Abstract
The peak conditions and timing of metamorphism have been determined for the early Paleozoic Greenland Group in south Westland, New Zealand. The Greenland Group, which is the most extensive pre-Cenozoic unit west of the Alpine Fault in Westland, is dominated by greenschist facies greywacke and argillite. However, in the Jackson River valley this unit comprises amphibolite facies pelitic and psammitic gneisses. Psammitic horizons are dominated by biotite, plagioclase and quartz. Pelitic horizons have similar mineralogy but also contain sillimanite and K–feldspar, as well as boudinaged K–feldspar-bearing leucosomes. Conventional geothermometry and thermodynamic modelling indicate that peak metamorphism occurred between 660 and 700 °C and 3.5 and 6.0 kbar. The timing of this moderately high–T/low–P event is constrained to be mid-Paleozoic by U–Th–Pb dating of overgrowths on detrital zircon (334 ± 18 Ma) and confirmed to be Carboniferous by a single population of monazite grains (343 ± 3 Ma). Greenland Group metamorphism in the Jackson River valley may represent Carboniferous high crustal heat flow in the Gondwana margin hinterland while crustal thickening was occurring closer to the subduction zone.
Introduction
The Alpine Fault juxtaposes amphibolite facies Mesozoic Haast Schist against early Paleozoic Greenland Group in south Westland (). The metamorphic history of the Haast Schist has been extensively studied (Turner Citation1933; Mason Citation1962; Cooper Citation1972, Citation1974; Wallace Citation1974; Grapes & Watanabe Citation1992; Chamberlain et al. Citation1995; Mortimer Citation2000; Mortimer & Cooper Citation2004; Vry et al. Citation2004; Cooper & Ireland Citation2013) but comparatively little is known about the pressure–temperature–time (P–T–t) history of the adjacent Greenland Group. Reconnaissance metamorphic studies have been conducted by Turner (Citation1930, Citation1933), Mason & Taylor (Citation1987) and Mortimer et al. (Citation2013), but these inves-tigations provide only brief field observations and mineral descriptions.
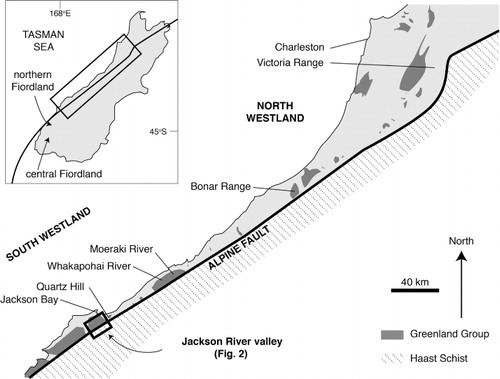
This article reports the results of a study into amphibolite facies Greenland Group immediately west of the Alpine Fault in the Jackson River valley in south Westland (–). The metamorphic conditions and relationships are evaluated through petrographic observations, conventional geothermometry, thermodynamic modelling and U–Th–Pb zircon and monazite dating. The significance of this metamorphic history is then examined in a New Zealand context through comparison of similar aged metamorphic rocks in Fiordland.
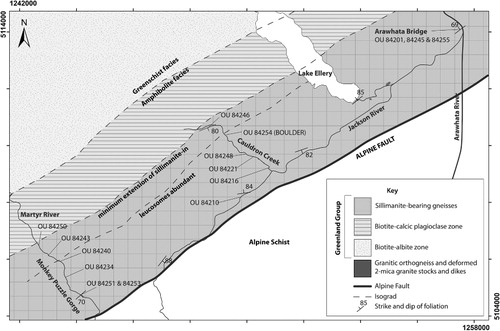
Geological setting
The Ordovician Greenland Group is part of New Zealand’s Western Province Buller Terrane. The Greenland Group is a regionally extensive unit that largely consists of metamorphosed inter-bedded greywackes and argillites (Laird Citation1972; Laird & Shelley Citation1974; Mortimer et al. Citation2013). Analysis of low-grade rocks shows the unit to be dominated by quartz, with other prominent components being feldspar and lithic fragments (Laird Citation1972). Geochemistry indicates that the Greenland Group protoliths were relatively immature passive margin sediments (Roser et al. Citation1996). Much of the unit contains a weak greenschist facies metamorphic overprint consisting of chlorite, albite, sericite and quartz (Laird & Shelly; Shelley Citation1975; Mortimer et al. Citation2013). Amphibolite facies paragneiss assemblages are uncommon, with the best-documented example being the metasedimentary component (Pecksniff Metasedimentary Gneiss) to the Charleston Metamorphic Group in north Westland (White Citation1994). Other documented amphibolite facies metasedimentary occurrences are in the Bonar Range (Jongens Citation2006; Scott et al. Citation2011), Victoria Range (Ireland & Gibson Citation1998) and parts of south Westland (Mortimer et al. Citation2013) ().
The precise timing of Greenland Group metamorphic events is not well constrained. Argillites in the low-grade rocks contain a greenschist facies assemblage that forms a prominent axial planar cleavage to macroscopic folds that have wavelengths in the order of 100 m to km (Laird & Shelly; Shelley Citation1975; Rattenbury & Stewart Citation2000). K–Ar ages from these rocks have been interpreted to indicate that metamorphism occurred at c. 440 Ma (Adams et al. Citation1975), but the inspected rocks have also been shown to contain detrital muscovite grains (Adams & Kelley Citation1998), which means that the K–Ar ages likely represent mixtures of detrital and metamorphic micas. What can be stated with confidence is that, at least in north Westland, the greenschist facies cleavage to the macroscopic folding pre-dates c. 371 Ma cross-cutting Karamea Suite granitoids (Tulloch et al. Citation2009). In the case of the amphibolite facies assemblages, monazite from Greenland Group paragneiss in the Bonar Range has yielded U–Th–Pb ages of 373.4 ± 4.1 to 358.5 ± 3.7 Ma (Scott et al. Citation2011). Monazite grains in amphibolite facies paragneiss at Charleston and in the Victoria Range have also yielded Devonian dates (Ireland & Gibson Citation1998; Hiess et al. Citation2010). However, orthogneisses within the amphibolite facies Charleston Metamorphic Group show a Cretaceous metamorphic overprint (Kimbrough & Tulloch Citation1989; Tulloch & Kimbrough Citation1989; Ireland & Gibson Citation1998; Sagar & Palin Citation2011).
Greenland Group in south Westland
Rocks of the Greenland Group in south Westland – the region of the current study – form an elongate swathe that extends from the Tasman Sea eastwards to the Alpine Fault (Mortimer et al. Citation2013) (). Chlorite zone greywackes and argillites occur at the west coast, with biotite–albite (greenschist facies), biotite–calcic plagioclase (amphibolite facies) and K–feldspar–sillimanite isograds mapped towards the Alpine Fault (Mortimer et al. Citation2013). Isotopic dates from this region are sparse and difficult to interpret. Aronson (Citation1965) reported a scattering of Carboniferous to Permian ages (all old Rb–Sr ages reported in this article have been recalculated with decay constants of Steiger & Jäger Citation1977) from a Greenland Group ‘schist’ at Moeraki River. In a subsequent study, Aronson (Citation1968) reported a Rb–Sr date of 357 ± 8 Ma from granite at Quartz Hill that intrudes the Greenland Group. The Jackson Bay area has whole-rock K–Ar dates of 432 ± 18 and 428 ± 16 Ma, and a Whakapohai River sample has a whole-rock K–Ar age of 350 ± 7 Ma (Adams et al. Citation1975). As noted above, the significance of the whole-rock K–Ar dates is difficult to assess because of the presence of detrital muscovite grains. Adams (Citation2004) reported Rb–Sr whole-rock ages of 340 ± 13 Ma from Arawhata Bridge, 458 ± 9 Ma from Jackson Bay and 412 ± 10 Ma from the Whakapohai River.
Geology of Greenland Group rocks at Jackson River
Rocks of the Greenland Group in the Jackson River valley consist of interlayered psammitic and pelitic schists and gneisses that dip subvertically and strike to the northeast (). The psammitic and pelitic rocks can be easily distinguished from one another by their mineralogy, texture and colour, and by the abundance of banded leucosome segregations and pods within the pelitic lithologies. A gneissic foliation is well developed and is prominent within the banded pelitic rocks (). Some pelitic rocks are spotted, with small pale patches composed of muscovite and/or sillimanite aligned parallel to a weak foliation (). Meta-granitoid sheets are parallel to the general fabric orientation, and continuous stretches (stocks?) of meta-granite occur in the river transects and along the Jackson River road and Monkey Puzzle Gorge (–). Undeformed lamprophyre dikes cut across foliation at the Arawhata Bridge. There are no dates on these dikes, but they are petrographically similar to lamprophyres in central–northern Westland that also cut across foliation and have yielded Cretaceous Ar–Ar ages (Van der Meer et al. Citation2013).
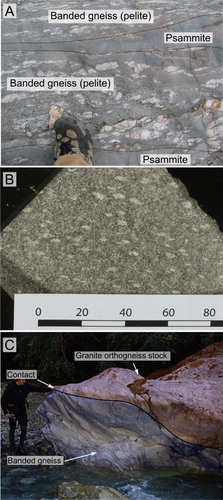
Paragneisses are dominated by biotite, feldspar and quartz with or without muscovite, sillimanite and/or K–feldspar. Accessory minerals are tourmaline, zircon, apatite, monazite, ilmenite and, in some cases, rutile. The relative abundances of these minerals vary according to the primary compositional layering that occurs on cm to m scales. Post-peak metamorphic hydration has caused some biotite to break down to green chlorite that is speckled with tiny ilmenite grains. Plagioclase displays lamellar twinning and its composition is oligoclase to andesine. Prismatic sillimanite and/or fibrolite grains can be found replacing muscovite porphyroblasts and are usually accompanied by K–feldspar. In the case of spotted paragneisses, muscovite porphyroblasts are partially replaced by ovoid knots of fibrolite (). Sillimanite is not abundant in the banded gneisses, but prismatic and fibrous grains can still be found nucleating on muscovite and biotite (, ). The prominent felsic segregations within the pelitic rocks () are composed of K–feldspar, plagioclase and quartz () with or without small quantities of muscovite, biotite, tourmaline, apatite and zircon. Plagioclase commonly shows well-developed crystal faces and stepped boundaries.
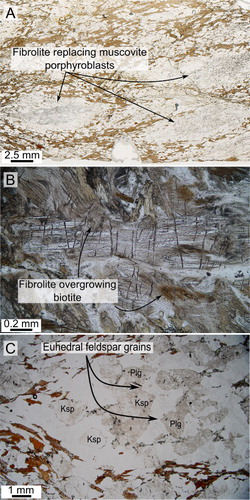
Metamorphism
Mineralogical constraints on metamorphism
The observed mineral assemblages allow preliminary assessment of the metamorphic conditions experienced by the Greenland Group in the Jackson River valley. The most common occurrence of sillimanite is within muscovite porphyroblasts (). This is commonly accompanied by formation of K–feldspar, and the association is, therefore, probably due to the dehydration reaction:
Quantitative geothermometry
In an attempt to quantify conditions of peak metamorphism, the Ti-in-biotite geothermometer of Henry et al. (Citation2005) was applied to 14 samples that contain biotite, oligoclase, quartz, ilmenite and titanite or rutile. At least 10 biotite grains were analysed per sample to cover the range of grain morphologies. The geothermometer is calibrated for Ti-saturated pelitic rocks that equilibrated under reducing conditions at P = 4–6 kbar. Jackson River Greenland Group samples contain ilmenite, rutile or titanite and therefore indicate saturation or near saturation of Ti (Ghent & Stout Citation1984; Chambers & Kohn Citation2012). Graphite is absent, but the occurrence of ilmenite instead of magnetite suggests reducing rather than oxidising conditions. Metamorphic pressure is not quantitatively constrained, but is estimated to be c. 5 kbar (see below). Representative data are summarised in and the locations of samples are shown in .
Table 1 Representative biotite analyses from the Jackson River area.
At face value, the distribution in Ti-in-biotite results could be construed as indicating temperature gradients within the mapped area. For example, the eastward transect up Monkey Puzzle Gorge yields higher temperatures closer to the Alpine Fault. However, a transect eastward down Cauldron Creek suggests that higher temperatures occur further away from the fault. In detail, these variations are less than the precision of the geothermometer, and samples from the small exposure at Arawhata Bridge span the complete temperature range inferred for the Monkey Puzzle Gorge and Cauldron Creek sections. Furthermore, the mineralogy described above indicates that these rocks reached conditions sufficient to cause partial melting, yet the Ti-in-biotite geothermometer results systematically fall below that required to initiate water-saturated partial melting. Only the highest calculated average Ti-in-biotite estimates (c. 650 ± 24 °C, ) overlap with temperatures required for formation of a water-saturated melting (c. 660–670 °C (Spear et al. Citation1999) and indicated below from the thermodynamic modelling). Note that the ± 24 °C error value is endemic to the geothermometer and is therefore a minimum estimate; a more conservative value for an averaged population might be ± 50 °C. Even so, some samples displaying partial melting textures still fall outside the temperature required for water-saturated melting. We cannot appeal to a poor electron microprobe standardisation for the systematically low temperature values because the composition of a well-known Smithsonian Ti-bearing hornblende standard was reproduced with this analytical routine. However, many of the analysed rocks show subtle signs of retrogression. Henry et al. (Citation2005) note that applying the geothermometer to chloritised biotite will result in low temperatures because this mineral excludes Ti from its structure. Although attempts were made to avoid chloritised grains, there are few samples that are devoid of chlorite and the microprobe beam may have either encountered this mineral within the activation volume area or in sub-microscopic interlayers, thus affecting the Fe/Mg ratio and Ti concentration in biotite.
In conclusion, the Ti-in-biotite results mainly appear to underestimate the thermal conditions of metamorphism that affected many of the Jackson River Greenland Group samples, with the effects of retrogression having likely affected the analysed biotite grains. Nonetheless, the freshest samples yield temperatures that just overlap with those required for minimum melting. These results () suggest that the peak thermal conditions of Jackson River Greenland were very unlikely to exceed much beyond 670 °C. If a conservative geothermometer estimate of ± 50 °C is assumed, then the maximum temperature is close to 700 °C.
Thermodynamic modelling
To further constrain the P–T conditions and determine at what temperatures partial melting should theoretically take place in a Jackson River Greenland Group sample, a pelitic whole-rock composition (collected from Cauldron Creek) was modelled using the Gibbs free energy minimisation Theriak–Domino software package (De Capitani & Petrakakis Citation2010). The whole-rock analysis composition is: SiO2 = 68.49; TiO2 = 0.66; Al2O3 = 13.96; Fe2O3 = 5.32; MnO = 0.06; MgO = 2.74; CaO = 1.86; Na2O = 1.61; K2O = 3.22; H2O = 1.20. The elements considered in the modelling routine were Na, Ca, Fe, K, Mg, Al, Si, Ti, H and O. Fe3+ was recast as Fe2+. Because P will mainly be housed in apatite, P2O5 and a portion of CaO = 1.31 × P2O5, were excluded from the modelling routine. Mn is also minimal and was excluded due to the lack of solution models for Mn-bearing minerals in the database. H was taken as the loss on ignition value, and O was calculated via charge balance. The internally consistent dataset used was the modified Thermocalc 5.5 database of Holland & Powell (Citation1998). Activity models used were biotite, garnet, orthopyroxene and melt from White et al. (Citation2007), cordierite from Holland & Powell (Citation1998), white mica from Coggon & Holland (Citation2002) and feldspar from Baldwin et al. (Citation2005).
Several important features occur in the pseudosection shown in . The observed assemblage (biotite, sillimanite, K–feldspar, plagioclase, quartz and a crystallised liquid phase) and the lack of kyanite, andalusite and cordierite constrain the peak conditions to > 660 and P > 3.3 kbar. The predicted stability field for the rock assemblage is rather large, but can be partially constrained using the Ti-in-biotite results. Because freshest Ti-in-biotite samples suggest a maximum peak temperature of c. 700 °C, the P of metamorphism would be < 6 kbar. Although this is not a strong premise for establishing an upper bound to peak metamorphic P, we do note that kyanite has not been reported from any Greenland Group sample (whereas andalusite locally has been; Turner Citation1933; Mortimer et al. Citation2013), which suggests that the prograde metamorphic path likely had a high temperature but low pressure trajectory that avoided the kyanite stability field. The theoretical thermodynamically stable assemblage for the inspected rock composition at 700 °C and 5 kbar is: quartz (47%), plagioclase (24%, andesine), biotite (24%, Mg# = 52), K–feldspar (3%), sillimanite (2%) and ilmenite (< 0.5%) with a liquidus phase, which matches the observed minerals, their proportions and their chemistries well. Melt isopleths (not shown) indicate that the degree of melting should remain low until significantly higher temperatures are reached and biotite breaks down. Assuming a crustal density of 2.7 g/cm3, a peak temperature of 700 °C and peak pressure of 5 kbar, the geothermal gradient at the time of metamorphism would have been a fairly hot 37 °C/km. If P = 4 kbar and T = 660 °C, then the geothermal gradient would have been slightly higher.
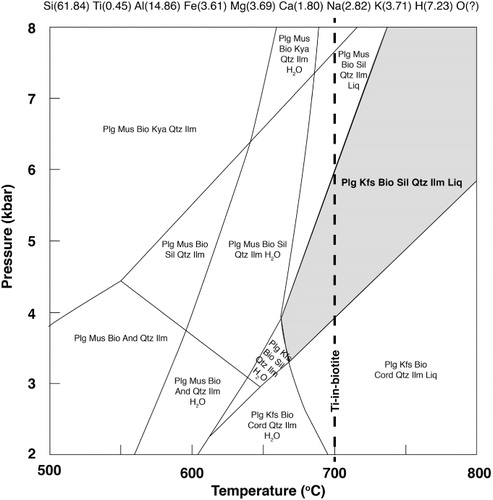
Zircon and monazite U-Th-Pb isotope dating
Methodology
Zircon grains were analysed by laser ablation inductively coupled plasma mass spectrometry (LA–ICP–MS) at the Centre for Trace Element Analysis at the University of Otago. Zircon dating followed the method described by Scott & Palin (Citation2008). Specific details related to the current study are that the LA–ICP–MS spot diameter was set at 23 µm and the data were standardised to TEMORA zircon and NIST610 silicate glass. Dates with discordance > 10% were excluded from the final pooled age calculation, along with any spots that had individual mean squared weighted deviation (MSWD) > 10. The LA–ICP–MS technique has the ability to analyse multiple spots within a zircon grain as it ‘depth profiles’; at each spot, the laser will drill down to a depth of c. 20 µm and the ablated material is analysed in real time. In a situation in which the laser drills right through a zoned grain, multiple dates can be determined from a single spot analysis (e.g. Scott et al. Citation2009a). This technique was used to date the overgrowths on zircon grains. Zircon data are summarised in SF Table 1.
Monazite grains were identified in a polished thin section using back-scattered electron imaging and energy-dispersive X-ray microanalysis (EDS) carried out on a JEOL 6400 SEM fitted with an Oxford Instruments Link Analytical X–ray detector located in the Centre for Microscopy, Characterisation and Analysis (CMCA) at The University of Western Australia. Those grains large enough (> 10 µm) for ion microprobe analysis were drilled as c. 3-mm plugs from polished thin sections and cast in a 25 mm epoxy disc. The compositions of the monazite crystals, for the purposes of matrix matching with standards, were determined by quantitative EDS with an Oxford Instruments X–Max50 detector and AZtec software, mounted on a TESCAN Vega3 SEM at CMCA. U, Th and Pb analyses were obtained with a sensitive high-resolution ion microprobe in the John de Laeter Centre for Isotope Research at Curtin University. Analytical spots on monazite were 8–10 µm, with c. 0.2 nA primary O2− ion beams. The primary standard reference material used for correction of isotopic ratios was GM2 (Kennedy & Möller Citation2012). Data were corrected for instrumental mass fractionation and over-counts on 204Pb. Data were corrected for common Pb based on measured 204Pb. Reported ages are 206Pb/238U. Data processing and age calculations were performed using Squid2 and Isoplot software of Ludwig (Citation2003). Monazite data are summarised in SF Table 2.
Results
Zircon grains were extracted from pelitic gneiss (OU84221) collected from halfway up Cauldron Creek. U–Th–Pb populations identified from the cores of grains occur at 1663 ± 80 (n = 15, MSWD = 7.0; 2σ errors on all data), 946 ± 27 (n = 17, MSWD = 8.3) and 561 ± 14 (n = 26, MSWD = 10) (SF Table 1). These detrital age populations broadly overlap with populations in other Greenland Group samples (Ireland & Gibson Citation1998) (). The Jackson River detrital zircons, however, have thin overgrowths that truncate the internal zoning (). Three successfully dated overgrowths yielded a pooled age of 334 ± 18 Ma. The overgrowths have distinctly lower Th/U ratios (< 0.1) than the cores, as is typical for metamorphic zircon (Hoskin & Schaltegger Citation2003).
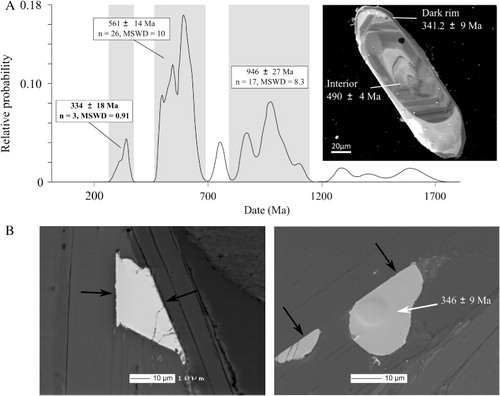
Monazite grains were located within a sample of pelitic gneiss from Arawhata Bridge (OU84201). Some monazite grains show straight grain boundaries that are almost always parallel to the cleavage in biotite, with some grains having grown along biotite cleavage planes (). Nineteen analyses (SF Table 2) were made on 12 grains. One analysis has > 2% common 206Pb due to overlap of the analysis spot with the matrix and is not included in the pooled age. The remaining 18 analyses give a weighted mean 206Pb/238U age of 343.0 ± 3.3 Ma (MSWD = 1.4, 2σ error).
Discussion
P–T–t history of Jackson River Greenland Group
The Greenland Group in the Jackson River valley experienced metamorphism at moderate to high T (c. 660–700 °C) and moderate to low P (c. 3.5–6 kbar) in the Early Carboniferous (343.0 ± 3.3 Ma). Metamorphism was accompanied by partial melting of pelitic horizons, but not psammitic horizons. Monazite textural relationships suggest that the Carboniferous metamorphism was accompanied by imposition of a penetrative foliation (). Even in samples that have a spotted appearance (), the spots are preferentially aligned parallel to the host rock foliation. Therefore, we rule out the moderately high T at moderately low P conditions as due to contact metamorphism in the aureole of a pluton. The temperature of metamorphism appears to have remained too low for the partial melts to have been efficiently extracted from the rocks; however, S–type granites were emplaced into other the parts of the Western Province at the same time (Tulloch et al. Citation2009), which means that crustal melting and efficient melt extraction was likely occurring at greater depths.
A method of achieving high heat flow and associated deformation in the shallow crust is to bring a heat source, such as the upper mantle, close to the surface of the earth during an orogenic episode. Any fabrics formed during deformation at this time will therefore form under relatively high geothermal gradient. This is a process that commonly occurs during thinning of the crust in extensional tectonic regimes (Lister & Davis Citation1989). In a Westland context, Tulloch et al. (Citation2009) suggested, on geochemical grounds, that emplacement of Devonian–Carboniferous plutons was related to regional crustal extension. Deformation accompanying thinning of the lithosphere was also proposed to explain middle Paleozoic moderate to high T/low P Devonian–Carboniferous metamorphic assemblages in the Bonar Range in central Westland (Scott et al. Citation2011). Although some of the high Carboniferous heat budget may have been complemented by mafic magmatism (Turnbull et al. Citation2013), such magmatism appears to have been of small volume and a regional extension-related elevated geotherm appears to be a reasonable explanation for the metamorphic assemblages.
Implications for the Gondwana margin
Early Carboniferous metamorphism in the Western Province is not restricted to Westland. It has also affected parts of central Fiordland () (Ireland & Gibson Citation1998; Daczko et al. Citation2009; Scott et al. Citation2009a) and northern Fiordland (Scott et al. Citation2009b). Quantitative P–T estimates for the Fiordland Early Carboniferous metamorphic fabrics yielded values of c. 6–8 kbar and ≤ 650 °C (Ireland & Gibson Citation1998; Scott et al. Citation2009a,Citationb). Indeed, Ireland & Gibson Citation(1998) interpreted Fiordland Carboniferous metamorphic ages and kyanite-bearing assemblages to represent middle Paleozoic crustal thickening. To explain the apparent tectonic discrepancies between Fiordland (inferred crustal thickening) and Westland (inferred crustal thinning) despite their similar ages of metamorphism, these blocks need to be restored to their pre-Alpine Fault positions (). This reconstruction shows that Jackson River Greenland Group would have lain well to the (present-day) south of Fiordland, on the westernmost flank of the Campbell Plateau. The elevated geothermal regime inferred from the amphibolite facies Greenland Group and Paleozoic plutonism in Westland might, therefore, represent processes that occurred in the back-arc of the middle Paleozoic convergent Gondwana margin, with the Fiordland block lying closer to the subduction zone and hence experiencing contemporaneous crustal thickening. Future work should aim to further constrain the P–T–t histories of other Western Province Paleozoic paragneisses to test this hypothesis.
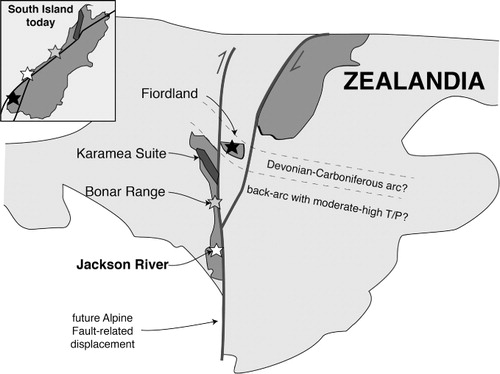
Conclusions
This study places P–T–t constraints on amphibolite facies Greenland Group in south Westland. In the Jackson River valley, rocks of the Greenland Group underwent metamorphism at amphibolite facies conditions (between c. 660 and 700 °C and c. 3.5 and 6 kbar) in the Early Carboniferous (c. 343 Ma). The conditions were suitable for partial melting of pelitic horizons, and prominent foliation–parallel leucosomes represent trapped in-situ crustal partial melts. The moderate–high T/P regime and associated deformation is interpreted to relate to an elevated Carboniferous regional geotherm, perhaps due to lithosphere thinning during extension.
Supplementary files
Supplementary file 1: Table S1. U–Th–Pb zircon data from paragneiss from Cauldron Creek (OU84221).
Supplementary file 2: Table S2. U–Th–Pb monazite data from paragneiss from Arawhata Bridge (OU84201).
Table S2. U-Th-Pb monazite data from paragneiss from Arawhata Bridge (OU84201).
Download MS Word (15.6 KB)Table S1. U-Th-Pb zircon data from paragneiss from Cauldron Creek (OU84221).
Download MS Word (31.1 KB)Acknowledgements
We gratefully acknowledge an AUSIMM New Zealand Branch Education Endowment Trust Scholarship (to MCP) for supporting the costs of monazite and zircon dating, the Department of Geology Benson Fund for supporting the costs of fieldwork, Michael Palin for calibrating the laser and helping interpret the zircon analyses, and Alan Cooper for comments on a draft. Journal review comments by Julie Vry, Nick Mortimer, and an anonymous person improved the work. We acknowledge the facilities of the Australian Microscopy & Microanalysis Research Facility at the Centre for Microscopy, Characterisation & Analysis at the University of Western Australia, a facility funded by the University, State and Commonwealth Governments. Monazite U–Th–Pb analyses were conducted using the SHRIMP ion microprobes of the John de Laeter Centre at Curtin University, Perth, Australia.
References
- Adams CJ 2004. Rb-Sr age and strontium isotope characteristics of the Greenland Group, Buller Terrane, New Zealand, and correlations at the East Gondwanaland margin. New Zealand Journal of Geology and Geophysics 47: 189–200.
- Adams CJ, Harper CT, Laird MG 1975. K-Ar ages of low grade metasediments of the Greenland and Waiuta Groups in Westland and Buller, New Zealand. New Zealand Journal of Geology and Geophysics 18: 39–48.
- Adams CJ, Kelley S 1998. Provenance of Permian-Triassic and Ordovician metagraywacke terranes in New Zealand: Evidence from 40Ar/39Ar dating of detrital micas. Geological Society of America Bulletin 110: 422–432.
- Aronson JL 1965. Reconnaissance rubidium—strontium geochronology of New Zealand plutonic and metamorphic rocks. New Zealand Journal of Geology and Geophysics 8: 401–423.
- Aronson JL 1968. Regional geochronology of New Zealand. Geochimica et Cosmochimica Acta 32: 669–697.
- Baldwin JA, Powell R, Brown M, Moraes R, Fuck RA 2005. Modelling of mineral equilibria in ultra high-temperature metamorphic rocks from the Anápolis–Itauçu Complex, central Brazil. Journal of Metamorphic Geology 23: 511–531.
- Carmichael DM 1969. On the mechanism of prograde metamorphic reactions in quartz-bearing pelitic rocks. Contributions to Mineralogy and Petrology 20: 244–267.
- Chamberlain CP, Zeitler PK, Cooper AF 1995. Geochronologic constraints of the uplift and metamorphism along the Alpine Fault, South Island, New Zealand. New Zealand Journal of Geology and Geophysics 38: 515–523.
- Chambers JA, Kohn MJ 2012. Titanium in muscovite, biotite, and hornblende: modelling, thermometry and rutile activities of metapelites and amphibolites. American Mineralogist 97: 543–555.
- Chinner GA 1961. The origin of sillimanite in Glen Clova, Angus. Journal of Petrology 2: 312–323.
- Coggon R, Holland TJB 2002. Mixing properties of phengitic micas and revised garnet–phengite thermobarometers. Journal of Metamorphic Geology 20: 683–696.
- Cooper AF 1972. Progressive metamorphism of metabasic rocks from the Haast Schist Group of southern New Zealand. Journal of Petrology 13: 457–492.
- Cooper AF 1974. Multiphase deformation and its relationship to metamorphic crystallisation at Haast River, South Westland, New Zealand. New Zealand Journal of Geology and Geophysics 17: 855–880.
- Cooper AF, Ireland TR 2013. Cretaceous sedimentation and metamorphism of the western Alpine Schist protoliths associated with the Pounamu Ultramafic Belt, Westland, New Zealand. New Zealand Journal of Geology and Geophysics 56: 188–199.
- Daczko NR, Milan LA, Halpin JA 2009. Metastable persistence of pelitic metamorphic assemblages at the root of a Cretaceous magmatic arc–Fiordland, New Zealand. Journal of Metamorphic Geology 27: 233–247.
- De Capitani C, Petrakakis K 2010. The computation of equilibrium assemblage diagrams with Theriak/Domino software. American Mineralogist 95: 1006–1016.
- Foster CT 1991. The role of biotite as a catalyst in reaction mechanisms that form sillimanite. The Canadian Mineralogist 29: 943–963.
- Ghent ED, Stout MZ 1984. TiO2 activity in metamorphosed pelitic and basic rocks; principles and applications to metamorphism in southeastern Canadian Cordillera. Contributions to Mineralogy and Petrology 86: 248–255.
- Grapes R, Watanabe T 1992. Metamorphism and uplift of Alpine Schist in the Franz Josef–Fox Glacier area of the Southern Alps, New Zealand. Journal of Metamorphic Geology 10: 171–180.
- Henry DJ, Guidotti CV, Thomson JA 2005. The Ti-saturation surface for low-to-medium pressure metapelitic biotites: implications for geothermometry and Ti-substitution mechanisms. American Mineralogist 90: 316–328.
- Hiess J, Ireland T, Rattenbury M 2010. U-Th-Pb zircon and monazite geochronology of Western Province gneissic rocks, central-south Westland, New Zealand. New Zealand Journal of Geology and Geophysics 53: 241–269.
- Holland TJB, Powell R 1998. An internally consistent thermodynamic data set for phases of petrological interest. Journal of Metamorphic Geology 16: 309–343.
- Hoskin PW, Schaltegger U 2003. The composition of zircon and igneous and metamorphic petrogenesis. Reviews in Mineralogy and Geochemistry 53: 27–62.
- Ireland T, Gibson GM 1998. SHRIMP monazite and zircon geochronology of high-grade metamorphism in New Zealand. Journal of Metamorphic Geology 16: 149–167.
- Jongens R 2006. Gneissic rocks of the Bonar Range, central Westland, New Zealand. New Zealand Journal of Geology and Geophysics 49: 281–286.
- Kennedy AK, Möller A 2012. New monazite reference material for microanalysis. Microanalytical Reference Materials. Microanalysis Society Golden Colorado Abstracts May 15–17. Pp. 14–15.
- Kimbrough DL, Tulloch AJ 1989. Early Cretaceous age of orthogneiss from the Charleston Metamorphic Group, New Zealand. Earth and Planetary Science Letters 95: 130–140.
- Laird MG 1972. Sedimentology of the Greenland Group in the Paparoa Range, West Coast, South Island. New Zealand Journal of Geology and Geophysics 15: 372–393.
- Laird MG, Shelley D 1974. Sedimentation and early tectonic history of the Greenland Group, Reefton, New Zealand. New Zealand journal of Geology and Geophysics 17: 839–854.
- Lister GS, Davis GA 1989. The origin of metamorphic core complexes and detachment faults formed during Tertiary continental extension in the northern Colorado River region, USA. Journal of Structural Geology 11: 65–94.
- Ludwig KR 2003. Isoplot/Ex version 3.0. A geochronological toolkit for Microsoft Excel. Berkeley, Berkeley Geochronological Centre Special Publication.
- Mason BH 1962. Metamorphism in the Southern Alps of New Zealand. Bulletin of the American Museum of Natural History 123: 211–248.
- Mason BH, Taylor SR 1987. High-grade basement gneisses and granitoids in Westland, New Zealand. Journal of the Royal Society of New Zealand 17: 115–138.
- Mortimer N 2000. Metamorphic discontinuities in orogenic belts: example of the garnet–biotite–albite zone in the Otago Schist, New Zealand. International Journal of Earth Sciences 89: 295–306.
- Mortimer N 2014. The oroclinal bend in the South Island of New Zealand. Journal of Structural Geology 64: 32–38.
- Mortimer N, Cooper AF 2004. U–Pb and Sm–Nd ages from the Alpine Schist, New Zealand. New Zealand Journal of Geology and Geophysics 47: 21–28.
- Mortimer N, Nathan S, Jongens R, Kawachi Y, Ryland C, Cooper AF et al. 2013. Regional metamorphism of the Early Palaeozoic Greenland Group, South Westland, New Zealand. New Zealand Journal of Geology and Geophysics 56: 1–15.
- Rattenbury MS, Stewart M 2000. Structural setting of the Globe–Progress and Blackwater gold mines, Reefton goldfield, New Zealand. New Zealand Journal of Geology and Geophysics 43: 435–445.
- Roser BP, Cooper RA, Nathan S, Tulloch AJ 1996. Reconnaissance sandstone geochemistry, provenance, and tectonic setting of the lower Paleozoic terranes of the West Coast and Nelson, New Zealand. New Zealand Journal of Geology and Geophysics 39: 1–16.
- Sagar MW, Palin JM 2011. Emplacement, metamorphism, deformation and affiliation of mid-Cretaceous orthogneiss from the Paparoa Metamorphic Core Complex lower-plate, Charleston, New Zealand. New Zealand Journal of Geology and Geophysics 54: 273–289.
- Scott JM, Cooper AF, Palin JM, Tulloch AJ, Kula J, Jongens R et al. 2009a. Tracking the influence of a continental margin on growth of a magmatic arc, Fiordland, New Zealand, using thermobarometry, thermochronology, and zircon U–Pb and Hf isotopes. Tectonics 28: 15.
- Scott JM, Palin JM 2008. LA–ICP–MS U–Pb zircon ages from Mesozoic plutonic rocks in eastern Fiordland, New Zealand. New Zealand Journal of Geology and Geophysics 51: 105–113.
- Scott JM, Palin JM, Cooper AF, Fagereng Å, King RP 2009b. Polymetamorphism, zircon growth and retention of early assemblages through the dynamic evolution of a continental arc in Fiordland, New Zealand. Journal of Metamorphic Geology 27: 281–294.
- Scott J, Muhling J, Fletcher I, Billia M, Palin JM, Elliot T et al. 2011. The relationship of Palaeozoic metamorphism and S-type magmatism on the paleo-Pacific Gondwana margin. Lithos 127: 522–534.
- Shelley D 1975. Temperature and metamorphism during cleavage and fold formation of the Greenland Group, north of Greymouth. Journal of the Royal Society of New Zealand 5: 65–75.
- Spear FS, Kohn MJ, Cheney JT 1999. P-T paths from anatectic pelites. Contributions to Mineralogy and Petrology 134: 17–32.
- Steiger R, Jäger E 1977. Subcommission on geochronology: convention on the use of decay constants in geo-and cosmochronology. Earth and Planetary Science Letters 36: 359–362.
- Tulloch AJ, Kimbrough DL 1989. The Paparoa metamorphic core complex, New Zealand: cretaceous extension associated with fragmentation of the pacific margin of Gondwana. Tectonics 8: 1217–1234.
- Tulloch AJ, Ramezani J, Kimbrough DL, Faure K, Allibone AH 2009. U-Pb geochronology of mid-Paleozoic plutonism in western New Zealand: implications for S-type granite generation and growth of the east Gondwana margin. Geological Society of America Bulletin 121: 1236–1261.
- Turnbull RE, Tulloch AJ, Ramezani J 2013. Zetland Diorite, Karamea Batholith, west Nelson: field relationships, geochemistry and geochronology demonstrate links to the Carboniferous Tobin Suite. New Zealand Journal of Geology and Geophysics 56: 83–99.
- Turner FJ 1930. The metamorphic and ultrabasic rocks of the Lower Cascade Valley, South Westland. Transactions of the New Zealand Institute 61: 170–201.
- Turner FJ 1933. The metamorphic and intrusive rocks of southern Westland. Transactions of the New Zealand Institute 63: 178–284.
- Van der Meer QHA, Scott JM, Waight TE, Sudo M, Scherstén A, Cooper AF et al. 2013. Magmatism during Gondwana break-up: new geochronological data from Westland, New Zealand. New Zealand Journal of Geology and Geophysics 56: 229–242.
- Vry J, Baker J, Maas R, Little TA, Grapes R, Dixon M 2004. Zoned (Cretaceous and Cenozoic) garnet and the timing of high grade metamorphism, Southern Alps, New Zealand. Journal of Metamorphic Geology 22: 137–157.
- Wallace RC 1974. Metamorphism of the Alpine Schist, Mataketake Range, South Westland, New Zealand. Journal of the Royal Society of New Zealand 4: 253–266.
- White PJ 1994. Thermobarometry of the Charleston metamorphic group and implications for the evolution of the Paparoa metamorphic core complex, New Zealand. New Zealand Journal of Geology and Geophysics 37: 201–209.
- White RW, Powell R, Holland TJB 2007. Progress relating to calculation of partial melting equilibria for metapelites. Journal of Metamorphic Geology 25: 511–527.
- Wyllie PJ 1977. Crustal anatexis: an experimental review. Tectonophysics 43: 41–71.
- Yardley BW 1977. The nature and significance of the mechanism of sillimanite growth in the Connemara Schists, Ireland. Contributions to Mineralogy and Petrology 65: 53–58.