional palaeoclimatic analyses.
Acknowledgements
We thank the Department of Conservation for allowing fieldwork on Franz Josef Glacier and Massey University for funding Wilfried Hagg's visit and research costs. The work was undertaken while Martin Brook was a senior lecturer in Earth Science within the Institute of Natural Resources, Massey University. New Zealand Aerial Mapping is thanked for prompt provision of an excellent aerial photo. We would like to extend our gratitude to editors Tom Crick and Simon Cox, along with the anonymous reviewers who have assisted in significantly improving this manuscript.
References
- Adhikary , S , Nakawo , M , Seko , K and Shakya , B . 2000 . Dust influence on the melting process of glacier ice: experimental results from Lirung Glacier, Nepal Himalayas . IAHS Publication , 264 : 43 – 52 .
- Alexander , DJ , Davies , TR and Shulmeister , J . 2011 . A steady-state mass-balance model for the Franz Josef Glacier, New Zealand: testing and application . Geografiska Annaler , 93A ( 1 ) : 41 – 54 . doi: 10.1111/j.1468-0459.2011.00003.x
- Anderson , BM and Mackintosh , AN . 2006 . Temperature change is the major driver of late-glacial and Holocene glacier fluctuations in New Zealand . Geology , 34 ( 2 ) : 121 – 134 . doi: 10.1130/G22151.1
- Anderson , BM. , Mackintosh AN 2012 . Controls on mass balance sensitivity of maritime glaciers in the Southern Alps, New Zealand: The role of debris-cover . Journal of Geophysical Research 117 : F01003 , doi: 10.1029/2011JF002064
- Anderson , B , Lawson , W , Owens , I and Goodsell , B . 2006 . Past and future mass balance of ‘Ka Roimata o Hine Hukatere’ Franz Josef Glacier, New Zealand . Journal of Glaciology , 52 ( 179 ) : 597 – 607 . doi: 10.3189/172756506781828449
- Anderson , B , Lawson , W and Owens , I . 2008 . Response of Franz Josef Glacier Ka Roimata o Hine Hukatere to climate change . Global and Planetary Change , 63 : 23 – 30 . doi: 10.1016/j.gloplacha.2008.04.003
- Barrows , TT , Lehman , SJ , Fifield , KL and De Deckker , P . 2007 . Absence of cooling in New Zealand and the adjacent ocean during the Younger Dryas chronozone . Science , 318 : 86 – 89 . doi: 10.1126/science.1145873
- Bogie D 2007 . Review: Franz Josef Glacier & Fox Glacier (Terminal Faces) Hazard identification and management . Wellington , New Zealand , Department of Conservation .
- Braithwaite , RJ . 1995 . Positive degree-day factors for ablation on the Greenland Ice Sheet studied by energy-balance modelling . Journal of Glaciology , 41 ( 137 ) : 153 – 160 .
- Brock , B , Rivera , A , Casassa , G , Bown , F and Acuna , C . 2007 . The surface energy balance of an active ice-covered volcano: Villarrica Volcano, southern Chile . Annals of Glaciology , 45 : 104 – 114 . doi: 10.3189/172756407782282372
- Brock BW , Mihalcea C , Kirkbride MP , Diolaiuti G , Cutler MEJ , Smiraglia C 2010 . Meteorology and surface energy fluxes in the 2005-2007 ablation seasons at the Miage debris-covered glacier, Mont Blanc Massif, Italian Alps . Journal of Geophysical Research: Atmospheres 115 : Article Number: D09106 doi: 10.1029/2009JD013224 .
- Brook MS , Paine S 2012 . Ablation of ice-cored moraine in a humid maritime climate: Fox Glacier, New Zealand . Geografiska Annaler 94A : 339 – 349 . doi: 10.1111/j.1468-0459.2011 .
- Carter , L , Manighetti , B and Neil , H . 2003 . From icebergs to pongas: Antarctica's ocean link with New Zealand . Water and Atmosphere , 11 ( 3 ) : 30 – 31 .
- Chinn , TJ . 2001 . Distribution of the glacial water resources of New Zealand . Journal of Hydrology (NZ) , 40 ( 2 ) : 139 – 187 .
- Chinn , TJH , Winkler , S , Salinger , MJ and Haakensen , N . 2005 . Recent glacier advances in Norway and New Zealand – a comparison for their glaciological and meteorological causes . Geografiska Annaler , 87A : 141 – 157 . doi: 10.1111/j.0435-3676.2005.00249.x
- Cuffey , W and Paterson , WSB . 2010 . The Physics of Glaciers , Burlington : Butterworth-Heinemann .
- Evans E 2003 . Ablation at the terminus of the Franz Josef Glacier . BSc (Hons) dissertation . Otago University , New Zealand .
- Eyles , N and Rogerson , RJ . 1978 . A framework for the investigation of medial moraines formation: Austerdalsbreen, Norway, and Berendon Glacier, British Columbia, Canada . Journal of Glaciology , 20 : 99 – 113 .
- Fujita , K and Sakai , A . 2000 . Air temperature environment on the debris covered area of Lirung Glacier, Langtang Valley, Nepal Himalayas . IAHS Publication , 264 : 83 – 88 .
- Gunn , BM . 1964 . Flow rates and secondary structures of Fox and Franz Josef Glaciers, New Zealand . Journal of Glaciology , 5 ( 38 ) : 173 – 190 .
- Hagg , W , Mayer , C , Lambrecht , A and Helm , A . 2008 . Sub-debris melt rates on southern Inylchek Glacier, central Tian Shan . Geografiska Annaler , 90A ( 1 ) : 55 – 63 . doi: 10.1111/j.1468-0459.2008.00333.x
- Herman , F , Anderson , B and Leprince , S . 2011 . Mountain glacier velocity variation during a retreat/advance cycle quantified using sub-pixel analysis of ASTER images . Journal of Glaciology , 57 ( 202 ) : 197 – 207 . doi: 10.3189/002214311796405942
- Hooker , BL and Fitzharris , BB . 1999 . The correlation between climatic parameters and the retreat and advance of Franz Josef Glacier, New Zealand . Global and Planetary Change , 22 ( 1–4 ) : 39 – 48 . doi: 10.1016/S0921-8181(99)00023-5
- Ishikawa , N , Owens , IF and Sturman , AP . 1992 . Heat balance characteristics during fine periods on the lower parts of the Franz Josef Glacier, South Westland, New Zealand . International Journal of Climatology , 12 : 397 – 410 . doi: 10.1002/joc.3370120407
- Kayastha , RB , Takeuchi , Y , Nakawo , M and Ageta , Y . 2000 . Practical prediction of ice melting beneath various thickness of debris cover on Khumbu Glacier, Nepal, using a positive degree-day factor . IAHS Publication , 264 : 71 – 81 .
- Kirkbride MP 1989 . The influence of sediment budget on geomorphic activity of the Tasman Glacier, Mount Cook National Park, New Zealand . PhD thesis, University of Canterbury, New Zealand .
- Kirkbride , M and Warren , CR . 1999 . Tasman Glacier, New Zealand: 20th-century thinning and predicted calving retreat . Global and Planetary Change , 22 : 11 – 28 . doi: 10.1016/S0921-8181(99)00021-1
- Lambrecht , A , Mayer , C , Hagg , W , Popovnin , V , Rezepkin , A , Lomidze , N and Svanadze , D . 2011 . A comparison of glacier melt on debris-covered glaciers in the northern and southern Caucasus . The Cryosphere Discussions , 5 : 431 – 459 . doi: 10.5194/tcd-5-431-2011
- Loomis SR 1970 . Morphology and structure of an ice-cored medial moraine, Kaskawulsh Glacier, Yukon . In : Loomis SR , Dozier J , Ewing KJ Studies of Morphology and Stream Action on Ablating Ice . Research Paper No. 57 , Arctic Institute of North America Pp. 1 – 56 .
- Marcus , MG , Moore , RD and Owens , IF . 1985 . Short-term estimates of surface-energy transfers and ablation on the lower glacier, Franz Josef Glacier, South Westland, New Zealand . New Zealand Journal of Geology and Geophysics , 28 ( 3 ) : 559 – 567 . doi: 10.1080/00288306.1985.10421208
- Mattson , LE . 2000 . The influence of a debris cover on the mid-summer discharge of Dom Glacier, Canadian Rocky Mountains . IAHS Publication , 264 : 25 – 33 .
- Mattson , LE and Gardner , JS . 1991 . Energy exchanges and ablation rates on the debris covered Rakhiot Glacier, Pakistan . Zeitschrift für Gletscherkunde und Glazialgeologie , 25 ( 1 ) : 17 – 32 .
- Mattson , LE , Gardner , JS and Young , GJ . 1993 . Ablation on debris covered glaciers: an example from the Rakhiot Glacier, Panjab, Himalaya . IAHS Publication , 218 : 289 – 296 .
- Mayer , C , Lambrecht , A , Hagg , W and Narozhny , Y . 2011 . Glacial debris cover and melt water production for glaciers in the Altay, Russia . The Cryosphere Discussions , 5 : 401 – 430 . doi: 10.5194/tcd-5-401-2011
- Mihalcea , C , Mayer , C , Diolaiuti , G , Lambrecht , A , Smiraglia , C and Tatari , G . 2006 . Ice ablation and meteorological conditions on the debris-covered area of Baltoro glacier, Karakoram, Pakistan . Annals of Glaciology , 43 : 292 – 300 . doi: 10.3189/172756406781812104
- Mihalcea , C , Brock , BW , Diolaiuti , G , D'Agata , C , Citterio , M , Kirkbride , MP , Cutler , MEJ and Smiraglia , C . 2008 . Using ASTER satellite and ground-based surface temperature measurements to derive supraglacial debris cover and thickness patterns on Miage Glacier (Mont Blanc Massif, Italy) . Cold Regions Science and Technology , 52 : 341 – 354 . doi: 10.1016/j.coldregions.2007.03.004
- Nakawo , M and Young , GJ . 1981 . Field experiments to determine the effect of a debris layer on ablation of glacier ice . Annals of Glaciology , 2 : 85 – 91 . doi: 10.3189/172756481794352432
- Nicholson , L and Benn , DI . 2006 . Calculating ice melt beneath a debris layer using meteorological data . Journal of Glaciology , 52 ( 178 ) : 463 – 470 . doi: 10.3189/172756506781828584
- Oerlemans , J . 1997 . Climate sensitivity of Franz Josef Glacier, New Zealand, as revealed by numerical modelling . Arctic and Alpine Research , 29 ( 2 ) : 233 – 239 . doi: 10.2307/1552052
- Oerlemans , J . 2001 . Glaciers and climate change , Rotterdam : Balkema .
- Oerlemans , J . 2005 . Extracting a climate signal from 169 glacier records . Science , 308 : 675 – 677 . doi: 10.1126/science.1107046
- Ohmura , A . 2001 . Physical basis for the temperature-based melt index method . Journal of Applied Meteorology , 40 : 753 – 761 . doi: 10.1175/1520-0450(2001)040%3C0753:PBFTTB%3E2.0.CO;2
- Østrem , G . 1959 . Ice melting under a thin layer of moraine and the existence of ice cores in moraine ridges . Geografiska Annaler , 41 : 228 – 230 .
- Owens IF , Sturman AP , Ishikawa N 1992 . High rates of ablation on the lower part of the Franz Josef Glacier, South Westland . Proceedings of the New Zealand Geographical Society , Hamilton : 576 – 582 .
- Popovnin , VV and Rozova , AV . 2002 . Influence of sub-debris thawing on ablation and runoff of the Djankuat Glacier in the Caucasus . Nordic Hydrology , 33 : 79 – 94 .
- Purdie , J and Fitzharris , BB . 1999 . Processes and rates of ice loss at the terminus of Tasman Glacier, New Zealand . Global and Planetary Change , 22 : 79 – 91 . doi: 10.1016/S0921-8181(99)00027-2
- Purdie , H , Brook , MS and Fuller , IC . 2008 . Seasonal variation in ablation and surface velocity on a temperate maritime glacier: Fox Glacier, New Zealand . Arctic, Antarctic, and Alpine Research , 40 ( 1 ) : 140 – 147 . doi: 10.1657/1523-0430(06-032)[PURDIE]2.0.CO;2
- Reid , TD and Brock , BW . 2010 . An energy-balance model for debris-covered glaciers including heat conduction through the debris layer . Journal of Glaciology , 56 ( 199 ) : 903 – 916 . doi: 10.3189/002214310794457218
- Reznichenko , N , Davies , T , Shulmeister , J and McSaveney , M . 2010 . Effects of debris on ice-surface melting rates: an experimental study . Journal of Glaciology , 56 ( 197 ) : 384 – 394 . doi: 10.3189/002214310792447725
- Reznichenko , NV , Davies , TRH and Alexander , DJ . 2011 . Effects of rock avalanches on glacier behaviour and moraine formation . Geomorphology , 132 ( 3–4 ) : 327 – 338 . doi: 10.1016/j.geomorph.2011.05.019
- Richardson , J and Brook , MS . 2010 . Ablation of debris-covered ice: some effects of the 25 September 2007 Mt Ruapehu eruption . Journal of the Royal Society of New Zealand , 40 : 45 – 55 . doi: 10.1080/03036758.2010.494714
- Scherler , D , Bookhagen , B and Strecker , MR . 2011 . Spatially variable response of Himalayan glaciers to climate change affected by debris cover . Nature Geoscience , 4 ( 3 ) : 156 – 159 . doi: 10.1038/ngeo1068
- Shukla , A , Arora , MK and Gupta , RP . 2010 . Synergistic approach for mapping debris-covered glaciers using optical–thermal remote sensing data with inputs from geomorphometric parameters . Remote Sensing of Environment , 114 : 1378 – 1387 . doi: 10.1016/j.rse.2010.01.015
- Shulmeister , J , Davies , TR , Evans , DJA , Hyatt , OM and Tovar , DS . 2009 . Catastrophic landslides, glacier behaviour and moraine formation - A view from an active plate margin . Quaternary Science Reviews , 28 ( 11–12 ) : 1085 – 1096 . doi: 10.1016/j.quascirev.2008.11.015
- Smiraglia , C , Diolaiuti , G , Casati , D and Kirkbride , MP . 2000 . Recent areal and altimetric variations of Miage Glacier (Monte Bianco massif, Italian Alps) . IAHS Publication , 264 : 227 – 233 .
- Stuart SJ 2011 . Observations and modelling of precipitation in the Southern Alps of New Zealand . MSc thesis, Antarctic Research Centre, Victoria University of Wellington .
- Takeuchi , N , Kohshima , S , Yoshimura , Y , Seko , K and Fujita , K . 2000 . Characteristics of cryoconite holes on a Himalayan glacier, Yala Glacier Central Nepal . Bulletin of Glacier Research , 17 : 51 – 59 .
- Tangborn , W and Rana , B . 2000 . Mass balance and runoff of the partially debris-covered Langtang Glacier, Nepal . IAHS Publication , 264 : 99 – 108 .
- Tovar , DS , Shulmeister , J and Davies , TR . 2008 . Evidence for a landslide origin of New Zealand's Waiho Loop moraine . Nature Geoscience , 1 ( 8 ) : 524 – 526 . doi: 10.1038/ngeo249
- Turney , CSM , McGlone , MS and Wilmshurst , JM . 2003 . Asynchronous climate change between New Zealand and the North Atlantic during the last deglaciation . Geology , 31 ( 3 ) : 223 – 226 . doi: 10.1130/0091-7613(2003)031%3C0223:ACCBNZ%3E2.0.CO;2
- Vacco , DA , Alley , RB and Pollard , D . 2010 . Glacial advance and stagnation caused by rock avalanches . Earth and Planetary Science Letters , 294 ( 1–2 ) : 123 – 130 . doi: 10.1016/j.epsl.2010.03.019
- Wei , Y , Tandong , Y , Baiqing , X and Hang , Z . 2010 . Influence of supraglacial debris on summer ablation and mass balance in the 24K Glacier, southeast Tibetan Plateau . Geografiska Annaler , 92A : 353 – 360 . doi: 10.1111/j.1468-0459.2010.00400.x
- Zhang , Y , Fujita , K , Liu , SY , Liu , Q and Nuimura , T . 2011 . Distribution of debris thickness and its effect on ice melt at Hailuogou glacier, southeastern Tibetan Plateau, using in situ surveys and ASTER imagery . Journal of Glaciology , 57 ( 206 ) : 1147 – 1157 . doi: 10.3189/002214311798843331