Abstract
Metamorphic mineral compositions of a staurolite-bearing greyschist from the middle reaches of the Moeraki River valley in south Westland reveal peak equilibration at c. 558 ± 50 °C and c. 6.1 ± 1.2 kbar. Two c. 83 Ma U–Pb monazite age populations from the cores of monazite-apatite-allanite-epidote corona structures in mylonitised schists from near Fox Glacier confirm that Alpine Schist metamorphism occurred during the Late Cretaceous. The published spread in Late Cretaceous metamorphic ages indicates that metamorphism was diachronous or was a protracted event. Further dating is required to pin down the cryptic transition into the Jurassic–Early Cretaceous metamorphosed Otago Schist, but the Alpine Schist must extend at least 11 km east of the Alpine Fault in south Westland and overprint the suture between the Pounamu and Rakaia terranes. The P–T–t results imply that the Late Cretaceous crust represented by portions of the Alpine Schist was probably of similar thickness to that beneath the Southern Alps today, but with dehydration and partial melting occurring near the base. The crust under Westland and Otago may be dry and therefore strong.
Introduction
A significant New Zealand geological discovery of recent times is the recognition that portions of the protolith to the Alpine Schist were deposited as long ago as during the Early Cretaceous (Cooper & Ireland Citation2013, Citation2015) (). This finding remains compatible with radiometric dating results, which show Alpine Schist metamorphism to have occurred between c. 100–70 Ma (Vry et al. Citation2004; Mortimer & Cooper Citation2004). Recognition of Early Cretaceous deposition and Late Cretaceous metamorphism is significant because it means that the world-renowned metamorphic transition (e.g. Turner Citation1981) from Late Jurassic–Early Cretaceous metamorphosed prehnite-pumpellyite and greenschist facies Otago Schist (Mortimer Citation1993) through to amphibolite facies Alpine Schist (Crawford Citation1966; Cooper Citation1972) represents neither a continuous depositional nor metamorphic sequence (Cooper & Ireland Citation2015).
Figure 1 Metamorphic zonation map of the Alpine Schist. The map is modified from the QMap seamless compilation, from which data are derived from Nathan et al. (Citation2002), Cox & Barrell (Citation2007) and Rattenbury et al. (Citation2010) . Stars indicate sites for which P–T and/or radiometric data have been obtained. Mon = monazite; Zr = zircon. The K-feldspar zone is an overprint on oligoclase zone assemblages (Grapes & Watanabe Citation1992). Grey boxes are data collected in this current study. Additional data are from: (A) Cooper (Citation1980); (B) Grapes & Watanabe (Citation1992); (C) Vry et al. (Citation2004); (D) Mortimer & Cooper (2005); (E) Vry et al. (Citation2008); (F) Cooper & Ireland (Citation2013); (G) Cooper & Ireland (Citation2015).
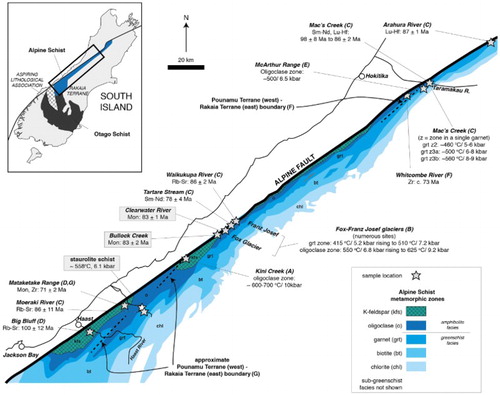
We contribute to the metamorphic story of Alpine Schist in two ways. First, we report the results of an investigation into the mineral chemistry of a staurolite-bearing schist from the Moeraki River in south Westland (Wallace Citation1975) with the specific goal of establishing quantitative P–T conditions for the area. Second, we report U–Pb monazite ages for two schists from near Fox Glacier, with the purpose of using this moderately high-temperature geochronometer to enhance knowledge of the timing of Alpine Schist amphibolite facies metamorphism in south Westland. The new quantitative data are compared to other Alpine Schist results and permit broad assessment of the thickness of the Late Cretaceous metamorphic pile.
Geological setting
The prominent metamorphic overprint that affects metasedimentary rocks east of the Alpine Fault throughout Otago and Westland is referred to as the Haast Schist and is geographically subdivided into the Otago Schist and Alpine Schist portions (e.g. Mason Citation1962; Grindley Citation1963; Mortimer Citation1993, Citation2000) (). The topic of our study, the Alpine Schist, crops out as a narrow elongate belt extending over c. 200 km along strike in the hanging wall of the Alpine Fault. This metamorphic overprint affects portions of the Rakaia Terrane (including the Aspiring Lithological Association) as well as the newly recognised Pounamu Terrane (Cooper & Ireland Citation2013, Citation2015). Pounamu Terrane rocks are dominated by quartzofeldspathic lithologies with minor metabasite, calc-silicate, chert and a basal serpentinised ultramafic zone (Cooper & Reay Citation1983; Ireland et al. Citation1984; Cooper & Ireland Citation2013, Citation2015). Protolith zircon ages from meta-tuffs just above the ultramafic rocks indicate deposition was occurring at c. 108 Ma (Cooper & Ireland Citation2013, Citation2015), long after deposition of either the adjacent Permian–Triassic Rakaia Terrane (Adams et al. Citation2007) or Late Jurassic Aspiring Lithological Association (Jugum et al. Citation2013). The suture between the Rakaia and Pounamu terranes in northern Westland is the base of the meta-ultramafic rocks, but where the meta-ultramafic rocks are absent the two terranes can currently only be subdivided using detrital zircon dates (Cooper & Ireland Citation2015) ().
Metamorphic grade in the Alpine Schist increases dramatically towards the Alpine Fault in south–central Westland (). Grapes & Watanabe (Citation1992) and Grapes (Citation1995) interpreted garnet-bearing assemblages at a distance of c. 5–8 km from the Alpine Fault zone to have formed at c. 415 °C and 5.2 kbar. Peak values of c. 10 kbar at 650–700 °C were deduced from a Cr-kyanite whiteschist adjacent to the Alpine Fault (Cooper Citation1980), with sample locations reported by McClintock & Cooper (Citation2003). By today's standards, the values derived from the whiteschists are semi-quantitative, having been constrained by the positions of reactions in a petrogenetic grid constructed for bulk-rock compositions not strictly represented by the schists. Nevertheless, the high pressures are consistent with quantitative estimates (9 kbar) for rocks near to the Alpine Fault at Fox Glacier and Franz Josef (Grapes & Watanabe Citation1992). The rapid change in calculated equilibration metamorphic pressures (c. 5–10 kbar, equating to c. 19 km thickness assuming a typical crustal density) over a distance of <8 km in central Westland means that the metamorphic zones have been thinned by faulting (e.g. Grapes & Watanabe Citation1992; Craw et al. Citation1994; Craw Citation1998; Little et al. Citation2002) and/or formed at different times (Mortimer & Cooper Citation2004).
A well-characterised garnet-bearing schist from Mac's Creek in north Westland has yielded one of the most complete P–T histories for the northern portion of the Alpine Schist (). The studied rock comes from within the Alpine Fault zone but records pre-mylonitisation prograde metamorphism from greenschist facies (c. 460 °C, 5–6 kbar) through to a peak at c. 560 °C and 8–9 kbar (Vry et al. Citation2004). That study was followed up by a detailed analysis of several garnet-bearing greyschists 3.5 km distant from the Alpine Fault zone in the nearby McArthur Range (Vry et al. Citation2008) (). Thermodynamic modelling was used to conclude that garnet growth began at c. 380 °C/2.5 kbar and continued to c. 490 °C/8.5 kbar, with the last growth phase having formed at c. 500 °C/6.5 kbar during decompression. Garnet growth primarily in the greenschist facies is supported by the plagioclase inclusions in garnet being mostly albite.
Methodology
Electron microprobe
Major-element mineral chemical compositions and X-ray maps were determined using wavelength-dispersive spectroscopy (WDS) on the University of Otago JEOL JXA-8600 electron microprobe analyser. Operating conditions were 15 kV accelerating potential, 20 nA beam current and a 1–10 µm beam diameter with counting times of 30 s on the sample and 10 s for the background. The collected raw data were converted to element wt% by comparison with characteristic X-ray peaks with Smithsonian microbeam standards (NMNH 87375, NMNH 85276, NMNH111312-44, NMNH122142, NMNH 164905, NMNH145883) using the ZAF matrix correction technique. High-resolution backscattered electron images were used to choose suitable areas for analysis with respect to the mutual mineral relations required for subsequent thermobarometry. Element maps were undertaken using wavelength-dispersal spectrometry and a 15 kV and 20 nA current, stepped every 5 μm. Fe3+ was calculated by the method of Droop (Citation1987).
Sensitive high-resolution ion microprobe (SHRIMP)
The method for obtaining monazite ages is the same as presented in Palmer et al. (Citation2015). However, details relevant to the particular Alpine Schist samples are as follows. Monazite crystals were drilled (in c. 3 mm plugs) from polished thin-sections and cast in a 25 mm epoxy disc. The primary standard reference material used for correction of measured isotopic ratios was GM3 (Kennedy & Möller Citation2012). U, Th and Pb analyses were obtained with a SHRIMP II located in the John de Laeter Centre for Isotope Research at Curtin University. Analytical spots on monazite were 6–8 μm and made with a c. 0.3 nA primary O2– ion beam. Data were corrected for instrumental mass fractionation and overcounts on 204Pb. Data processing and age calculations were performed using Squid2 and plotted using the Isoplot software of Ludwig (Citation2003).
Staurolite schist
A staurolite-bearing schist was found in situ by AF Cooper and RC Wallace in the early 1970s in oligoclase zone greyschists halfway up the Moeraki River in south Westland () (NZTM grid reference: E5140710/N1315004) (Wallace Citation1975). This specimen contains the only known occurrence of staurolite in the Alpine Schist; aluminosilicate minerals are entirely absent from quartzofeldspathic lithologies, although kyanite occurs in rare meta-chert and meta-ultramafic rocks (Cooper Citation1980; McClintock & Cooper Citation2003) and corundum is found in aluminous silica-undersaturated meta-serpentinites (Grapes & Palmer Citation1996). We have thoroughly re-inspected the staurolite-bearing sample with the aim of determining the most suitable mineral compositions for modern geothermobarometric techniques. Two thin-sections were made of different portions of the specimen and are labelled OU32550A and OU32550B.
Garnet
Garnet occurs in both specimens as euhedral to subhedral porphyroblasts commonly 1–2 mm in diameter (A), although OU32550A contains significantly more grains that are of larger diameter than OU32550B. Inclusion trails of oriented biotite, quartz, epidote, apatite, ilmenite and <0.05 mm zircon grains are common. The alignment of epidote and apatite inside and outside of garnet are evident in Ca element maps (B). The element maps show the garnets to have a high-Mn–low-Mg core and to be normally prograde zoned (C–D). Microprobe analyses reveal the cores to be Alm55Prp3Grs26Sps15And1 (Wallace Citation1975) and the rims to be Alm76Prp13Grs10Sps1And0 (). The very outermost garnet rims are subtly Mn-enriched (D), and garnet adjacent to small cracks commonly shows a slightly brighter response in backscattered electron images. Some grains have been partially replaced by chlorite.
Figure 2 Electron microprobe images of minerals in OU32550A. A, High-contrast backscattered electron image of a garnet (Grt) with inclusions of biotite (Bt), plagioclase (Pl) and quartz (Qz). The garnet has been partially replaced by chlorite (Chl). Biotite has been partially replaced by white mica (Ms). B, Ca element map shows the alignment of epidote (Ep) and apatite (Ap) through the garnet. The garnet has a slightly high Ca concentration in the core. C, The element map illustrates the prominent increase in Mg towards the rims of garnet. Note also the aligned inclusions, most of which are epidote. D, Garnets are normally zoned from a Mn-rich core to a Mn-poor rim. The very margin of the grain displays a slight Mn-enrichment.
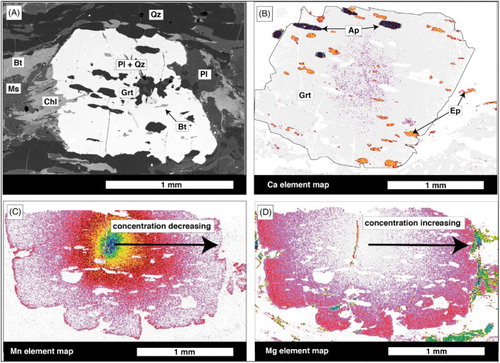
Table 1 Representative mineral analyses.a
Plagioclase
Plagioclase occurs as inclusions in garnet or distributed through the matrix as single grains and myrmekitic intergrowths. Coarse plagioclase grains show a patchy zonation under backscattered electron imaging, with the cores being slightly darker than the rims (A). This zoning relates to a change from an oligoclase interior (An24–26) to an andesine exterior (An33–34) (). The more calcic rim occurs adjacent to garnet and is in sharp contact with biotite (A–B). Albite was searched for but not found.
Staurolite
Staurolite occurs as subhedral to anhedral porphyroblasts with inclusions of quartz and ilmenite (A–B). Grain boundaries with biotite, plagioclase or quartz are commonly sharp, although many grain margins have been partially replaced by white mica, chlorite and a tiny Fe oxide that was too small to analyse (but is most likely ilmenite). Staurolite grains are Fe-rich and not systematically zoned; Mg# (=100×Mg/(Mg+Fe)) varies between 17 and 19 (). Grains contain 2.3–3.0 wt% ZnO.
Figure 4 A, OU32550A: backscattered electron image of staurolite (St) with quartz (Qz) inclusions. Margins of staurolite in contact with plagioclase (Pl), quartz (Qz) and biotite (Bt) appear sharp and in textural equilibrium. White mica (Ms) overprints biotite and impinges on the staurolite margins. B, OU32550B: staurolite with inclusions of ilmenite (Ilm) under replacement by white mica, chlorite (Chl) and ilmenite. Biotite is also being replaced by white mica.
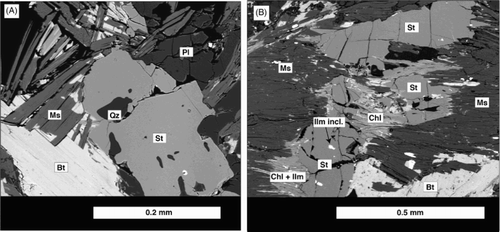
Biotite
Biotite defines the schistosity in the rocks and also occurs as inclusions within garnet (A). Biotite has an average Mg# of 53 in OU32550A and 55 in OU32550B (). The averaged biotite TiO2 concentration in OU32550A (1.55 wt %) is slightly lower than OU32550B (1.63 wt %). Unlike garnet and plagioclase, biotite shows no systematic zoning although some grains have been partially chloritised, especially along cleavage planes. These alteration areas were avoided as much as possible with the microprobe beam, although some were evidently encountered within the electron beam activation area as indicated by a slight decrease in K2O content in some analyses. Many biotite grains have been partially replaced by white mica. Several analyses have anomalously low TiO2 (<1 wt%) and high SiO2 (>37 wt%), and probably represent beam overlap with white mica.
White mica
White mica is a prominent phase in the investigated rocks. It appears to mainly be a replacement mineral as it overgrows biotite (A) and occurs along with chlorite ± ilmenite partially replacing staurolite grain margins (B). These reaction textures probably represent a hydration retrograde reaction (unbalanced) such as: biotite + staurolite + H2O = white mica + chlorite + Fe–Ti oxide. White mica may also be a peak metamorphic phase, but the degree of retrogression of biotite, and hence the abundance of secondary white mica, makes deciphering which grains are the peak metamorphic assemblage particularly difficult.
P–T conditions
The textural relationships in OU32550A and OU32550B suggest that garnet, andesine, biotite, staurolite, ilmenite, epidote and quartz represent the peak metamorphic assemblage. The occurrence of oligoclase/andesine, rather than peristerite feldspars known to occur in lower-temperature Alpine Schist, indicates temperatures in excess of c. 500 °C at moderate pressures (Crawford Citation1966; Cooper Citation1972; Grapes & Otsuki Citation1983; Vry et al. Citation2008). The absence of leucosomes in this pelitic rock suggests water-saturated partial melting has not occurred and therefore that temperatures most likely did not exceed c. 675 °C. In addition to establishing the peak mineral assemblage, calculating T and P requires determining which portions of zoned minerals (e.g. garnet and plagioclase in our case) represent an equilibrium state. Since the garnets show normal growth zoning patterns, the composition selected for geothermobarometry is that with the highest Mg# and lowest Mn and Ca, which occurs close to the rims (B–C). For plagioclase, the andesine compositions are considered most likely to be in equilibrium with garnet as more calcic plagioclase domains typically occur on the feldspar rims and occur adjacent to garnet (A–B); the increase in Ca in plagioclase and decrease in Ca in garnet suggests net transfer between these two phases since other Ca-bearing phases, such as epidote, are only a minor component.
Conventional geothermobarometry
Simultaneous solution of the Fe–Mg garnet-biotite (GB) exchange geothermometer (Holdaway Citation2000) and garnet-biotite-plagioclase-quartz (GBPQ) geobarometer (Wu et al. Citation2004) for the inferred peak assemblages yield P–T values of 579 °C and 6.5 kbar for OU32550A and 537 °C and 5.6 kbar for OU32550B. The error endemic to each geothermobarometric method is c. ± 50 °C (Holdaway Citation2000) and ± 1.2 kbar (Wu et al. Citation2004). The average of these two values is 558 ± 50 °C and 6.1 ± 1.2 kbar. Since a Ti-saturation phase (ilmenite) is present and the P of metamorphism is very close to the calibrated 4–6 kbar Ti-in-biotite saturation surface, Ti-in-biotite geothermometry (Henry et al. Citation2005) can be applied as an independent check for garnet-biotite equilibrium. Although rutile is absent and therefore the aTiO2 cannot be easily constrained, meta-pelites with ilmenite commonly have values of >0.8 –1.0 (e.g. Ghent & Stout Citation1984; Ashley & Law Citation2015). Assuming an aTiO2 = 1, the average Ti-in-biotite temperature for all unchloritised biotite analyses is 575 °C ± 50 °C (2σ, with this value representing the intra-sample scatter) in OU32550A and 556 °C (±47 °C, 2σ) in OU32550B. Both Ti-in-biotite temperature estimates overlap with the values calculated by garnet-biotite exchange thermometry, indicating that the selected garnet and biotite analyses permissibly attained equilibrium.
Equilibrium thermodynamic analysis
We have not undertaken bulk-rock metamorphic thermodynamic modelling of the staurolite schist specimen for several reasons. First, the examined samples record the influx of H2O by the chloritisation of garnet and micas, and the only way that a bulk-rock geochemical analysis could be used would be to assume that the alteration was isochemical with respect to all other elements. Second, a significant correction would need to be made to account for fractionation during garnet and plagioclase grain growth, since the interiors of these minerals are not in equilibrium with minerals in the rock matrix. Thirdly, the mineral assemblages and mineral compositions of OU32550A and OU32550B are subtly different, which means that the sample composition must vary on a small scale and a bulk-rock analysis would combine these different domains. Instead, we supplement the conventional geothermobarometric estimates using the AveragePT method in the Thermo-Calc computer program and the Holland & Powell (Citation1998) internally consistent database. For OU32550A, the following independent reactions:
23 grossular + 6 Mg–staurolite + 48 quartz = 8 pyrope + 69 anorthite + 12 H2O;
23 grossular + 6 Fe-staurolite + 48 quartz = 8 almandine + 69 anorthite + 12 H2O;
pyrope + 2 grossular + 3 eastonite + 6 quartz = 3 phlogopite + 6 anorthite; and
2 grossular + 3 almandine + 3 eastonite + 6 quartz = 2 pyrope + 3 annite + 6 anorthite
yield a rather imprecise intercept of 682 ± 170 °C (2σ) and 8.9 ± 4.0 (2σ) kbar. For OU32550B, reactions (1) and (2) as well as:
23 phlogopite + 4 Mg-staurolite = 13 pyrope + 23 eastonite + 14 quartz + 8 H2O; and
85 phlogopite + 12 Fe–staurolite = 39 pyrope + 16 annite + 69 eastonite + 42 quartz + 24 H2O
yield an equally imprecise average intercept of 648 °C ± 194 °C (2σ) and 9.1 ± 4.8 (2σ) kbar. Despite the poor precision, the results in both cases overlap with the conventionally derived P–T estimates. The GBPQ geobarometer is based on reaction (4) but uses a different set of solution models for the behaviour of garnet, biotite and plagioclase (Wu et al. Citation2004).
U–Pb monazite dating
In an attempt to locate monazite in the Alpine Schist and better constrain the timing of amphibolite facies metamorphism, we undertook a regional reconnaissance study on a series of schist specimens held in the University of Otago rock collection. Unfortunately, most inspected samples contain Rare Earth Element (REE)-bearing epidote group minerals, including the staurolite-bearing schist described above, instead of readily datable REE-bearing phosphates. However, monazite was identified in two mylonitic quartzofeldspathic schists from near Fox Glacier: one from Bullock Creek (OU83148; NZTM grid reference E1353155/N5178501) and another from the Clearwater River (OU85057; NZTM grid reference E5138279/N1360192) ().
Monazite in OU83148 and OU85057 occurs in the cores of spectacular coronitic structures that are similar to those described from the Swiss Alps by Finger et al. (Citation1998). In both studied Alpine Schist samples a central monazite grain is typically surrounded by apatite containing blebs of thorite, and then by allanite with epidote at the outermost margins (). The blebs of thorite in apatite may represent dissolution of originally coarse monazite via a reaction such as monazite + Ca–silicate + fluid = allanite + apatite + epidote + thorite (Regis et al. Citation2012). Because of the scarcity of monazite, data are restricted to seven analyses made on two monazite grains in each sample (). In OU83148, two analyses are outliers with the remaining five yielding a pooled 238U/206Pb age of 82.9 ± 2.3 Ma with a mean square weighted deviation (MSWD) of 2.3. In sample OU85057, one analysis has >2% common-Pb and is excluded from the age calculation and there is one outlier. The five remaining analyses yield a pooled 238U/206Pb age of 82.6 ± 1.0 Ma with a MSWD of 0.9.
Figure 5 A, OU83148: backscattered electron imaging reveals phosphate-epidote corona structures in Alpine Schist from Bullock Creek and the Clearwater River in south Westland (see for locations). The coronas consist of a monazite (Mon) core, surrounded by apatite (Ap) and blebs of thorite (Th), allanite (All) and epidote (Ep). The outer portions of the coronas are parallel to the mylonitic fabric, which consists of quartz (Qz), biotite (Bt) and white mica (Ms). A grain of zircon (Zr) occurs in the matrix. B, OU85057: the zones surrounding monazite consist of small blebs of thorite within apatite, perhaps due to dissolution of an earlier-formed coarser-grained monazite.
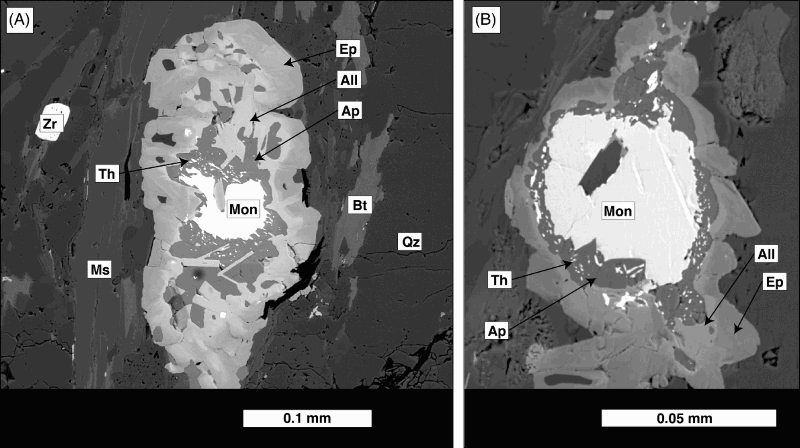
Table 2 Monazite U–Th–Pb data.
Discussion
P–T conditions of south–central Westland Alpine Schist
The P–T estimates derived from the oligoclase zone staurolite schist in the Moeraki River valley indicate peak equilibration in the amphibolite facies at c. 558 ± 50 °C and c. 6.1 ± 1.2 kbar. As the investigated staurolite schist occurs c. 11 km from the Alpine Fault and close to the garnet zone (which contains albite in the Haast area), we compare our P–T estimates with those made on rocks just above the albite-out isograd in the Fox Glacier–Franz Josef area by Grapes & Watanabe (Citation1992) (). Their estimates for albite-free oligoclase zone schists cluster at T = 500 –550 °C and P = 6–8 kbar (±1 kbar) (), which is within error of our results. A point of difference between areas is that the oligoclase metamorphic mineral zone is much closer to the Alpine Fault in the Fox Glacier–Franz Josef area than in the Haast area ().
Figure 6 Summary of the Cretaceous P–T path for the Alpine Schist in south Westland. The geothermobarometric results from OU32550A and OU32550B are indicated by the two transparent ovals with thick margins. Grapes & Watanabe's (Citation1992) geothermobarometric results from Fox Glacier and Franz Josef are represented by white and grey circles with thin margins; ellipses represent the addition of goethermobarometer-endemic errors of ±50 °C and 1 kbar. The arrowed clockwise trajectories are P–T paths from Grapes & Watanabe (Citation1992) and the thick black line indicates their inferred metamorphic array. Ca-plagioclase-in and albite-out reactions are from Vry et al. (Citation2008).
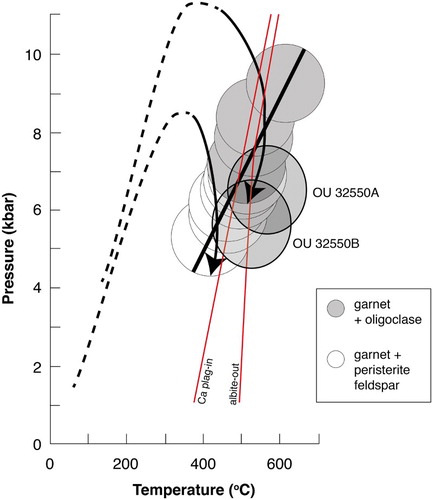
Grapes & Watanabe (Citation1992) interpreted their Fox Glacier–Franz Josef P–T data to show that the Alpine Schist metamorphic array records peak T and textural equilibrium during decompression. This is common in collisional environments because rocks follow a clockwise P–T path in which heating lags behind attainment of maximum pressure (e.g. Thompson Citation1990). More recently, however, Vry et al. (Citation2008) have suggested that the Grapes & Watanabe (Citation1992) P–T estimates are too high by c. 2 kbar for the observed mineral assemblages. Assuming that (1) the mineral analyses of Grapes & Watanabe (Citation1992) were of appropriate quality for geothermobarometry and (2) their geothermobarometry has yielded accurate results, the interpretation of Vry et al. (Citation2008) throws doubt on the use of conventional geothermobarometry in the Alpine Schist (including our work), and therefore requires further examination.
The conclusion of Vry et al. (Citation2008) that conventional geothermobarometry estimates are too high is based upon the results of their own equilibrium thermodynamic modelling. Using the results of a pseudosection calculated for a greyschist from the McArthur Range, and assuming that this rock has an equivalent composition to greyschists in the Fox Glacier–Franz Josef area, Vry et al. (Citation2008) suggested that the albite-free oligoclase zone schists at Fox Glacier–Franz Josef plot in P–T space where they should instead contain both oligoclase and albite (). As a result, they suggest that the P–T array of Grapes & Watanabe (Citation1992) should be shifted down in P by c. 2 kbar to match their calculations. However, when the endemic errors of ±50 °C and ±1.0 kbar are applied to the Grapes & Watanabe (Citation1992) results, the P–T estimates actually overlap areas either side of the positions of Ca plag-in and albite-out reactions (). Furthermore, there are uncertainties in determining the precise position of reactions using an internally consistent thermodynamic modelling package; for example, solution models are still being debated for even relatively simple minerals such as feldspar. The currently limited thermodynamic activity models for the behaviour of Ti, Mn and/or Fe3+ in many minerals will also influence the exact positions of reactions. Finally, no two bulk-rock compositions of different rocks are likely to be identical, which means that even subtle chemical differences are likely to shift the calculated oligoclase and albite stability fields. While pseudosection analysis yields powerful insights into the Alpine Schist P–T path, we suggest that P–T estimates derived from experimentally calibrated metamorphic reactions via conventional geothermobarometry (if the data are of good quality), are capable of providing reliable P and T values. As a result, we consider our P–T estimates calculated by the GB-GBPQ method (supported by Ti-in-biotite estimates) to be an appropriate representation of the conditions of oligoclase zone metamorphism in the Moeraki River area.
Timing of metamorphism
Although monazite was not located in the staurolite-bearing specimen and the exact age of staurolite formation is undated, Vry et al. (Citation2004) report a Rb–Sr isochron age of 86.2 ± 11 Ma for a magnetite-garnet schist specimen collected by GW Grindley in the 1960s from c. 1 km east of the staurolite schist locality (). This date is only a two-point isochron and therefore not very robust, but it does overlap with the two new monazite ages (82.9 ± 2.3 Ma and 82.6 ± 1.0 Ma). The staurolite-bearing schist's amphibolite facies fabric is therefore most probably Late Cretaceous in age and not part of the Otago Schist, which formed during Late Jurassic–Early Cretaceous time (e.g. Gray & Foster Citation2004). This conclusion means that the Alpine Schist overprint must extend at least as far to the east as the middle reaches of the Moeraki River valley, 11 km from the Alpine Fault, and well into the oligoclase zone. The Alpine Schist in south Westland must also overprint the suture between the Pounamu Terrane and Rakaia Terrane (), which is constrained from detrital zircon data by Cooper & Ireland (Citation2015) to occur southwest of the staurolite-bearing schist sample site ().
Despite resolving tight c. 83 Ma monazite ages from two mylonitised Alpine Schists in the course of this study, these are distinct from a younger monazite age of 71 ± 2 Ma for a greyschist from the Mataketake Range (Mortimer & Cooper Citation2004; confirmed by recent U–Pb dating of zircon metamorphic overgrowths in the same sample by Cooper & Ireland Citation2015) (). The difficulty with monazite is ascertaining the precise metamorphic conditions at which it grew. Although monazite commonly forms at amphibolite facies conditions, it has also been documented to form within greenschist facies rock (e.g. Franz et al. Citation1996). This is further complicated in the two studied monazite-bearing rocks because Alpine Fault mylonitisation obscures the relationship of monazite to the amphibolite facies assemblages that are now represented by porphyroclasts of garnet, plagioclase, etc. in these specimens. Mortimer & Cooper (Citation2004) also obtained a four-point Rb–Sr isochron age of 100 ± 12 Ma from an amphibolite at Big Bluff in the Haast River valley that is along strike from the c. 71 Ma monazite age. To the north of Fox Glacier, Vry et al. (Citation2004) report a three-point Rb–Sr age of 86 ± 2 Ma from Waikukupa River and a two-point Sm–Nd age of 78 ± 4 Ma from Tartare Stream (). Further north still, Cooper and Ireland (Citation2013) report c. 73 Ma zircon overgrowths on detrital grains, and Vry et al. (Citation2008) resolved Lu–Hf and Sm–Nd garnet ages of 98 ± 8 Ma and 86 ± 2 Ma (). Evidently Alpine Schist metamorphism is a Late Cretaceous event, but the formation of the observed fabrics was diachronous and/or occurred over a protracted time period (e.g. Mortimer & Cooper Citation2004).
The new metamorphic monazite ages overlap with the emplacement age of at least one granite pegmatite dyke (Batt et al. Citation1999) from the swarm in the Mataketake Range (Wallace Citation1974). This dyke swarm was emplaced from c. 82 –68 Ma and represents anatectic crustal melting (Chamberlain et al. Citation1995). The peak temperatures calculated by geothermobarometry for the known highest grade Alpine Schist (c. 625 °C; Grapes & Watanabe Citation1992) are, however, substantially below the point at which water-saturated partial melting of a pelite should take place (c. 675 °C). The pegmatites must therefore be derived from deeper in the metamorphic pile than is currently exposed (A). The highest calculated pressures for amphibolite facies metamorphic fabrics, c. 10 kbar from directly above the Alpine Fault (Cooper Citation1980; Grapes & Watanabe Citation1992; Vry et al. Citation2004), equate to c. 36 km depth (assuming a typical crustal density of 2.85 g cm–3). To achieve melting at a minimum of water-saturated temperatures means that the Cretaceous crust was as thick as the crustal root today beneath the Southern Alps (c. 44 km; Scherwath et al. Citation2003). As the highest grade Alpine Schist at the surface is found directly above the Alpine Fault, the lower crustal décollement thought to be associated with the Alpine Fault (e.g. Koons et al. Citation2003) may have dissected unmelted amphibolite facies rocks containing pegmatites in the hanging wall from a zone of partial melting in the footwall (B). Late Cretaceous metamorphism has also been recognised in lower crustal gabbroic and granulite xenoliths in Oligocene–Miocene alkaline intraplate basalts in East Otago (Beinlich et al. Citation2006; Ireland et al. Citation2009), although Otago Schist at the surface records only Late Jurassic–Early Cretaceous metamorphism. Portions of the lower crust beneath Westland and Otago are therefore probably quite dry and therefore strong.
Figure 7 A, Simple block cartoon illustrating the distribution of c. 100 –70 Ma greenschist and amphibolite facies metamorphic fabrics. This metamorphic event was accompanied by partial melting at or near the base of the crust, the products of which are seen as pegmatite dykes in Alpine Schist. Granulite facies crustal xenoliths in East Otago also record Late Cretaceous metamorphic ages (Ireland et al. Citation2009). B, Schematic present-day cross- section through Westland and Otago. The Alpine Schist assemblages are being exhumed in the hanging wall of the Alpine Fault. The lower crustal décollement, predicted from thermo-mechanical modelling to join the Alpine Fault at depth (Koons et al. Citation2003), may have localised along the top of lower crust that was dehydrated during the Late Cretaceous.
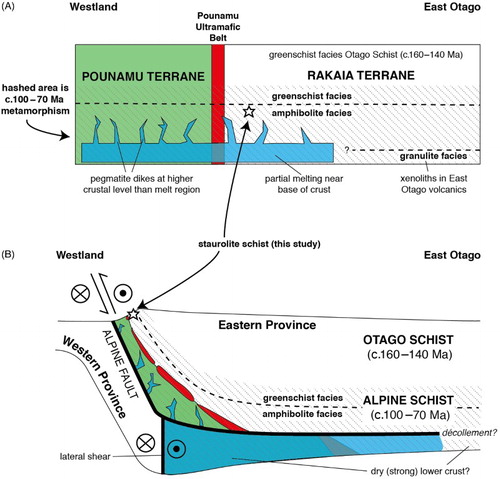
Conclusions
Our analyses offer new insights into metamorphism of the Alpine Schist in south Westland.
Investigation of the only known sample of staurolite-bearing schist reveals that the averaged P–T conditions attained by oligoclase zone rocks in the Moeraki River valley were c. 558 ± 50 °C and c. 6.1 ± 1.2 kbar. Peak metamorphism of the staurolite schist most likely occurred during the Late Cretaceous, which means that the metamorphic fabric in the investigated sample cannot be part of the older (c. 140 Ma) Otago Schist. This also means that the cryptic transition with the Otago Schist must occur further to the east, and the Alpine Schist overprints the suture between the Rakaia and Pounamu terranes.
Two U–Pb ages of c. 83 Ma for monazite populations in greyschists near Fox Glacier confirm Late Cretaceous Alpine Schist metamorphism. These ages are older than the one other monazite date from the Alpine Schist (c. 71 Ma), and so support the inference of Mortimer & Cooper (Citation2004) that Late Cretaceous Alpine Schist metamorphism was either diachronous or was a protracted event.
Integration of the P–T–t data, coupled with the occurrence of pegmatite dykes, indicates that the Late Cretaceous crust beneath Westland was of similar thickness to that below the Southern Alps today, but with melting taking place near the base. Similar metamorphic ages have been obtained from granulite and gabbroic xenoliths from beneath East Otago, which implies that the lower crust under these areas is probably regionally dry and therefore strong.
Acknowledgements
We appreciate the time and effort of B Dragovic, R Jongens, N Mortimer, J Vry and an anonymous reviewer in reviewing the manuscript. We also acknowledge the facilities of the Australian Microscopy & Microanalysis Research Facility at the Centre for Microscopy, Characterisation & Analysis at the University of Western Australia, a facility funded by the University, State and Commonwealth Governments of Australia. Monazite U–Th–Pb analyses were conducted using the SHRIMP ion microprobes of the John de Laeter Centre at Curtin University, Perth, Australia.
Associate Editor: Dr Richard Jongens.
Disclosure statement
No potential conflict of interest was reported by the authors.
References
- Adams CJ, Campbell HJ, Griffin WL 2007. Provenance comparisons of Permian to Jurassic tectonostratigraphic terranes in New Zealand: perspectives from detrital zircon age patterns. Geological Magazine 144: 701–729.
- Ashley KT, Law RD 2015. Modeling prograde TiO2 activity and its significance for Ti-in-quartz thermobarometry of pelitic metamorphic rocks. Contributions to Mineralogy and Petrology 169: 23. doi:10.1007/s00410-015-1118-7
- Batt GE, Kohn BP, Braun J, McDougall I, Ireland TR 1999. New insight into the dynamic development of the Southern Alps, New Zealand, from detailed thermochronological investigation of the Mataketake range pegmatites. In: Ring U, Brandon MT, Lister GS, Willet SD, Exhumation processes: normal faulting, ductile flow and erosion. Geological Society 145: 261–282.
- Beinlich A, Palin J, Cooper AF 2006. Accessory mineral U-Pb Ti-Zr thermochronology of the deep crust of Zealandia: Rift, break-up and drift from 90 to 20 Ma. American Geophysical Union Fall Meeting 2006, abstract #V31B-0581. 2006AGUFM.V31B0581B.
- Chamberlain CP, Zeitler PK, Cooper AF 1995. Geochronologic constraints of the uplift and metamorphism along the Alpine Fault, South Island, New Zealand. New Zealand Journal of Geology and Geophysics 38(4): 515–523.
- Cooper AF 1972. Progressive metamorphism of metabasic rocks from the Haast Schist Group of southern New Zealand. Journal of Petrology 13: 457–492.
- Cooper AF 1980. Retrograde alteration of chromian kyanite in metachert and amphibolite whiteschist from the Southern Alps, New Zealand, with implications for uplift on the Alpine Fault. Contributions to Mineralogy and Petrology 75: 153–164.
- Cooper AF, Ireland TR 2013. Cretaceous sedimentation and metamorphism of the western Alpine Schist protoliths associated with the Pounamu Ultramafic Belt, Westland, New Zealand. New Zealand Journal of Geology and Geophysics 56: 188–199.
- Cooper AF, Ireland TR 2015. The Pounamu terrane, a new Cretaceous exotic terrane within the Alpine Schist, New Zealand; tectonically emplaced, deformed and metamorphosed during collision of the LIP Hikurangi Plateau with Zealandia. Gondwana Research 27: 1255–1269.
- Cooper AF, Reay A 1983. Lithology, field relationships, and structure of the Pounamu Ultramafics from the Whitcombe and Hokitika Rivers, Westland, New Zealand. New Zealand Journal of Geology and Geophysics 26: 359–379.
- Cox SC, Barrell DJA (compilers) 2007. Geology of the aoraki area: scale 1:250,000. Institute of Geological & Nuclear Sciences 1:250,000 geological map 15. Lower Hutt, GNS Science. 71 p. + 1 folded map.
- Craw D 1998. Structural boundaries and biotite and garnet ‘isograds’ in the Otago and Alpine Schists, New Zealand. Journal of Metamorphic Geology 16: 395–402.
- Craw D, Rattenbury MS, Johnstone RD 1994. Structures within greenschist facies Alpine Schist, central Southern Alps, New Zealand. New Zealand Journal of Geology and Geophysics 37: 101–111.
- Crawford ML 1966. Composition of plagioclase and associated minerals in some schists from Vermont, USA, and South Westland, New Zealand, with inferences about the peristerite solvus. Contributions to Mineralogy and Petrology 13: 269–294.
- Droop GTR 1987. A general equation for estimating Fe3+ concentrations in ferromagnesian silicates and oxides from microprobe analyses, using stoichiometric criteria. Mineralogical Magazine 51(361): 431–435.
- Finger F, Broska I, Roberts MP, Schermaier A (1998). Replacement of primary monazite by apatite-allanite-epidote coronas in an amphibolite facies granite gneiss from the eastern Alps. American Mineralogist 83: 248–258.
- Franz G, Andrehs G, Rhed D (1996). Crystal chemistry of monazite and xenotime from Saxothuringian-Moldanubian metapelites, NE Bavaria, Germany. European Journal of Mineralogy 8: 1097–1118.
- Ghent ED, Stout MZ 1984. TiO2 activity in metamorphosed pelitic and basic rocks: principles and applications to metamorphism in southeastern Canadian Cordillera. Contributions to Mineralogy and Petrology 86: 248–255.
- Grapes RH 1995. Uplift and exhumation of Alpine Schist, Southern Alps, New Zealand: thermobarometric constraints. New Zealand Journal of Geology and Geophysics 38: 525–533.
- Grapes R, Otsuki M 1983. Peristerite compositions in quartzofeldspathic schists, Franz Josef-Fox Glacier area, New Zealand. Journal of Metamorphic Geology 1: 47–61.
- Grapes R, Palmer K 1996. (Ruby—Sapphire)—Chromian Mica—Tourmaline Rocks from Westland, New Zealand. Journal of Petrology 37: 293–315.
- Grapes R, Watanabe T 1992. Metamorphism and uplift of Alpine schist in the Franz Josef-Fox Glacier area of the Southern Alps, New Zealand. Journal of Metamorphic Geology 10: 171–180.
- Gray DR, Foster DA 2004. 40Ar/39Ar thermochronologic constraints on deformation, metamorphism and cooling/exhumation of a Mesozoic accretionary wedge, Otago Schist, New Zealand. Tectonophysics 385: 181–210.
- Grindley GW 1963. Structure of the Alpine schists of south Westland, Southern Alps, New Zealand. New Zealand Journal of Geology and Geophysics 6: 872–930.
- Henry DJ, Guidotti CV, Thomson JA 2005. The Ti-saturation surface for low-to-medium pressure metapelitic biotites: implications for geothermometry and Ti-substitution mechanisms. American Mineralogist 90(2–3): 316–328.
- Holdaway MJ 2000. Application of new experimental and garnet Margules data to the garnet-biotite geothermometer. American Mineralogist 85(7–8): 881–892.
- Holland TJB, Powell R 1998. An internally consistent thermodynamic data set for phases of petrological interest. Journal of Metamorphic Geology 16: 309–343.
- Ireland TR, Reay A, Cooper AF 1984. The The Pounamu Ultramafic Belt in the Diedrich Range, Westland, New Zealand. New Zealand Journal of Geology and Geophysics 27: 247–256.
- Ireland T, Reay A, Sipiera P 2009. Geochronology of granulites from the Kakanui mineral breccia. In: Barrell DJA, Tulloch AJ eds. Geological Society of New Zealand and New Zealand Geophysical Society Joint Annual Conference, Oamaru, 23–27 November 2009: programme and abstracts. Geological Society of New Zealand miscellaneous publication 128A. Wellington, Geological Society of New Zealand.
- Jugum D, Norris RH, Palin JM 2013. Late Jurassic detrital zircons from the Haast Schist and their implications for New Zealand terrane assembly and metamorphism. New Zealand Journal of Geology and Geophysics 56: 223–228.
- Kennedy AK, Möller A 2012. New monazite reference material for microanalysis. Microanalytical Reference Materials. Microanalysis Society Golden Colorado Abstracts May 15–17: 14–15.
- Koons PO, Norris RJ, Craw D, Cooper AF 2003. Influence of exhumation on the structural evolution of transpressional plate boundaries: an example from the Southern Alps, New Zealand. Geology 31: 3–6.
- Little TA, Holcombe RJ, Ilg BR 2002. Ductile fabrics in the zone of active oblique convergence near the Alpine Fault, New Zealand: identifying the neotectonic overprint. Journal of Structural Geology 24: 193–217.
- Ludwig KR 2003. User’s manual for ISOPLOT 3, a geochronological toolkit for Microsoft Excel. Special Publication No. 4. Berkeley, CA, Berkeley Geochronology Center.
- Mason BH 1962. Metamorphism in the Southern Alps of New Zealand. Bulletin of the American Museum of Natural History 123(4): 211–248.
- McClintock MK, Cooper AF 2003. Geochemistry, mineralogy, and metamorphic history of kyanite-orthoamphibole-bearing Alpine Fault mylonite, South Westland, New Zealand. New Zealand Journal of Geology and Geophysics 46: 47–62.
- Mortimer N 1993. Jurassic tectonic history of the Otago Schist, New Zealand. Tectonics 12: 237–244.
- Mortimer N 2000. Metamorphic discontinuities in orogenic belts: example of the garnet–biotite–albite zone in the Otago Schist, New Zealand. International Journal of Earth Sciences 89: 295–306.
- Mortimer N, Cooper AF 2004. U-Pb and Sm-Nd ages from the Alpine Schist, New Zealand. New Zealand Journal of Geology and Geophysics 47: 21–28.
- Nathan S, Rattenbury MS, Suggate RP (compilers) 2002. Geology of the Greymouth area: scale 1:250,000. Institute of Geological & Nuclear Sciences 1:250,000 geological map 12. Lower Hutt, Institute of Geological & Nuclear Sciences. 58 p. + 1 folded map.
- Palmer MC, Scott JM, Muhling JR, Kennedy AK 2015. Carboniferous metamorphism and partial melting of the Greenland Group in the Jackson River valley, south Westland. New Zealand Journal of Geology and Geophysics 58: 22–32.
- Rattenbury MS, Jongens R, Cox SC (compilers) 2010. Geology of the haast area: scale 1:250,000. Institute of Geological & Nuclear Sciences 1:250,000 geological map 14. Lower Hutt, Institute of Geological & Nuclear Sciences. 58 p. + 1 folded map.
- Regis D, Cenki-Tok B, Darling J, Engi M 2012. Redistribution of REE, Y, Th, and U at high pressure: allanite-forming reactions in impure meta-quartzites (Sesia Zone, Western Italian Alps). American Mineralogist 97(2–3): 315–328.
- Scherwath M, Stern T, Davey F, Okaya D, Holbrook WS, Davies R, Kleffmann S 2003. Lithospheric structure across oblique continental collision in New Zealand from wide-angle P wave modeling. Journal of Geophysical Research: Solid Earth 108(B12): 2566. doi:10.1029/2002JB002286
- Thompson AB 1990. Heat, fluids and melting in the granulite facies. In: Vielzeuf D, Vidal PH eds. Granulites and crustal evolution. Dordrecht, Kluwer Academic Publishers. Pp. 37–57.
- Turner FJ 1981. Metamorphic petrology. Washington, DC, Hemisphere Publishing Corporation. 524 p.
- Vry JK, Baker J, Maas R, Little TA, Grapes R, Dixon M 2004. Zoned (Cretaceous and Cenozoic) garnet and the timing of high grade metamorphism, Southern Alps, New Zealand. Journal of Metamorphic Geology 22(3): 137–157.
- Vry JK, Powell R, Williams J 2008. Establishing the P–T path for Alpine Schist, Southern Alps near Hokitika, New Zealand. Journal of Metamorphic Geology 26: 81–97.
- Wallace RC 1974. Metamorphism of the Alpine Schist, Mataketake Range, South Westland, New Zealand. Journal of the Royal Society of New Zealand 4: 253–266.
- Wallace RC 1975. Staurolite from the haast schist in South Westland. New Zealand Journal of Geology and Geophysics 18(2): 343–348.
- Wu CM, Zhang J, Ren LD 2004. Empirical garnet–biotite–plagioclase–quartz (GBPQ) geobarometry in medium-to high-grade metapelites. Journal of Petrology 45: 1907–1921.