Abstract
Newly determined Late Cretaceous 40Ar/39Ar ages on megacrystic kaersutite from four lamprophyre dikes, and a U–Pb zircon age on a trachyte, from central and north Westland (New Zealand) are presented. These ages suggest that the intrusion of mafic dikes (88–86 and 69 Ma) was not necessarily restricted to the previously established narrow age range of 80–92 Ma. The younger lamprophyre and trachyte dikes (c. 68–70 Ma) imply that tensional stresses in the Western Province were either renewed at this time, or that extension and related magmatism continued during opening of the Tasman Sea. Extension-related magmatism in the region not only preceded Tasman seafloor spreading initiation (starting at c. 83 Ma, lasting to c. 53 Ma), but may have sporadically continued for up to 15 Ma after continental break-up.
Introduction
Cretaceous–Paleogene rifting of the Gondwana margin thinned the continental crust of Zealandia and eventually culminated in the opening of the Tasman Sea between Australia and New Zealand (e.g. Bradshaw Citation1989; Tulloch & Kimbrough Citation1989; Laird & Bradshaw Citation2004; Schellart et al. Citation2006). In New Zealand, the earliest stages of thinning (c. 110–100 Ma) were associated with the formation of metamorphic core complexes (e.g. Tulloch & Kimbrough, Citation1989; Spell et al. Citation2000), accompanied by emplacement of granitoid plutons (Muir et al. Citation1997; Waight et al. Citation1997, Citation1998a; Tulloch et al. Citation2009). Progressive extension is expressed in the deposition of terrestrial Pororari Group sediments in extensional grabens across central Westland (e.g. Nathan Citation1978; White Citation1987; Beggs et al. Citation2008) and equivalents in the region offshore of Westland (Bishop Citation1992). Mafic dikes concentrated in the swarms of the Paparoa and Hohonu Ranges (Wellman & Cooper Citation1971; Hunt & Nathan Citation1976; Adams & Nathan Citation1978; Grindley & Oliver Citation1979; Waight et al. Citation1998b) intruded during the later stages of thinning. Felsic and mafic magmatic activity at c. 82 Ma (Waight et al. Citation1998b) is significant because it coincides with the age of the oldest seafloor in the Tasman Sea (Weissel & Hayes Citation1977; Veevers et al. Citation1991). It has therefore been regarded as magmatism coincident with the initiation of seafloor spreading that lasted until c. 53.3 Ma (Gaina et al. Citation1998). At the onset of seafloor spreading, the New Zealand microcontinent (Zealandia) formed through separation from Australia and Antarctica.
The granitoids and the core complex fabrics in the Western Province have been dated using U–Pb zircon and 40Ar/39Ar methods (Kimbrough & Tulloch 1989; Tulloch & Kimbrough Citation1989; Muir et al. Citation1997; Spell et al. Citation2000; Sagar & Palin 2011), and the occurrences of Cenozoic mafic magmatism in the Western Province and elsewhere in New Zealand have recently been reviewed by Timm et al. (Citation2010). The timing of Late Cretaceous–Early Cenozoic lamprophyric and other mafic magmatism in the Western Province, coeval with extension and rifting, is much less precisely known. Initial K/Ar dating of dikes in the Hohonu Range and surrounding areas by Wellman & Cooper (Citation1971) yielded a scatter of ages that they suggested represented an emplacement age of between c. 137 and 107 Ma. The majority of these ages have since been shown to be geologically implausible because the dikes intrude granitoids dated to be around 110 Ma (Waight et al. Citation1997). Waight (Citation1995) and Waight et al. (Citation1998b) argued on the base of cross-cutting relationships that lamprophyres in the Hohonu Range were instead intruded primarily before, but also synchronous with, the intrusion of the c. 82 Ma French Creek Granite (Tulloch et al. Citation1994; Waight et al. Citation1998b).
Thus far, the only reliable absolute ages of lamprophyric magmatism in Westland are those of Adams & Nathan (Citation1978) who obtained K/Ar ages of 80–92 Ma (recalculated to decay constants of Steiger & Jäger Citation1977, see ) from the Buller Gorge, in agreement with their cross-cutting relationships with coarse-grained non-marine sedimentary rocks of the Pororari Group. Although these and other older K/Ar ages effectively represent total gas ages with no control over the effect of possible open system behaviour in the whole rock (argon loss or uptake post-dating emplacement), they are at this time the best approximation of the actual intrusion age of most of the dikes in Westland.
Table 1 Recalculation of K/Ar ages of lamprophyre dikes and a basalt sill reported by Adams & Nathan (Citation1978) to decay constants of Steiger & Jäger (Citation1977).
Table 2 Results of 40Ar/39Ar analyses by laser heating at Universität Potsdam and Lund University (OU79915, OU79943, OU79956 and KST-2).
Camptonitic lamprophyre dikes also occur in the Victoria Range (Tulloch Citation1979) and have now been located in the Bonar Range, c. 130 km south of the Buller area, where they cut sillimanite-grade gneisses (Jongens Citation2006; Scott et al. Citation2011a). Lamprophyric dikes are also reported between Bald Hill and the southernmost parts of Westland (south Westland: Nathan Citation1977, Citation1978; Bald Hill: Ryland Citation2011; Haast area: Randall Citation2004). In south Westland, the 64.8 ± 0.8 Ma Arnott Basalt (Timm et al. Citation2010) and a 61.4 ± 0.8 Ma rhyolite clast (Phillips et al. Citation2005) remain the only accurately dated magmatic events during Tasman Sea spreading. Mafic dikes have also been found in the deformed Fraser Complex and Granite Hill Complex (Rattenbury Citation1991; Waight et al. Citation1997). The vast majority of the dikes found in the Fraser Complex were deformed during mylonitization of the surrounding country rock (Rattenbury Citation1991) but this does not seem to be the case for dikes found on Granite Hill (Waight Citation1995; Waight et al. Citation1997).
Apart from mafic lamprophyres, more felsic quartz trachyte and phonolitic dikes occur sporadically. Two trachyte dikes have previously been dated by K/Ar in biotite, yielding ages of 64 and 73 Ma (Tulloch & Kimbrough Citation1989) These trachytes occur in the upper plate of the Paparoa metamorphic core complex and in the Victoria Range, and record extension during Tasman Sea spreading (Tulloch & Kimbrough Citation1989; Beggs et al. Citation2008).
In this paper, we document new radiometric ages for a selection of rocks from central Westland. 40Ar/39Ar ages are reported for lamprophyre dikes from Lake Brunner (or Lake Moana), the Hohonu Ranges and the Bonar Range (); these ages are supported by biotite 40Ar/39Ar cooling ages from Bonar Range orthogneiss. This new information reveals that the lamprophyre dike swarms represent either one prolonged or two (or more) distinct periods of extension and magmatism. We also report a U–Pb zircon age for a deformed trachyte dike in the Fraser Complex. Although imprecise, the interpreted age overlaps with ages of undeformed dikes outside the complex. The Fraser Complex protolith therefore appears to have experienced magmatism and tectonism similar to that of basement rocks of the Western Province.
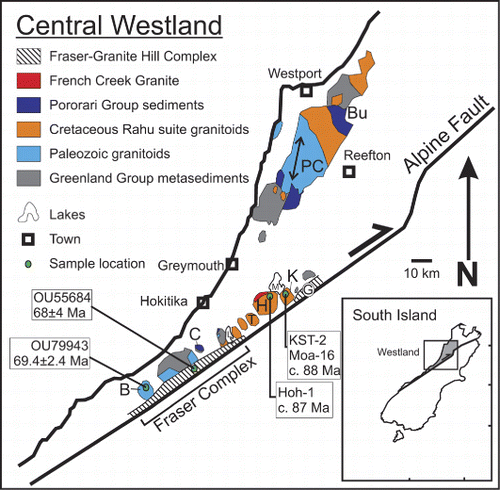
Methods
40Ar/39Ar geochronology
Hand-picked mineral separates were analysed using the 40Ar/39Ar method at the University of Nevada Las Vegas (UNLV) (samples Hoh-1, Moa-16), Universität Potsdam in Germany (OU 79943, 79956 and 79915) and Lund University in Sweden (KST-2). The results are reported in . Details of the analytical methods used at UNLV have been reported in the Appendix to Scott et al. (Citation2011b), and analytical procedures of 40Ar/39Ar dating at Universität Potsdam are summarized in Wiederkehr et al. (Citation2009). Sample KST-2 was analysed at Lund University, Sweden following the methods described by Hermansson et al. (Citation2008). Data from UNLV and from Universität Potsdam were standardized against FC-2, Fish Canyon tuff sanidine (with an assumed age of 28.02 ± 0.28 Ma after Renne et al. (Citation1998)) at UNLV, whereas an age of 27.5 Ma (after Uto et al. Citation1997) was assumed for analyses carried out at Universität Potsdam, the reported data are corrected for this minor difference and are all standardized against the age after Renne et al. (Citation1998). Data from Lund University were standardized against Taylor Creek rhyolite (TCR) sanidine (28.34 ± 0.28 Ma, Renne et al. Citation1998).
Total gas ages are calculated by weighting by the amount of 39Ar released. A plateau age should consist of three or more contiguous gas fractions with analytically indistinguishable ages (i.e. all plateau steps overlap in age at ± 2σ analytical error) and comprise a significant portion of the total gas released (typically > 50%). For each sample, if possible, inverse isochron diagrams were constructed to check for the effects of excess argon. Reliable isochrons are based on the mean squared weighted deviates (MSWD) criteria of Wendt & Carl (Citation1991) and, as for plateaux, must comprise contiguous steps and a significant fraction of the total gas released. Ages reported are at the 2σ (standard deviation) confidence level. For samples that did not yield a plateau age by these criteria, the age is approximated with a weighted mean of representative heating steps. In this case, the heating steps represent a significant fraction of the gas released but do not produce a real plateau age because one or more steps deviate more than 2σ. The determination of the preferred age for each sample is discussed below.
U–Pb geochronology
Zircon grains were extracted using conventional crushing and heavy liquid separation techniques, mounted in epoxy and then imaged under cathodoluminescence using the JEOL JXA-8600 microprobe at the University of Otago geology department. U–Th–Pb analyses were conducted by laser ablation inductively coupled plasma mass spectrometry (LA-ICP-MS) at the Australian National University, Canberra. All data were standardized against TEMORA-2 zircon and NIST-610 glass. The data acquisition method is the same as reported in detail by Scott & Palin (Citation2008). Data points were excluded from age calculations if they had an internal MSWD of > 10 or if they were more than 10% discordant. Reported ages are at 2σ confidence level.
Sample setting and results
Lake Brunner and the Hohonu Range
The geology of the region surrounding Lake Brunner and the Hohonu Range is dominated by Cretaceous granitoids intruding Early Paleozoic Greenland Group metasedimentary rocks of the Buller Terrane (Waight et al. Citation1997, Citation1998a, Citation1998b; Suggate & Waight Citation1999). The Cretaceous granitoids (114–109 Ma), which are the most voluminous group of basement rocks in this area, are part of the I–S-type Rahu Suite (Waight et al. Citation1997, Citation1998a). A prominent exception is the volumetrically minor A-type French Creek Granite (81.7 ± 1.2 Ma) on the western flank of the Hohonu Range (Waight et al. Citation1997, Citation1998b). Most plutons are intruded by lamprophyric to doleritic and rare phonolitic/trachytic dikes of the Hohonu Dike Swarm. On the basis of field relationships, Waight et al. (Citation1998b) inferred that the dikes are primarily older than, but also extend to younger than, the French Creek Granite. Rare composite dikes indicate contemporaneous mafic and silicic magmatism. The dikes may represent up to 40% of outcrop or stream boulders and several stream traverses indicate that c. 7% crustal dilation occurred during dike emplacement (Waight et al. Citation1998b). This indicates that, at least locally, dike magmatism accompanied significant crustal extension. Dike abundances decrease dramatically to the south and north of the Hohonu Range and Mount Te Kinga, with c. 30 dikes observed on Mount Turiwhate, and only two dikes found on Mount Tuhua directly west of Lake Kaniere (). The dike swarm shows a strong WNW–ESE preferred orientation, which parallels the line of opening of the Tasman Sea, and the age of the French Creek Granite (Waight et al. Citation1998b) overlaps with the oldest known oceanic crust in the Tasman Sea (Weissel & Hayes Citation1977), all strongly indicative that the alkaline magmatism of the Hohonu Dike Swarm and French Creek Granite was intimately linked to crustal extension and the early stages of break-up of New Zealand and Australia (Waight et al. Citation1998b).
Sample description
The dated samples (Hoh-1, Moa-16 and KST-2) from Lake Brunner and the Hohonu Range are lamprophyres that were targeted due to the presence of megacrystic kaersutite (K–Ti-rich amphibole, see ). KST-2 was collected from an in situ composite dike in an unnamed creek draining into Lake Brunner. The dike is c. 2 m wide and comprises an outer margin of phonolite grading over a short c. 10 mm distance into lamprophyre containing abundant kaersutite megacrysts and spinel harzburgite xenoliths. It is likely to be a similar dike to that collected as a stream boulder for this study (Moa-16) and to the dike containing the mantle nodules described in detail by Tulloch & Nathan (Citation1990). shows the boulder from which Moa-16 originates, with the visible large black kaersutite grains and similar sized peridotite xenoliths. In thin section, megacrystic kaersutite is orange brown–pale brown pleochroic and clear, rarely containing sparse up to 2 mm opaque inclusions. Sub-mm-wide veinlets of groundmass material intrude megacrystic kaersutite from the rims (). The megacrystic kaersutite differs slightly from lamprophyre groundmass kaersutite because of the smooth shape (as opposed to euhedral crystals) and a commonly occurring sub-mm-thick (commonly partially resorbed) rim around megacrystic kaersutite on the outermost edges. The size of kaersutite ranges from several cm, visible in outcrop, down to c. 0.05 cm, slightly larger than euhedral kaersutite found in the groundmass. Different sections of the dike show a spread of matrix kaersutite ranging between 0.05 and 0.2 mm in the smallest dimension, commonly showing an aspect ratio of c. 1:4, kaersutite makes up between 10 and 50% of the groundmass. The remainder of the groundmass is made up of subhedral c. 0.1 × 0.7 mm grainy feldspar; extremely fine but up to 0.2 mm-long apatite needles are pervasive through the feldspar fabric. Opaque minerals range in size from < 0.01 mm, commonly up to 0.2 mm, and sometimes ranging up to a cm in size. Interstitial spaces between feldspar are filled with green, fine-grained aggregates of epidote and up to 1 mm sized carbonate. The typical carbonate + epidote and rarely fine-grained albite ocelli occur as up to 1 cm large patches, interpreted to represent the latest crystallizing volatile-rich phases. Anhedral to euhedral clinopyroxene that is rarely zoned and commonly partially altered to a fine-grained epidote aggregate occurs in sizes similar to megacrystic kaersutite (in thin section) and is interpreted to represent the xenocrystic equivalent originating from peridotite xenoliths with the rims representing possible magmatic overgrowth. The host dike can be classified as a camptonite (Rock Citation1987).
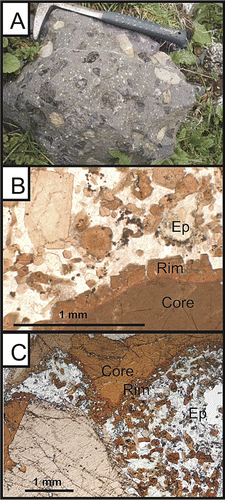
The dated kaersutite sample Hoh-1 comes from a lamprophyre dike intruding granite on a ridge crest in the Hohonu Range. This dike also contains xenoliths of granite and small xenoliths of spinel peridotite, along with xenocrysts of clinopyroxene, feldspar and spinel. The lamprophyre is inequigranular and composed of phenocrysts of augite (1 mm) and chlorite–tremolite pseudomorphs of olivine (1.5 mm) set in a groundmass composed of kaersutite, biotite, plagioclase and magnetite. Kaersutite megacrysts display a slight zoning at the rims (). There are abundant xenocrysts of quartz or feldspar, and xenoliths of granite, always with a reaction corona of fine prismatic crystals of augite around their margins. Many mafic mineral species show marginal chloritization. Ocelli of coarse-grained calcite occur sporadically. Mineralogically, the rock is an alkali lamprophyre and the dominance of groundmass plagioclase indicates that it is a typical camptonite. The presence of granitic material in disequilibrium with the mafic assemblage in the Hoh-1 sample indicates minor contamination by the granitic wallrock.
Geochronology
40Ar/39Ar data for Hoh-1 do not fit criteria for calculating a plateau or isochron age. The total gas age is c. 80 Ma. However, the first three steps (15% of the 39Ar released) have distinctly young ages (33–77 Ma and disrupted Ca/K values, see ), possibly representing young disturbance. If these three steps are excluded from the age calculation on the basis that they represent out-gassing of alteration patches or inclusions in the kaersutite, the weighted average age is 86.8 ± 1.0 Ma, which incorporates 80.8% of the 39Ar released (). This age is taken to be the age of dike intrusion. Moa-16 also fails to meet criteria for a plateau or an isochron (). The total gas age is c. 86 Ma; however, this pooled age includes the disturbed first four steps in the age spectrum (3.8% 39Ar released, with variable Ca/K values, see ). If these data points are excluded, the remaining data give a weighted average age of 86.4 ± 2.7 Ma ().
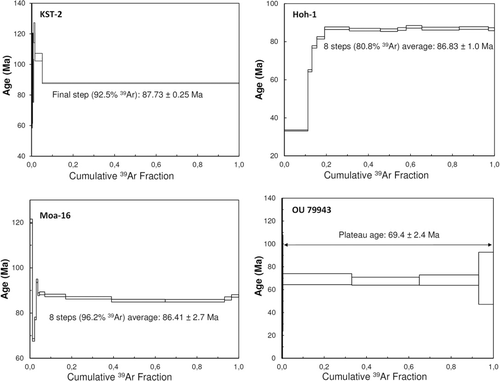
Table 3 Results of 40Ar/39Ar analyses at UNLV (Hoh-1 and Moa-16).
40Ar/39Ar data in KST-2 do not fit the criteria for calculating a plateau or isochron age because during step heating c. 95% of total argon was released in the last step (). The first heating steps yielded highly variable ages between 60 and 120 Ma; these steps are excluded from the age calculation on the basis that they may represent out-gassing of alteration patches or inclusions in the kaersutite. The age of the final heating step (87.7 Ma) may provide a closer approximation of the actual age of intrusion. This age overlaps with those obtained for Moa-16 and Hoh-1 ().
Bonar Range
The Bonar Range is composed of Devonian–Carboniferous orthogneisses and Early Paleozoic metasedimentary gneisses representing reworked Palaeozoic S-type granites and Greenland Group metasedimentary rocks, respectively (Jongens Citation2006; Scott et al. Citation2011a). U–Th–Pb ages of 370–350 Ma for monazite that has grown along biotite cleavage in one paragneiss was interpreted as indicating that metamorphism and deformation occurred during the Devonian–Carboniferous, coeval with the extensive S-type Karamea Suite granitic magmatism in the Western Province (Scott et al. Citation2011a). Although several mafic dikes cutting the gneissic country rock have been identified, they are less common than in the Hohonu–Lake Brunner area.
Biotite fractions from two orthogneisses (OU 79956 and OU 79915, see ), and a kaersutite fraction from a phenocryst in a lamprophyre dike (OU 79943, see ) were selected for 40Ar/39Ar chronology. Biotite in OU 79956 has a total gas age of 94.9 ± 0.5 Ma. The data meet the criteria for a plateau, which has an age of 95.7 ± 0.4 Ma for 99.8% of the 39Ar released, as well as an isochron, which has an age of 95.5 ± 1.2 Ma and a 40Ar/36Ar value of 289 ± 32. Although the ages are effectively the same, the isochron 40Ar/36Ar value is statistically indistinguishable from atmospheric Ar (295.5) and is taken as the best result. 40Ar/39Ar data for the biotite fraction in OU 79915 have a total gas age of 91.3 ± 0.4 Ma, but this age incorporates a distinctly young initial step. The four central steps (54% of the 39Ar released) meet the criteria of a plateau, and resolve an age of 92.9 ± 0.6 Ma. This age is considered the best result because the isochron age, 93.0 ± 1.8 Ma, has an unsatisfactory 40Ar/36Ar value for atmospheric Ar (129 ± 652).
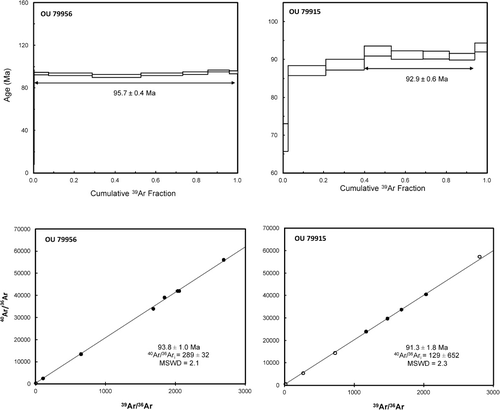
Although the biotite-bearing orthogneisses are cross-cut by lamprophyre and basaltic dikes, in situ samples were extensively altered and so a fresh lamprophyre specimen found as a stream boulder in the centre of the range was analysed. A kaersutite phenocryst from this lamprophyre (OU 79943) yielded a total gas age of 69.8 ± 2.9 Ma. Of the 39Ar, 99.6% was released in the final four heating steps, which also meet the criteria for a plateau at 69.4 ± 2.4 Ma (). As the errors for isochron ages are high, no meaningful isochron age could be constructed. The most likely intrusion age is 69 Ma.
Fraser Complex
The Fraser Complex is an elongated group of deformed rocks sandwiched between the Alpine Fault and the Fraser Fault. The complex is composed of amphibolite–facies orthogneiss and paragneiss (Rattenbury Citation1991). The protolith may represent a metamorphosed equivalent of regional granitic and gneissic basement to Greenland Group sediments (Rattenbury Citation1991). More recently it has been suggested to represent metamorphosed Buller Terrane sedimentary rocks and intrusives, including 110 Ma Rahu Suite granitoids as the youngest unit identified within the protolith (Hiess et al. Citation2010). Equivalent but typically less-deformed lithologies to the Fraser Complex are found on Granite Hill east of Lake Brunner (Waight et al. Citation1997; ). The Fraser Complex was metamorphosed at kyanite grade (Rattenbury Citation1991) in the Cretaceous (Hiess et al. Citation2010), and was subsequently mylonitized. K/Ar dating of biotite (Rattenbury Citation1987) suggests cooling through the biotite closure temperature (c. 450 °C, Villa Citation1998) at c. 44–61 Ma. Basaltic, lamprophyric and trachyte dikes in the Fraser Complex were deformed during mylonitization.
Because the lamprophyre dikes in the Fraser Complex are mylonitized and close to the active Alpine Fault, any determined 40Ar/39Ar ages could yield the age of cooling after deformation or be significantly disturbed rather than providing the timing of dike emplacement. Therefore, one trachyte dike (OU 55684) was selected for U–Th–Pb zircon geochronology. The selected sample was collected from the Tuke River and has been described by Rattenbury (Citation1991). A total of 31 analyses were carried out on prismatic zircon grains from the trachyte by LA-ICP-MS (see ). Some of the apparently youngest ages come from inclusion-rich zones in zircon grains. Qualitative electron microprobe investigations of these inclusions using energy dispersive spectrometry indicates that they comprise Th-rich phases [likely thorite (Th,U)SiO4, see ] and another silicate phase, probably biotite (too small to analyse but qualitative major element abundances suggest a biotite-like composition). Many analyses (including those in the thorite-bearing domains) were either discordant or had internal MSWD values of > 10; these data are excluded from any further age calculation. The remaining analyses yield 208Pb-corrected ages between 1241 and 63 Ma, with the older ages clearly inherited from the source region or contamination during ascent. The youngest six grains, which are from subhedral crystals that are larger than the older (inherited grains), yield a pooled age of 68.4 ± 3.5 Ma with an MSWD of 3.9. The high MSWD indicates that this data set could incorporate more than one population, but with such a small dataset, it is not possible to meaningfully refine this age. We interpret this to indicate that the emplacement of this trachyte dike occurred between 65 and 72 Ma (see ). Although this age is imperfect, we note that it matches the age range of the two existing trachyte ages from Westland. A summary of the new geochronological results from this study is presented in .
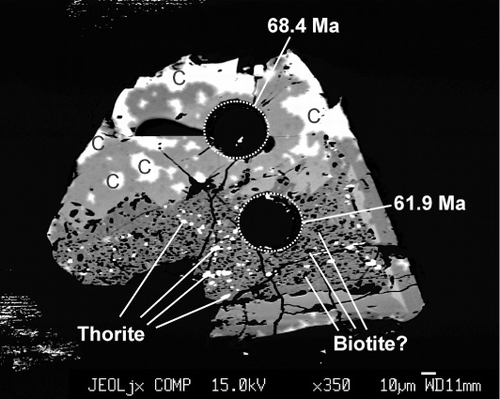
Table 4 Full LA-ICP-MS results for sample OU55684.
Table 5 Summary of new age results and their locations.
Discussion
The new geochronological data extend the known range of dike magmatism in Westland and shows that at c. 68 Ma magmatism was bimodal with mafic as well as trachytic dikes being emplaced. Although not very voluminous, post-110 Ma magmatism preceding Gondwana break-up is widely distributed through central (Adams & Nathan Citation1978; Tulloch Citation1979; White Citation1987; Rattenbury Citation1987, Citation1991; Tulloch & Kimbrough Citation1989; Waight et al. Citation1998b; Jongens Citation2006) and north Westland (Hunt & Nathan Citation1976). Additionally, post-110 Ma felsic (e.g. Phillips et al. Citation2005; Tulloch et al. Citation2009) and mafic (e.g. Nathan Citation1978; Randall Citation2004; Timm et al. Citation2010; Ryland Citation2011) magmatic rocks occur sporadically throughout south Westland. Geochronological studies of central-north Westland, including the newly presented data, are summarized from south to north in .
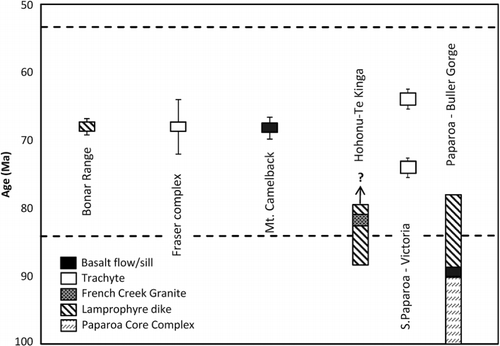
Deformation and magmatism in the Fraser Complex
Although imprecise, the interpreted 68 ± 4 Ma intrusion age of the deformed trachyte dike in the Fraser Complex overlaps with ages of mafic, as well as trachytic, magmatism outside the complex, indicating coupled magmatic and regional stress relations. Additionally, the age demonstrates that mylonitization of this Westland unit cannot be exclusively related to Early Cretaceous core complex formation (cf. Kimbrough et al. Citation1994) and is therefore likely to be related to the adjacent Alpine Fault, as speculated by Rattenbury (Citation1987, Citation1991). Combined with the biotite K/Ar cooling ages of 44–61 Ma (Rattenbury Citation1987) this would constrain the ductile mylonitization that affected the trachyte dike to have occurred between an interval with 72 and 44 Ma as the oldest and youngest possible ages, respectively. However, this age range precedes the onset of the Alpine Fault at 30–25 Ma (Cooper et al. Citation1987; Schellart et al. Citation2006; Furlong & Kamp Citation2009). Therefore, the ages in the Fraser Complex would imply that a previously unidentified stage of deformation between 72 and 44 Ma is recorded within the Fraser Complex that has no equivalent elsewhere in the Western Province. A better explanation is that the biotite cooling ages of 44–61 Ma are inaccurate and too old. This could be due to total gas ages representing a mixture of different age domains within the analysed mineral, as shown in detail for rocks adjacent to the Alpine Fault by Batt et al. (Citation2004).
Intrusion of dikes in the Bonar Range
The biotite plateau and isochron ages of 93–96 Ma for the Bonar Range orthogneiss represent a cooling of the rock below c. 450 °C, the suggested closure temperature for K/Ar in biotite (Villa Citation1998). This age does not appear to be reset by the intrusion of c. 69 Ma dikes or later activity related to the Alpine Fault. Rather, it coincides with cooling ages of the lower plate of the Paparoa metamorphic core complex to the north (Spell et al. Citation2000). Based on zircon fission-track analyses Ring & Bernet (Citation2010) have argued that in contrast to coeval core complex formation, cooling of the Bonar Range was slow and controlled by erosional denudation. Some of the zircon fission-track cooling ages from the Bonar Range, however, are older than the newly acquired biotite ages from the Bonar Orthogneiss by up to c. 30 Ma. These data fail to describe a plausible cooling history because the annealing temperature of fission tracks in zircon (c. 280 °C, Bernet Citation2009) is lower than the K/Ar closure age in biotite (c. 450 °C, Villa Citation1998). Therefore the actual uplift history of the Bonar Range must be more complex, but it is beyond the scope of this article to discuss this in detail.
Because the Ar closure temperature for amphibole (c. 600 °C, Kamber et al. Citation1995) is higher than that of biotite, the older biotite age of the country rock confirms that the lamprophyre kaersutite age of c. 69 Ma represents the lamprophyre dike intrusion age and that it has not been reset by metamorphism.
Regional age correlations
Both lamprophyre dike locations dated in the Hohonu Range have similar ages of c. 88 Ma. One of the previously reported ages, K/Ar in hornblende of 92 ± 5 Ma (GA3464, Wellman & Cooper Citation1971), obtained from a dike along the northern slope of Mt Turiwhate (see ) overlaps with the new age data and possibly reflects an accurate (albeit imprecise) intrusion age. Because field relationships indicate that dike intrusion was also contemporaneous with and post-dates the c. 82 Ma French Creek Granite, the new ages of 88 Ma indicate that the intrusion of dikes in the Hohonu Range lasted at least 6 Ma (from 88 to 82 Ma) and continued for an unknown period. The intrusion ages of lamprophyre dikes in the Hohonu Range therefore predate and overlap with the beginning of Tasman Sea spreading. This temporal relationship is also observed in basalts and lamprophyre dikes dated at 80–92 Ma (Adams & Nathan Citation1978) intruding Pororari Group sedimentary rocks in the Buller Gorge, directly north of the Paparoa metamorphic core complex. These ages indicate that mafic magmatism closely followed the cessation of rapid uplift of the core complex (Spell et al. Citation2000) that was later cut by trachyte dikes dated at c. 64 Ma with possible equivalents dated at c. 74 Ma in the Victoria Ranges to the east of the Paparoa Core Complex (Tulloch & Kimbrough Citation1989).
Bonar Range lamprophyre kaersutite 40Ar/39Ar (69 Ma) and Fraser Complex zircon U–Pb ages (68 ± 4 Ma) overlap with the previously dated (whole rock K/Ar) basalts at Mt Camelback and the base of the Arahura-1 drillcore (both c. 68 Ma, Sewell et al. Citation1988), situated between the Bonar and Hohonu Ranges and to the west of the Fraser Complex (see ). The restricted spread in age of the c. 69 Ma lamprophyre, basalts and trachyte found in central Westland differs from the wide spread in generally older ages (80–92 Ma) found in mafic dikes intruding Pororari Group sediments and Rahu Suite granitoids in the Paparoa Ranges (Adams & Nathan Citation1978; Tulloch & Kimbrough Citation1989). The occurrence of Pororari Group sediments on and close to Mt Camelback (Gage & Wellman Citation1944) indicates a similar age of the onset of extension. Therefore the age of c. 69 Ma may represent an interval of local mafic magmatism in central Westland during the Tasman Sea rifting stage, post-dating the lamprophyres associated with initial break-up. Alternatively, the young ages might represent the later stages of a continuous period of mafic magmatism related to extensional tectonics. To resolve these questions, additional absolute dating, geochemistry and isotopic analysis of a larger set of lamprophyre dikes from across Westland is in progress.
Significance of c. 68 Ma magmatism
Dike emplacement at (88–82 Ma) is clearly related to regional tension that ultimately led to continental break-up (e.g. Waight et al. Citation1998b). A cause for the c. 68 Ma dikes and basaltic magmatism in Westland seems less obvious. Paleogeographic reconstructions at 68 Ma place the Western Province adjacent to the offshore Campbell Plateau, south of the South Island (e.g. Sutherland 1999). Petroleum wells and stratigraphic sections on Campbell Island indicate a sharp increase in subsidence rate for the Campbell Plateau at 68 Ma (Cook et al. 1999). This has been explained by the migration of the plateau away from low density mantle now beneath Eastern Antarctica (Sutherland et al. Citation2010). The c. 69 Ma dikes in the Western Province may also be related to this tectonic change.
Conclusions
New 40Ar/39Ar ages indicate that Gondwana break-up-related magmatism in the Western Province was mostly concentrated at c. 88 Ma and at c. 68 Ma. Limited data so far suggest two discrete pulses of magmatism but a continuous period of magmatism cannot be ruled out.
The emplacement of dikes in Westland at c. 69 Ma coincides with tectonic changes on the offshore Campbell plateau.
U–Pb zircon dating of a deformed trachyte dike in the Fraser Complex supports the interpretation that deformation of the Fraser Complex occurred in the Cenozoic. Deformation is likely coupled to activity of the Alpine Fault.
Biotite 40Ar/39Ar dating of Bonar Range gneisses appears to conflict with previous zircon fission-track thermochronology.
Acknowledgements
Two anonymous reviewers are thanked for their review of the manuscript. Matthew Leybourne is thanked for comments and efficient and involved editorial handling. Andy Tulloch is thanked for constructive discussion and help in constructing . Joseph Kula is thanked for assistance in data interpretation. JMS acknowledges financial support from FRST contract UOX1004. The Danish Research Council for Nature and Universe is acknowledged for their support to TEW and QHAVDM (grant no. 10-085245).
References
- Adams CJ, Nathan S 1978. Cretaceous chronology of the lower Buller Valley, South Island, New Zealand. New Zealand Journal of Geology and Geophysics 18: 39–48. 10.1080/00288306.1975.10426345
- Batt GE, Baldwin SL, Cottam MA, Fitzgerald PG, Brandon MT, Spell TL 2004. Cenozoic plate boundary evolution in the South Island of New Zealand: New thermochronological constraints. Tectonics 23: TC4001. 10.1029/2003TC001527
- Bradshaw JD 1989. Cretaceous geotectonic patterns in the New Zealand region. Tectonics 8: 803–820. 10.1029/TC008i004p00803
- Beggs JM, Ghisetti FC, Tulloch AJ 2008. Basin and petroleum systems analysis of the West Coast region, South Island, New Zealand. In: Bevin JE, Bradshaw BE, Uruski C eds. Eastern Australasian Basins Symposium, Volume III. Sydney, PESA. Pp. 341–348.
- Bernet M 2009. A field-based estimate of the zircon fission-track closure temperature. Chemical Geology 259: 181–189. 10.1016/j.chemgeo.2008.10.043
- Bishop DJ 1992. Extensional tectonism and magmatism during the middle Cretaceous to Paleocene, North Westland, New Zealand. New Zealand Journal of Geology and Geophysics 35: 81–91. 10.1080/00288306.1992.9514502
- Compston W, Williams IS, Meyer C 1984. U-Pb geochronology of zircons from lunar breccia 73217 using a sensitive high mass-resolution ion microprobe. Journal of Geophysical Research 89: B524–B525. 10.1029/JB089iS02p0B525
- Cook RA, Sutherland R, Zhu H 1999. Cretaceous–Cenozoic geology and petroleum systems of the Great South Basin, New Zealand. Lower Hutt, New Zealand, Institute of Geological and Nuclear Sciences Monograph 20.
- Cooper AF, Barreiro BA, Kimbrough DL, Mattinson JM 1987. Lamprophyre dike intrusion and the age of the Alpine Fault, New Zealand. Geology 15: 941–944. 10.1130/0091-7613(1987)15%3C941:LDIATA%3E2.0.CO;2
- Furlong KP, Kamp PJJ 2009. The lithospheric geodynamics of plate boundary transpression in New Zealand: initiating and emplacing subduction along the Hikurangi margin, and the tectonic evolution of the Alpine Fault system. Tectonophysics 474: 449–462. 10.1016/j.tecto.2009.04.023
- Gage M, Wellman HW 1944. The geology of Koiterangi Hill, Westland. Transactions of the Royal Society of New Zealand 73: 351–364.
- Gaina C, Müller RD, Roest WR, Symonds P 1998. The tectonic history of the Tasman Sea: a puzzle with 13 pieces. A gravity anomaly animation. Earth Interactions 2: 1–23. 10.1175/1087-3562(1998)002%3C0001:TOOTTS%3E2.3.CO;2
- Grindley GW, Oliver PJ 1979. Palaeomagnetism of upper cretaceous dykes, Buller Gorge, North Westland, in relation to the bending of the New Zealand orocline. Bulletin of the Royal Society of New Zealand 18: 131–147.
- Hermansson T, Stephens MB, Page LM 2008. 40Ar/39Ar hornblende geochronology from the Forsmark area in central Sweden: constraints on late Svecofennian cooling, ductile deformation and exhumation. Precambrian Research 167: 303–315. 10.1016/j.precamres.2008.09.003
- Hiess J, Ireland T, Rattenbury MS 2010. U-Th-Pb zircon and monazite geochronology of Western province gneissic rocks, central-south Westland, New Zealand. New Zealand Journal of Geology and Geophysics 53: 241–269. 10.1080/00288306.2010.495450
- Hunt T, Nathan S 1976. Inangahua magnetic anomaly, New Zealand. New Zealand Journal of Geology and Geophysics 19: 395–406. 10.1080/00288306.1976.10423536
- Jongens R 2006. Gneissic rocks of the Bonar Range, central Westland, New Zealand. New Zealand Journal of Geology & Geophysics 49: 281–286. 10.1080/00288306.2006.9515166
- Kamber BS, Blenkinsop TG, Villa IM, Dahl PS 1995. Proterozoic transpressive deformation of the Northern Marginal Zone, Limpopo Belt, Zimbabwe. The Journal of Geology 103: 493–508. 10.1086/629772
- Kimbrough DL, Tulloch AJ 1989. Early Cretaceous age of orthogneiss from the Charleston Metamorphic Group, New Zealand. Earth and Planetary Science Letters 95: 130–140.
- Kimbrough DL, Tulloch AJ, Rattenbury MS 1994. Late Jurassic–Early cretaceous metamorphic age of Fraser Complex migmatite, Westland, New Zealand. New Zealand Journal of Geology and Geophysics 37: 137–142. 10.1080/00288306.1994.9514608
- Laird MG, Bradshaw JD 2004. The break-up of a long-term relationship: the cretaceous separation of New Zealand from Gondwana. Gondwana Research 7: 273–286. 10.1016/S1342-937X(05)70325-7
- Muir RJ, Ireland TR, Weaver SD, Bradshaw JD, Waight TE, Jongens R, et al. 1997. SHRIMP U–Pb geochronology of Cretaceous magmatism in northwest Nelson–Westland, South Island, New Zealand. New Zealand Journal of Geology and Geophysics 40: 453–463. 10.1080/00288306.1997.9514775
- Nathan S 1977. Cretaceous and lower tertiary stratigraphy of the coastal strip between buttress point and ship creek, south Westland, New Zealand. New Zealand Journal of Geology and Geophysics 20: 615–654. 10.1080/00288306.1977.10430725
- Nathan S 1978. Upper Cenozoic stratigraphy of South Westland, New Zealand. New Zealand Journal of Geology and Geophysics 21: 329–361. 10.1080/00288306.1978.10424061
- Nathan S, Rattenbury MS, Suggate RP ( compilers) 2002. Geology of the Greymouth area: scale 1:250,000. Institute of Geological & Nuclear Sciences 1:250,000 geological map 12. Lower Hutt, Institute of Geological & Nuclear Sciences. 1 folded map + 58 p.
- Phillips CJ, Cooper AF, Palin JM, Nathan S 2005. Geochronological constraints on Cretaceous–Paleocene volcanism in South Westland, New Zealand. New Zealand Journal of Geology and Geophysics 48: 1–14. 10.1080/00288306.2005.9515094
- Randall SB 2004. Granite Intrusions & Greenland Group Metasediments from the Haast Region, South Westland. Unpublished BSc honours thesis lodged at the Library. Dunedin, University of Otago.
- Rattenbury MS 1987. Timing of mylonitisation west of the Alpine Fault, central Westland, New Zealand. New Zealand Journal of Geology and Geophysics 30: 287–297. 10.1080/00288306.1987.10552623
- Rattenbury MS 1991. The Fraser Complex: high–grade metamorphic, igneous and mylonitic rocks in central Westland, New Zealand. New Zealand Journal of Geology and Geophysics 34: 23–33. 10.1080/00288306.1991.9514436
- Rock NMS 1987. The nature and origin of lamprophyres: an overview. Geological Society, London, Special Publications 30: 191–226.
- Renne PR, Swisher CC, Deino AL, Karner DB, Owens TL, DePaolo DJ 1998. Intercalibration of standards, absolute ages and uncertainties in 40Ar/39Ar dating. Chemical Geology 145: 117–152. 10.1016/S0009-2541(97)00159-9
- Ring U, Bernet M 2010. Fission-track analysis unravels the denudation history of the Bonar Range in the footwall of the Alpine Fault, South Island, New Zealand. Geological Magazine 147: 801–813. 10.1017/S0016756810000208
- Ryland CB 2011. The geology and structural evolution of the bald Hill-Maori saddle region, South Westland, New Zealand. Unpublished MSc thesis lodged at the Library. Dunedin, University of Otago.
- Sagar MW, Palin JM 2011. Emplacement, metamorphism, deformation and affiliation of mid-Cretaceous orthogneiss from the Paparoa Metamorphic Core Complex lower-plate, Charleston, New Zealand. New Zealand Journal of Geology and Geophysics 54: 273–289.
- Schellart WP, Lister GS, Toy VG 2006. A late cretaceous and cenozoic reconstruction of the Southwest Pacific region: tectonics controlled by subduction and slab rollback processes. Earth-Science Reviews 76: 191–233. 10.1016/j.earscirev.2006.01.002
- Scott JM, Palin JM 2008. LA–ICP–MS U–Pb zircon ages from Mesozoic plutonic rocks in eastern Fiordland, New Zealand. New Zealand Journal of Geology and Geophysics 51: 105–113. 10.1080/00288300809509853
- Scott JM, Muhling J, Fletcher I, Billia M, Palin JM, Elliot T, Günter C 2011a. The relationship of Palaeozoic metamorphism and S-type magmatism on the paleo-Pacific Gondwana margin. Lithos 127: 522–534. 10.1016/j.lithos.2011.09.008
- Scott JM, Cooper AF, Tulloch AJ, Spell TL 2011b. Crustal thickening of the early cretaceous paleo-Pacific Gondwana margin. Gondwana Research 20: 380–394. 10.1016/j.gr.2010.10.008
- Sewell RJ, Nathan S, Adams CJ 1988. Geochemistry of late cretaceous basaltic rocks from North Westland. New Zealand Geological Survey Record 35: 113–121.
- Spell TL, McDougall I, Tulloch AJ 2000. Thermochronologic constraints on the breakup of the Pacific Gondwana margin: the Paparoa metamorphic core complex, South Island, New Zealand. Tectonics 19: 433–451. 10.1029/1999TC900046
- Steiger RH, Jäger E 1977. Subcommission on geochronology: convention on the use of decay constants in geochronology and cosmochronology. Earth and Planetary Science Letters 36: 359–362. 10.1016/0012-821X(77)90060-7
- Suggate RP, Waight TE 1999. Geology of the Kumara-Moana area. Institute of Geological and Nuclear Sciences Map 24. Lower Hutt, New Zealand, Institute of Geological and Nuclear Sciences Ltd.
- Sutherland R 1999. Basement geology and tectonic development of the greater New Zealand region: an interpretation from regional magnetic data. Tectonophysics 308: 341–362.
- Sutherland R, Spasojevic S, Gurnis M 2010. Mantle upwelling after Gondwana subduction death explains anomalous topography and subsidence histories of eastern New Zealand and West Antarctica. Geology 38: 155–158. 10.1130/G30613.1
- Timm C, Hoernle K, Werner R, Hauff F, Van den Bogaard P, White J, et al. 2010. Temporal and geochemical evolution of the Cenozoic intraplate volcanism of Zealandia. Earth-Science Reviews 98: 38–64. 10.1016/j.earscirev.2009.10.002
- Tulloch AJ 1979. Plutonic and metamorphic rocks of the Victoria Range segment of the Karamea Batholith, southwest Nelson, New Zealand. Unpublished PhD thesis. Dunedin, University of Otago.
- Tulloch AJ, Kimbrough DL 1989. The Paparoa metamorphic core complex, New Zealand: cretaceous extension associated with fragmeotation of the pacific margin of Gondwana. Tectonics 8: 1217–1234. 10.1029/TC008i006p01217
- Tulloch AJ, Nathan S 1990. Spinel harzburgite xenoliths in alkali basalt and camptonite from North Westland and southeast Nelson, New Zealand. New Zealand Journal of Geology and Geophysics 33: 529–534. 10.1080/00288306.1990.10425679
- Tulloch AJ, Kimbrough DL, Waight TE 1994. The French creek granite, North Westland, New Zealand—Late Cretaceous A-type plutonism on the Tasman passive margin. In: van der Lingen GJ, Swanson KM, Muir RJ eds. Evolution of the Tasman Sea Basin: Proceedings of the Tasman Sea Conference, Christchurch, New Zealand, 27–30 November 1992. A. A. Balkema, Rotterdam. Pp. 65–67.
- Tulloch AJ, Ramezani J, Mortimer N, Mortensen J, van den Bogaard P, Maas R 2009. Cretaceous felsic volcanism in New Zealand and Lord Howe Rise (Zealandia) as a precursor to final Gondwana break-up. Geological Society, London, Special Publications 321: 89–118.
- Uto K, Ishizuka O, Matsumoto A, Kamioka H, Togashi S 1997. Laser-heating 40Ar/39Ar dating system of the Geological Survey of Japan: system outline and preliminary results. Bulletin of the Geological Survey of Japan 48: 23–46.
- Veevers JJ, McArthur C, Roots SR 1991. Review of seafloor spreading around Australia. I. synthesis of the patterns of spreading. Australian Journal of Earth Sciences 38: 373–389. 10.1080/08120099108727979
- Villa IM 1998. Isotopic closure. Terra Nova 10: 42–47. 10.1046/j.1365-3121.1998.00156.x
- Waight TE 1995. The geology and geochemistry of the Hohonu Batholith and adjacent rocks, North Westland, New Zealand. Unpublished PhD thesis. Christchurch, University of Canterbury.
- Waight TE, Weaver SD, Ireland TR, Maas R, Muir RJ, Shelley D 1997. Field characteristics, petrography, and geochronology of the Hohonu Batholith and the adjacent Granite Hill complex, North Westland, New Zealand. New Zealand Journal of Geology and Geophysics 40: 1–17. 10.1080/00288306.1997.9514736
- Waight TE, Weaver SD, Muir RJ 1998a. Mid-Cretaceous granitic magmatism during the transition from subduction to extension in southern New Zealand: a chemical and tectonic synthesis. Lithos 45: 469–482. 10.1016/S0024-4937(98)00045-0
- Waight TE, Weaver SD, Maas R, Eby GN 1998b. French creek granite and Hohonu Dyke Swarm, South Island, New Zealand: late cretaceous alkaline magmatism and the opening of the Tasman Sea. Australian Journal of Earth Sciences 45: 823–835. 10.1080/08120099808728438
- Weissel JK, Hayes DE 1977. Evolution of the Tasman Sea reappraised. Earth and Planetary Science Letters 36: 77–84. 10.1016/0012-821X(77)90189-3
- Wellman P, Cooper A 1971. Potassium-argon age of some New Zealand lamprophyre dikes near the Alpine Fault. New Zealand Journal of Geology and Geophysics 14: 341–350. 10.1080/00288306.1971.10421930
- Wendt I, Carl C 1991. The statistical distribution of the mean squared weighted deviation. Chemical Geology Isotope Geoscience Section 86: 275–285. 10.1016/0168-9622(91)90010-T
- White PJ 1987. The petrology, geochemistry and petrogenesis of igneous and metamorphic rocks from the Paparoa Range, southwest Nelson. Unpublished PhD thesis. Wellington, Victoria University of Wellington.
- Wiederkehr M, Sudo M, Bousquet R, Berger A, Schmid SM 2009. Alpine orogenic evolution from subduction to collisional thermal overprint: the 40Ar/39Ar age constraints from the Valaisan Ocean, Central Alps. Tectonics 28: TC6009. 10.1029/2009TC002496