ABSTRACT
The Glenroy Complex consists of retrogressed monzodioritic granulites (Woodham Orthogneiss) and heterogeneous metasedimentary rocks (Davis Creek Paragneiss). U–Pb zircon dating shows that the Woodham Orthogneiss comprises rocks ranging in age from 121.8 to 126.6 Ma that are correlatives of the geochemically similar Western Fiordland Orthogneiss Worsley Pluton in northern Fiordland. Permian–Jurassic detrital zircon age peaks in the Davis Creek Paragneiss indicate a maximum depositional age of c. 227 Ma, suggesting correlation with George Sound Paragneiss in northern Fiordland and the paleo-Pacific Gondwana margin cover sequence. Metamorphism of the Davis Creek Paragneiss is constrained to 120.6 ± 0.9 Ma by U–Pb dating of low Th/U zircon overgrowths. The McKnee Intrusives were intruded in two discrete episodes at 225.0 ± 2.7 Ma and 137.8 ± 0.7 Ma which, together with their geochemistry, suggests correlation with northern Fiordland Late Triassic and Early Cretaceous Darran Suite plutons. The HiSY Mt Cann Pluton has an age of 113.8 ± 1.0 Ma and is correlated with the Separation Point Granite. The Glenroy Complex and McKnee Intrusives were likely exhumed during at least two stages: initially in the Cretaceous prior to displacement from their northern Fiordland correlatives by the Alpine Fault; and during emplacement as tectonic blocks in the Matakitaki foreland thrust system at the Big Bend.
Introduction
The only well-known occurrences of granulites in New Zealand are in western Fiordland (Allibone et al. Citation2009b) and xenoliths found in Cenozoic volcanic rocks (e.g. Reay & Sipiera Citation1987). The Glenroy Complex, southeast Nelson, includes the only contiguous granulite facies rocks in New Zealand outside of Fiordland (Rattenbury et al. Citation2006). Despite this, the Glenroy Complex and adjacent plutonic rocks (McKnee Intrusives and Mt Cann Pluton) have received only cursory examination in the past (e.g. Adamson Citation1966; Hattori Citation1967; Fyfe Citation1968; Grew Citation1977; Tulloch & Campbell Citation1993). Glenroy Complex orthogneisses have previously been correlated with similar rocks in Nelson and Fiordland, such as the Pembroke Granulite of the Arthur River Complex (ARC) (Blattner & Graham Citation2000) and Western Fiordland Orthogneiss (WFO) (Tulloch Citation1981, Citation1983; Tulloch & Brathwaite Citation1986; Bradshaw Citation1989a; Kimbrough et al. Citation1993; Muir et al. Citation1995; Allibone et al. Citation2009b). However, to date these correlations have not been rigorously tested, and the absolute age and geochemical character of the Glenroy Complex remain unknown.
This paper provides the first detailed description, geochemistry and U–Pb zircon geochronology of the Glenroy Complex and adjacent plutonic rocks. The data are used to assess the stratigraphic nomenclature, age and affinity of high-grade metamorphic and plutonic crystalline rocks in the Glenroy area. The Glenroy area is in southeast Nelson, c. 35 km south of Murchison and includes the middle–lower Glenroy and Matakitaki river catchments in the NZTopo50 BS23 Matakitaki and BT25 Lewis Pass map sheets. Details of the revised stratigraphic nomenclature are provided in the Appendix. Map grid references for outcrop localities are given using the New Zealand Transverse Mercator Projection (NZTM) coordinate system.
Regional geological setting and background
The geology of New Zealand is commonly divided into pre-Late Cretaceous basement and unconformably overlying Late Cretaceous–Cenozoic sedimentary and volcanic rocks. The basement rocks are subdivided into the predominately Paleozoic Western Province, Paleozoic–Mesozoic Median Batholith and Late Paleozoic–Mesozoic Eastern Province. The Western and Eastern provinces are further subdivided into tectonostratigraphic terranes and batholiths. The Western Province terranes were part of the paleo-Pacific margin of Gondwana by at least Devonian time, while those of the Eastern Province were accreted to the edge of the supercontinent during the Mesozoic (Mortimer et al. Citation2014).
The basement terranes have been dextrally offset c. 480 km along the Australian–Pacific plate boundary Alpine Fault since c. 23 Ma (Benson Citation1952; Kamp Citation1986; Cooper et al. Citation1987). At a regional scale, the c. 850 km long Alpine Fault has a remarkably straight surface trace except in southeast Nelson where there is a gentle S-shaped restraining bend (A; Berryman et al. Citation1992). The bend is informally referred to as the ‘Big Bend’ due to similarities with the Transverse Ranges area of the San Andreas Fault (Yeats & Berryman Citation1987).
Figure 1. A, Geological map of the Glenroy area, compiled from new field-mapping, Cutten (Citation1987), Campbell (Citation1992) and Rattenbury et al. (Citation2006). Topographic data sourced from the Land Information New Zealand (LINZ) Data Service, and licensed for re-use under the Creative Commons Attribution 3.0 New Zealand licence. Map coordinates refer to NZTopo50 map series. B, Schematic cross-section through the Matakitaki foreland thrust system illustrating the geometry of contacts between the fault-bound blocks (after Cutten Citation1987; Campbell Citation1992).
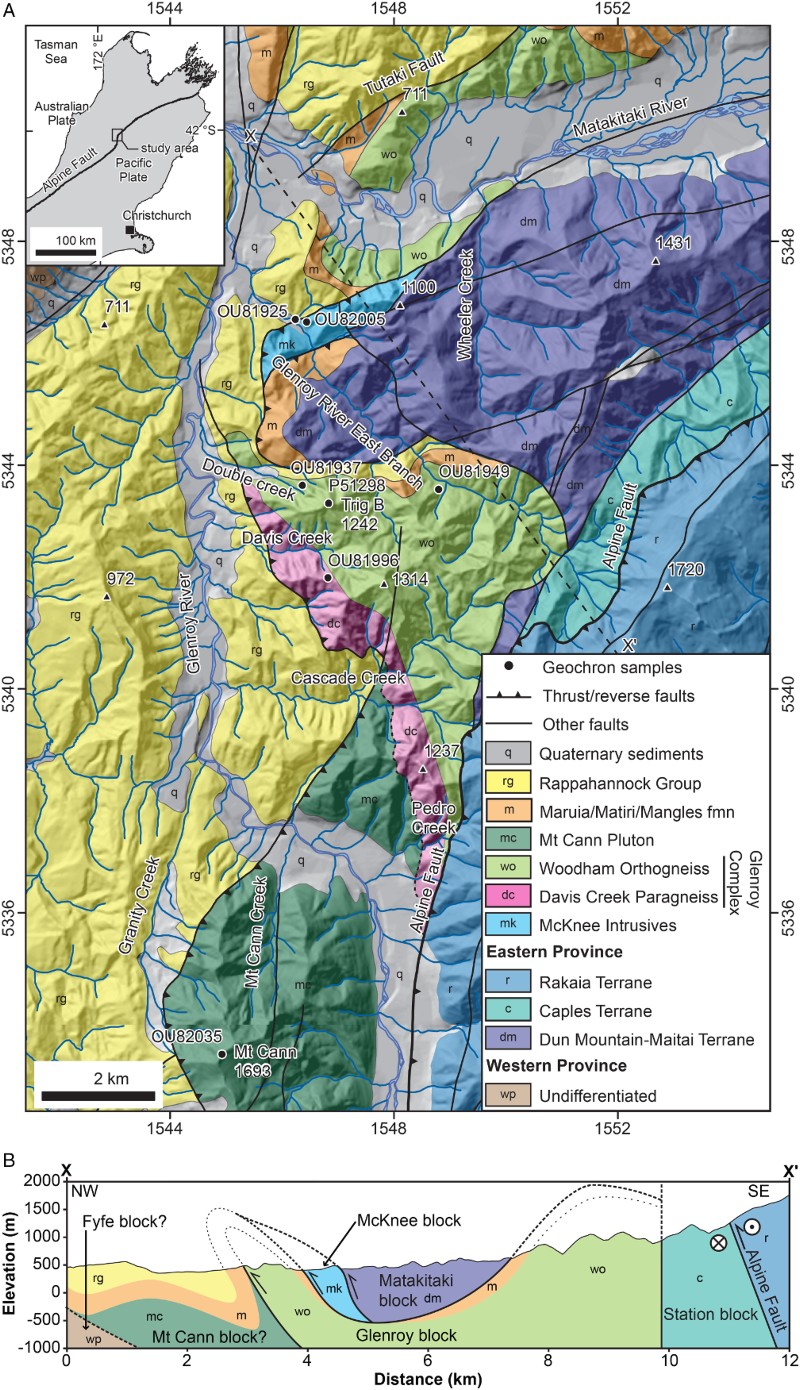
Western Province
The Western Province is dominated by Late Cambrian–Ordovician sedimentary and volcanic rocks, but high-grade metaplutonic and metasedimentary gneisses occur at scattered locations throughout (Nathan et al. Citation2002; Turnbull et al. Citation2010a). These gneisses were metamorphosed during the Late Devonian−Carboniferous and/or mid-Cretaceous (Ireland & Gibson Citation1998; Hiess et al. Citation2010; Sagar & Palin Citation2011; Scott et al. Citation2011b). Several granitic batholiths were intruded into the Western Province during the mid-Paleozoic and mid-Cretaceous (Tulloch Citation1988; Muir et al. Citation1994; Waight et al. Citation1997; Tulloch et al. Citation2009).
Median Batholith
Plutonic rocks ranging from gabbro to granite dominate the Median Batholith (Mortimer et al. Citation1999b). The Median Batholith can be subdivided into outboard (eastern) and inboard (western) parts (Tulloch & Kimbrough Citation2003; Allibone et al. Citation2009a; Scott Citation2013). The Outboard Median Batholith (OMB) includes all plutonic rocks between the Eastern and Western provinces and those that have intruded the western margin of the Eastern Province (Brook Street Terrane). Unmetamorphosed Late Triassic–Early Cretaceous I-type plutons dominate the OMB (Kimbrough et al. Citation1994; Muir et al. Citation1998; Mortimer et al. Citation1999a; Tulloch & Kimbrough Citation2003; Allibone et al. Citation2009a; Scott et al. Citation2009b). In contrast, the Inboard Median Batholith (IMB), which includes only those plutonic rocks intruded into the Western Province, is dominated by Early Cretaceous granulite facies orthogneisses but also includes Late Cambrian–mid-Cretaceous plutonic rocks (Allibone et al. Citation2009b).
Petrogenetic plutonic suites
The plutonic rocks making up the Median Batholith and batholiths of the Western Province are subdivided on the basis of their geochemistry and age into petrogenetic suites (Tulloch Citation1983, Citation1988; Allibone et al. Citation2009a; Tulloch et al. Citation2009; McCoy-West et al. Citation2014). As this paper addresses the affinity of plutonic rocks in the Glenroy area, it is necessary to provide some details about the relevant petrogenetic suites: Darran, Western Fiordland Orthogneiss (WFO) and Separation Point (SPS) suites.
The Darran Suite includes all subduction-related I-type LoSY (Sr/Y <40) (Tulloch & Kimbrough Citation2003) gabbroic–granitic rocks emplaced along the paleo-Pacific Gondwana margin between c. 230 Ma and 128 Ma (Allibone et al. Citation2009a). McCoy-West et al. (Citation2014) reassigned the isotopically primitive Late Permian–Early Permian gabbroic–dioritic rocks of the easternmost Median Batholith to the new Longwood Suite.
The SPS encompasses all mid-Cretaceous (c. 126–109 Ma) plutonic rocks in the Median Batholith with adakitic/HiSY (high Sr/Y; Sr/Y > 40) geochemistry, which is interpreted to indicate partial melting of a garnetiferous mafic igneous source rock (Tulloch & Rabone Citation1993; Muir et al. Citation1995; Tulloch & Kimbrough Citation2003). The WFO is included in the SPS on this basis. The WFO is a large composite plutonic body exposed over c. 3000 km2 in western Fiordland, which consists of numerous concordant intrusions emplaced between 126 and 116 Ma. The composition of the WFO ranges from ultramafic to monzonitic, although two-pyroxene dioritic–monzodioritic rocks are characteristic (Oliver Citation1977, Citation1980; Bradshaw Citation1990; Allibone et al. Citation2009b; Turnbull et al. Citation2010a). Allibone et al. (Citation2009b) subdivided the WFO into several plutons. However, the geochemistry of several WFO plutons (e.g. Malaspina and Worsley plutons) largely overlaps. The WFO plutons are therefore not differentiated on geochemical plots presented in this paper. In contrast to the WFO, the rest of the SPS is dominated by discrete, massive granodiorite–granite plutons intruded into the middle–upper crust between 126 and 109 Ma, and these lack post-magmatic metamorphic assemblages and layering (Tulloch Citation1983, Citation1988; Kimbrough et al. Citation1993; Kimbrough et al. Citation1994; Muir et al. Citation1994, Citation1998; Tulloch & Challis Citation2000; Scott & Palin Citation2008; Allibone et al. Citation2009a).
Subtle geochemical differences can be used to distinguish the WFO from the rest of the SPS (Allibone et al. Citation2009b). For example, the WFO has lower Na2O than the rest of the SPS at equivalent values of SiO2. Given these differences and the distinctive WFO rock types, the WFO is treated as a sub-group within the SPS, and the two are differentiated on geochemical variation diagrams.
Glenroy area
The Big Bend section of the Alpine Fault runs through the middle and upper Glenroy River valley along the west side of the Nardoo-Emily Peaks range (A). Shortening across the plate boundary due to convergence of the Pacific and Australian plates and the restraining nature of the Big Bend (Mann Citation2007) is manifest in the Glenroy area by development of a series of east-dipping imbricate fault blocks and large-scale folding of strata in the adjacent Maruia Basin (A, B; Cutten Citation1979; Campbell & Cutten Citation1985; Campbell Citation1992; Tulloch & Campbell Citation1993). Matakitaki foreland thrust system, named after the nearby Matakitaki River and ‘Bends foreland thrust system’ (Campbell Citation1992), is proposed as the name of the fold–thrust structure adjacent to the Alpine Fault Big Bend.
The Matakitaki foreland thrust system can be divided into six fault-bound blocks (B), which from east to west are: the Station, Matakitaki, McKnee, Glenroy, Mt Cann and Fyfe blocks (Campbell Citation1992; Tulloch & Campbell Citation1993). The easternmost block was tectonically emplaced first and the westernmost more recently (Campbell Citation1992; Tulloch & Campbell Citation1993). The Glenroy Complex, together with the lower Rappahannock Group (Frog Flat Formation, Middle Miocene), form the middle unit in the thrust system called the Glenroy block (B; Cutten Citation1987; Campbell Citation1992; Tulloch & Campbell Citation1993). In the middle section of the Glenroy River catchment, the Glenroy block structurally overlies Mt Cann Pluton monzodioritic rocks of the Mt Cann block (B). The Mt Cann block has in turn been thrust over metamorphic rocks (e.g. marble & amphibolite) of Western Province Takaka Terrane affinity in the Fyfe block (B; Cutten Citation1987; Campbell Citation1992). The Glenroy Complex is separated from the Matakitaki block in the lower Glenroy River East Branch by a fault-bound sliver of granitic rocks (McKnee Intrusives) which form the McKnee block (B). Rocks of the Dun Mountain–Maitai and Caples terranes make up the Matakitaki and Station (new name after Station Creek; formerly Caples block) blocks, respectively. Further north, the Glenroy Complex is inferred to be in contact with Jurassic layered mafic intrusives of the Rotoroa Complex (Rattenbury et al. Citation2006).
Glenroy Complex
Two formations comprise the Glenroy Complex: Davis Creek Paragneiss and Woodham Orthogneiss. The Davis Creek Paragneiss is dominated by quartzofeldspathic gneiss, which is intercalated with subordinate hornblende–biotite–plagioclase (referred to as hornblende gneisses in the following), biotite–muscovite–garnet–plagioclase (micaceous gneisses) and suspected meta-igneous granitic gneisses. The Appendix contains details of the crystalline rock types and stratigraphic nomenclature in the Glenroy area. The Woodham Orthogneiss ranges in composition from plagioclase hornblendite to biotite granite, but hornblende–biotite monzodioritic orthogneisses predominate. Orthopyroxene and clinopyroxene have been partially replaced by hornblende and quartz, and ilmenite is rimmed by titanite in hornblende–biotite orthogneisses (A, B). This suggests that the hornblende–biotite orthogneisses are the retrogressed equivalents of two-pyroxene–hornblende orthogneisses.
Figure 2. Plane-polarised light photomicrographs of (A, B) Woodham Orthogneiss hornblende-biotite monzodioritic orthogneiss and (C, D) McKnee Intrusives quartz monzodiorite (OU82005). A, Partial replacement of clinopyroxene and orthopyroxene by hornblende, biotite and quartz (OU81943). B, Incomplete replacement of ilmenite by titanite. Note the green-blue rims and green-brown cores of the hornblende (OU81910). C, Partial magmatic replacement of clinopyroxene by hornblende, which has in turn been partially altered to biotite. D, Textural evidence for sub-solidus replacement of biotite by muscovite, chlorite and epidote. Mineral abbreviations follow Whitney & Evans (Citation2010).
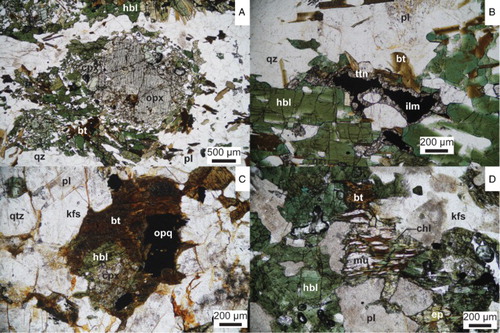
Sills of Woodham Orthogneiss have concordantly intruded the Davis Creek Paragneiss in Davis Creek (E1545728/N5343399) and on the west-facing slopes below Trig B (spot height 1242 m; E1545728/N5343399). The Davis Creek Paragneiss is therefore interpreted as a fragment of country rock into which the Woodham Orthogneiss magmas were intruded. The contact between the Davis Creek Paragneiss and the Mt Cann Pluton is shown as an intrusive contact by Rattenbury et al. (Citation2006). However, earlier maps (e.g. Campbell Citation1992) show the same contact as a thrust fault. Although this boundary fault was not observed during field mapping, the latter interpretation is favoured even though it may originally have been intrusive, and the contact is tentatively shown as a fault (A). Granitic (sensu lato) rocks mapped as Separation Point Suite hornblende–biotite granite (Rattenbury et al. Citation2006) (here Mt Cann Pluton) in the middle Cascade Creek catchment are considered part of the Davis Creek Paragneiss based on their unusually high quartz and feldspar content for magmatic rocks, lack of epidote, gneissic texture and LoSY (low Sr/Y) geochemistry ().
Table 1. Summary of the U–Pb zircon ages, suite affinity and whole-rock major- and trace-element data for representative samples of the Glenroy Complex, McKnee Intrusives and Mt Cann Pluton. Mineral abbreviations follow Whitney & Evans (Citation2010).
McKnee Intrusives
Two rock types make up the McKnee Intrusives: coarse-grained hornblende–biotite quartz monzodiorite (OU81925, 82004 and 82005) and medium-grained biotite granodiorite (OU81941). Hornblende–biotite quartz monzodiorite crops out north of the Glenroy River East Branch, while biotite granodiorite is exposed south of the river. The nature of the contact between the two rock types is uncertain. In the quartz monzodiorites, primary clinopyroxene has been mostly replaced by hornblende which in turn has been partially replaced by biotite (C, D). Biotite is partially altered to muscovite, chlorite and epidote (C, D). The replacement of clinopyroxene by hornblende and hornblende by biotite are considered to be a magmatic phenomenon, while alteration of biotite, Fe-Ti oxides and plagioclase is considered to have occurred under sub-solidus (greenschist facies) conditions. The McKnee Intrusives retain a magmatic interlocking texture and no foliation is evident (C, D).
Mt Cann Pluton
Much of the Mt Cann range consists of massive coarse-grained porphyritic biotite−epidote quartz monzodiorite, and is the dominant lithology of the Mt Cann Pluton. This lithology consists of pink alkali-feldspar phenocrysts set in an inequigranular, fine- to medium-grained groundmass of biotite, plagioclase, quartz, epidote, alkali-feldspar, Fe-Ti oxides and titanite. Orbicular granite has been reported from boulders in Pedro Creek (Reid et al. Citation1972) and was also observed in this study as boulders in Mt Cann Creek. The source of these boulders has not been located, but it is inferred to be the Mt Cann Pluton (Reid et al. Citation1972).
Analytical methods
U–Pb zircon dating by laser-ablation inductively coupled plasma mass spectrometry (LA-ICP-MS) and isotope dilution thermal ionisation mass spectrometry (ID-TIMS) methods were undertaken at the Australian National University and University of California Santa Barbara, respectively. Whole-rock major- and trace-element data were obtained by the X-ray fluorescence (XRF) and ICP-MS methods, respectively. A detailed description of all the analytical methods can be found in Summary S1. The details of all analysed samples and the full set of whole-rock geochemical data can be found in the online PETLAB database, which is accessible at http://pet.gns.cri.nz/. summarises the results of U–Pb zircon dating and provides whole-rock geochemistry of representative samples from each unit. The complete U–Th–Pb zircon datasets are provided in Tables S1–S7.
U–Pb zircon geochronology
Woodham Orthogneiss
Two-pyroxene–hornblende monzodioritic orthogneiss (OU81949)
This sample was chosen because it retains an interlocking magmatic texture and has a typical monzodioritic composition. Zircons from the sample range from prismatic euhedral grains to equant anhedral/rounded crystals and exhibit weak oscillatory and broad compositional zoning, sometimes overprinted by patchy zoning (A). One zircon comprises an inherited euhedral prismatic core (#33, 133 ± 4 Ma). Faint oscillatory zoning in the core appears to have been overprinted by sector zoning, which traverses the boundary with the magmatic zircon overgrowth (#34, 123 ± 4 Ma) (A). No textural evidence for dissolution-precipitation or in situ modification of zircon is observed (A), suggesting magmatic zircon was unaffected by subsequent metamorphism.
Figure 3. Cathodoluminescence images of zircon (left column), and combination stacked frequency histograms and Gaussian-summation probability density distribution plots of 206Pb*/238U zircon dates (right column) for: A–D, Woodham Orthogneiss (OU81949 and OU81937); E–H, McKnee Intrusives quartz monzodiorites (OU81925 and OU82005); I, J, Mt Cann Pluton quartz monzodiorite (OU82035). Asterisk indicates date is part of magmatic population.
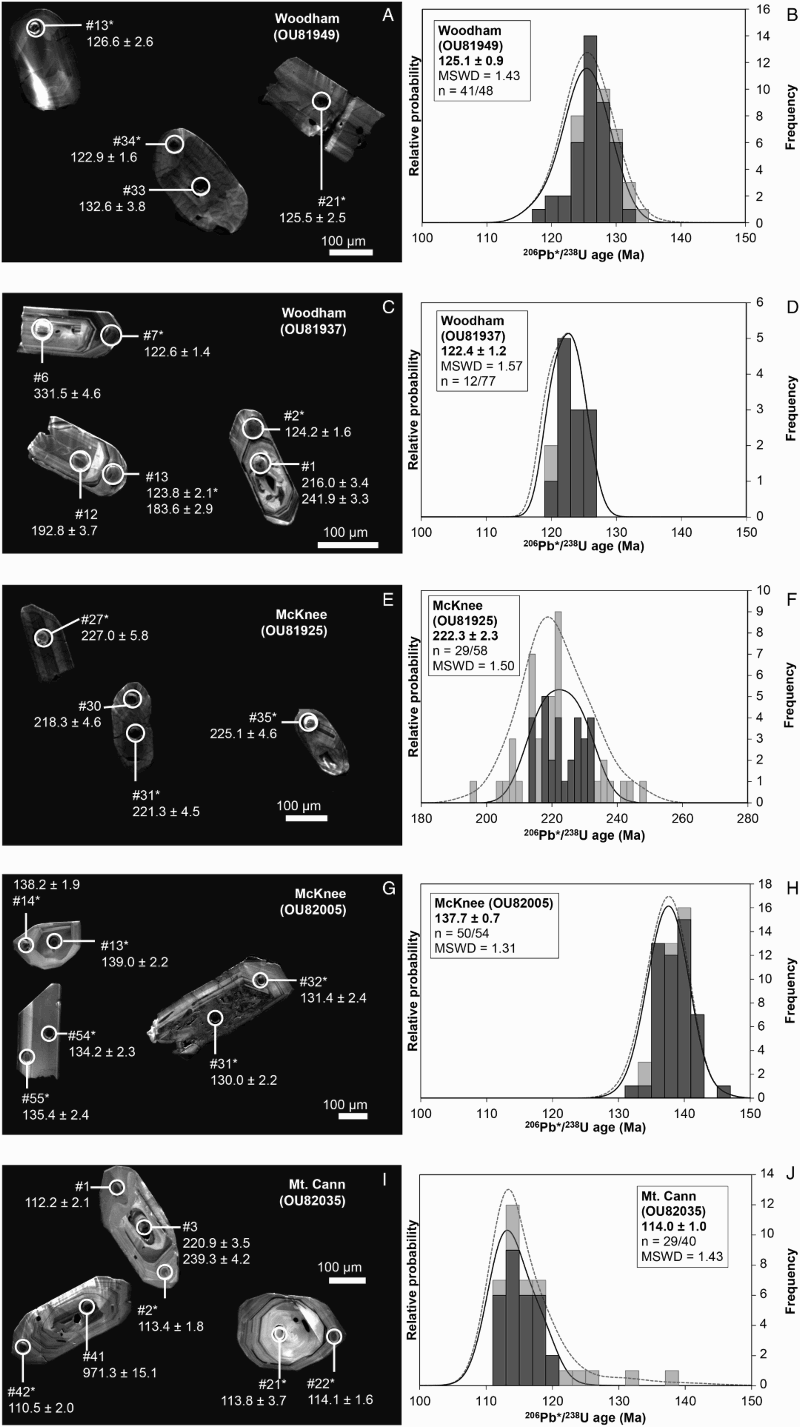
All 48 dates make up a single peak with a normal distribution and an error-weighted mean age of 125.4 ± 0.9 Ma (mean squared weighted deviation, MSWD = 1.57) (B). Five discordant and the two oldest concordant dates were excluded on the basis that they are likely inherited, giving improved error-weighted mean and Tera-Wasserburg concordia ages of 125.1 ± 0.9 Ma (MSWD = 1.43, n = 41/48) and 126.6 ± 1.3 Ma (n = 16/48; probability of concordance, P = 0.94), respectively (B). All but one of the dates have Th/U values of > 0.5, which is characteristic of magmatic zircon (Hoskin & Schaltegger Citation2003). The concordia age (126.6 ± 1.3 Ma) is therefore taken as the magmatic age of this sample.
Hornblende–biotite monzodioritic orthogneiss (P51298)
This sample contains partially altered ortho- and clinopyroxene and was selected for dating to assess, using the precise ID-TIMS method, whether amphibolite facies metamorphism had affected the U–Pb isotope system in zircon. The two multiple-grain zircon size fractions (<100 μm and > 100 μm) both yield concordant ages that agree within error (Table S2), which suggests that the U–Pb isotope system has not been affected by metamorphism. Together these analyses give an error-weighted mean age of 122.96 ± 0.35 Ma (MSWD = 0.003), which is considered to be the magmatic age of this sample.
Biotite monzogranitic orthogneiss (OU81937)
Sample OU81937 was chosen for dating to ascertain whether the felsic lithologies were intruded at the same time as their intermediate counterparts. Although zircons are mostly euhedral prisms, they typically comprise relict cores which show a range of textures, overgrown by oscillatory zoned zircon (C). Seventy-seven dates from 64 spot analyses fall within the range 369–118 Ma, but Carboniferous (c. 340 Ma) and Cretaceous (c. 122 Ma) age populations are dominant (D). Thirteen dates are Cretaceous, twelve of which define a single normally distributed population (MSWD = 1.57) with an error-weighted mean age of 122.4 ± 1.2 Ma (D). Cretaceous ages have Th/U ratios of c. 0.24–0.55 but the oscillatory zoning indicates a magmatic rather than metamorphic origin. Older zircon is considered to be inherited, which is consistent with the observed core-overgrowth textures (C). A Tera-Wasserburg concordia age of 121.8 ± 1.1 Ma (MSWD = 0.12; P = 0.72) is calculated from the nine concordant analyses in the mid-Cretaceous population, and is the preferred magmatic age.
Davis Creek Paragneiss
To assess the timing of metamorphism and possible affinity and provenance of the metasedimentary rocks making up the Davis Creek Paragneiss, a sample of biotite–muscovite–garnet quartzofeldspathic gneiss (OU81996) was collected from near the contact with the Woodham Orthogneiss. OU81996 contains zircons that are anhedral–subhedral and equant–prismatic in morphology (A). Most of the zircons comprise a pale, low-U, variously zoned, partially corroded core mantled by dull, commonly irregularly shaped, pitted overgrowths (A). There is a spread of ages over the range c. 117–365 Ma (n = 73; B). Five main zircon age groups were identified using the Unmix function in IsoPlot (Sambridge & Compston Citation1994), of which the mid-Cretaceous (c. 120 Ma) and Triassic (c. 240 Ma) are the largest. The Triassic group comprises two populations (B). Twenty-two dates ranging from 234 Ma to 251 Ma give a pooled age of 241.8 ± 2.0 Ma (MSWD = 1.60) for the major peak in the Triassic group. An error-weighted mean age of 227.1 ± 2.5 Ma is calculated for the younger subordinate population in the Triassic group from nine dates ranging over 218–230 Ma. All of the Triassic dates have Th/U ratios of > 0.5. The mid-Cretaceous group consists of 22 dates from a single population that were obtained solely from the overgrowths, and yield a pooled age of 120.1 ± 0.9 Ma (B). Fourteen of the mid-Cretaceous dates yield a Tera-Wasserburg concordia age of 120.6 ± 0.8 Ma (MSWD = 0.0014; P = 0.97) that is indistinguishable from the pooled age (C). All of the mid-Cretaceous dates are from zircon that lack oscillatory zoning, and only three have Th/U values > 0.1 (A, D).
Figure 4. Davis Creek Paragneiss quartzofeldspathic gneiss (OU81996). A, Cathodoluminescence images of representative zircons. Asterisk indicates that the age is part of the metamorphic population. B, Combination stacked frequency histograms and Gaussian-summation probability density distribution plot. P (1/20) is the probability that a zircon population representing 5% of the zircons in the rock would be missed when analysing 76 grains at random (Dodson et al. Citation1988). C, Tera-Wasserburg concordia plot showing the 14 concordant analyses from the metamorphic population. Error ellipses are shown at the 1σ level. P = probability of concordance. Error on concordia age is 2σ. D, Th/U versus 206Pb*/238U age plot. The dashed line marks the minimum Th/U ratio (0.5) of typical magmatic zircon (Hoskin & Schaltegger Citation2003).
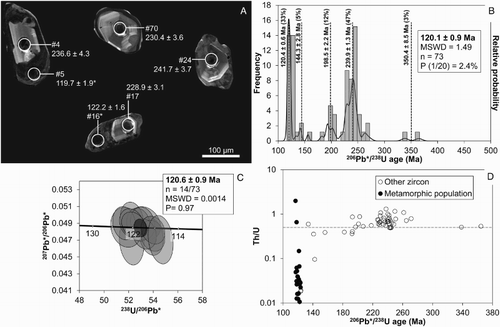
McKnee Intrusives
Hornblende–biotite quartz monzodiorite (OU81925)
Zircons from OU81925 have euhedral stubby prismatic morphologies with oscillatory zoning typical of magmatic granitoid zircon (E; Corfu et al. Citation2003). All but 1 of the 58 analyses from both the interior and exterior of grains yielded Late Triassic dates, comprising a unimodal population with an error-weighted mean age of 220.3 ± 2.7 Ma (MSWD = 4.08). Twenty-nine of the 58 dates give an error-weighted mean age of 222.3 ± 2.3 Ma with a MSWD value of 1.50 (F). Fourteen dates yield a conventional concordia age of 225.0 ± 2.7 Ma (MSWD = 2.1; P = 0.15). This is considered to be the best estimate of the magmatic age of this rock.
Hornblende–biotite quartz monzodiorite (OU82005)
Most zircons separated from OU82005 are euhedral stubby–elongate prisms that exhibit a range of compositional zoning patterns. Broad, oscillatory and sector zoning are most common (G). All 54 ages fall within the range 145–131 Ma and have a Gaussian distribution (MSWD = 1.35) with an error-weighted mean age of 137.6 ± 0.7 Ma, indicative of a single magmatic population. Excluding four dates that are more than 10% discordant yields a pooled age of 137.7 ± 0.7 (MSWD = 1.31) (H). A Tera-Wasserburg concordia age of 137.8 ± 0.7 Ma (MWSD = 0.18; P = 0.67) is given by 33 spot analyses and is considered to be the best estimate of the magmatic age.
Mt Cann Pluton
Zircons from Mt Cann Pluton sample OU82035 are euhedral prisms with well-developed oscillatory zoning, some of which comprise a relict core (I). Forty of the 48 dates obtained for the Mt Cann Pluton are Cretaceous. The remaining eight dates (c. 230 Ma, 300 Ma, 1000 Ma and 2600–2300 Ma) were acquired from distinct cores of zircons and are interpreted as inherited. The Cretaceous ages range over 138–110 Ma and define a positively skewed distribution (MSWD = 2.79). By removing eleven of the Cretaceous dates deemed to be inherited and those greater than 10% discordant, the remaining 29 dates yield an error-weighted mean age of 114.0 ± 1.0 Ma (MSWD = 1.43) (J). The Tera-Wasserburg concordia age of 113.8 ± 1.0 Ma (n = 19/48, MSWD = 0.84; P = 0.36) is taken as the crystallisation age.
Whole-rock geochemistry
Representative samples of the various petrogenetic suites that have similar SiO2 contents to the samples to which they are being compared are used on the chondrite-normalised REE plots. La/LuN is the ratio of La to Lu, where N indicates the values are chondrite-normalised (Sun & McDonough Citation1989). Eu/Eu* is the ratio of the chondrite-normalised measured Eu abundance to the predicted chondrite-normalised abundance, calculated as the mean of SmN and GdN.
Glenroy Complex
Woodham Orthogneiss
TiO2, Fe2O3t (total Fe oxide as Fe2O3), MnO, MgO, CaO and P2O5 decrease with increasing SiO2. In contrast, K2O increases with SiO2 while the trends of Al2O3 and Na2O are characterised by their hump shape with peaks at c. 58 wt% SiO2 (A, B).
Figure 5. Whole-rock geochemical variation diagrams of the Woodham Orthogneiss compared to the Arthur River Complex (ARC), Separation Point Suite (SPS) and Western Fiordland Orthogneiss (WFO): A, Al2O3 versus SiO2; B, Na2O versus SiO2; C, Rb versus SiO2; D, log(Sr/Y) versus Y; E, chondrite-normalised (Sun & McDonough Citation1989) rare Earth element (REE) plot; F, stacked histogram of Sr/Y ratios. Main data sources: Bradshaw & Kimbrough (Citation1991); Challis et al. (Citation1994); Burgess (Citation2004); Blattner (Citation2006); Scott & Palin (Citation2008); Allibone et al. (Citation2009a); Allibone et al. (Citation2009b); Scott et al. (Citation2009a); Turnbull et al. (Citation2010b); Price et al. (Citation2011); Tulloch et al. (Citation2011); Daczko et al. (Citation2012); McCoy-West et al. (Citation2014). *Percentage of total WFO, including the Woodham Orthogneiss. **Other WFO excludes the atypical Breaksea Orthogneiss and Hawes Head Ultramafics.
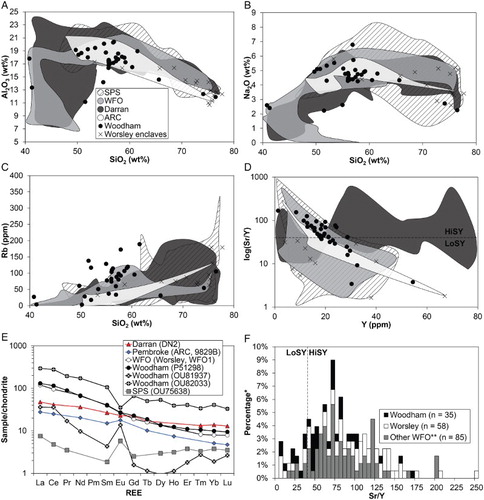
Most trace elements show less well-defined trends on Harker diagrams compared to the major elements. Y content ranges over 1–54 ppm, and shows no discernible trend with SiO2. As SiO2 increases, Sr (which has a wide range over 103–1835 ppm) trends towards lower values. On the other hand, Rb (C), Zr and Ba are positively correlated with SiO2. The Sr/Y ratio is negatively correlated with Y (D). Despite a wide range in Sr/Y values of 3–167, 74% of samples (n = 26/35) have Sr/Y ratios > 40 (e.g. P51298) and therefore classify as HiSY (Tulloch & Kimbrough Citation2003). Of the LoSY (Sr/Y < 40) samples, 9% (n = 3/35) have Sr/Y ratios of < 20 and 17% (n = 6/35) have ratios between 20 and 40.
The rare Earth element (REE) patterns typically lack Eu anomalies and are steep (La/LuN > 10) due to low heavy rare Earth element (HREE) concentrations, which for Lu range over 8–15 times chondritic values (E). La abundances have a large range of 57–180 times chondrite. Three samples have distinctive REE patterns. OU82032 and OU82033 have pronounced negative Eu anomalies (Eu/Eu* = 0.29–0.41), high REE abundances and relatively shallow trends (La/LuN = 9–10). The REE pattern of OU81937 is characterised by low REE concentrations (LaN = 36; LuN = 2.7) and its saddle-shape due to low middle-HREE abundances (DyN = 0.95) and large positive Eu anomaly (Eu/Eu* = 4.6).
Davis Creek Paragneiss
The quartzofeldspathic gneisses plot in the intermediate–felsic igneous and quartzose sedimentary fields on the F1–F2 plot (A; Roser & Korsch Citation1988). On the ACNK (Al-Ca*+Na-K, where * indicates apatite correction applied) triangle (Fedo et al. Citation1995), the quartzofeldspathic gneisses plot in a horizontal array above the Ab-Or tie line (B) and Zr/Sc ratios trend towards values similar to and greater than dacite and rhyolite (C). Positive Pb, Hf and Zr and negative P anomalies characterise the primitive mantle (PM) normalised trace-element diagram (spidergram) pattern (D).
Figure 6. Whole-rock geochemical variability of the Davis Creek Paragneiss compared to mean volcanic rock compositions, and average upper crust (McLennan Citation2001). A, F1–F2 discriminant function diagram (Roser & Korsch Citation1988). B, A–CN–K triangle (Fedo et al. Citation1995). *Apatite-correction applied (CaO = Ca – 1.67P). C, Th/Sc–Zr/Sc bivariate diagram (after Roser & Korsch Citation1999). D, Primitive mantle (PM) (Sun & McDonough Citation1989) normalised trace-element spider diagram. A, andesite; CAB, calc-alkaline basalt; D, dacite; R, rhyolite. Mineral abbreviations are those of Whitney & Evans (Citation2010).
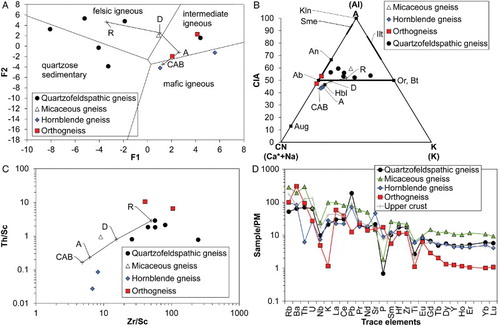
The suspected meta-igneous rocks plot in the intermediate igneous field on the F1–F2 plot, on the A–CN line in the ACNK triangle, and exhibit positive Pb and negative P anomalies on the spidergram (A–D). The pronounced negative K anomaly on the spidergram (D) and relatively high CaO () are distinctive features of these rocks.
The hornblende gneisses have similar Zr/Sc ratios to calc-alkaline basalt and andesite (C). However, their Th/Sc ratios differ from those of calc-alkaline basalts and andesites (C). The Sr/Y ratios range from 49 to 77. The positive Sr anomaly on the spidergram and the elevated HREE reflect the abundance of plagioclase and hornblende, respectively. On the ACNK triangle the hornblende gneisses plot near andesite/hornblende (B), and on the F1–F2 diagram they plot in the mafic igneous field (A).
A pronounced negative P anomaly and elevated HREE characterises the spidergram pattern of the micaceous gneiss (D). On the ACNK triangle this sample plots in a position near the Ab-Or tie-line (B). The micaceous gneiss plots near the intermediate–felsic igneous boundary on the F1–F2 plot (A), and has similar Th/Sc and Zr/Sc ratios to andesite/dacite (C).
McKnee Intrusives
Because only one Late Triassic McKnee Intrusives sample was analysed, the following description of geochemical trends relates exclusively to Early Cretaceous samples of the McKnee Intrusives. All of the major-element oxides are negatively correlated with SiO2 (e.g. A, B), except K2O which shows a positive correlation. Rb, Zr and Ba show weak positive correlations with SiO2, whereas the negative trends of Sr and Y are stronger (C, D). The Early Cretaceous samples have a restricted range of Sr of 396–453 ppm. The abundance of Y in the intermediate-composition Early Cretaceous samples also has a narrow range (15–16 ppm), but is only 6 ppm in the biotite granodiorite (OU81941). The Sr/Y ratios of the Cretaceous samples range from 26–73; the highest value is from the biotite granodiorite. The Late Triassic sample has a Sr/Y ratio of 68. The REE patterns of the Early Cretaceous samples are relatively flat (La/LuN = 7–11) and characterised by minor Eu anomalies (Eu/Eu* = 0.68–0.98) (E), while that of the Late Triassic sample is relatively steep (La/LuN = 16) (F).
Figure 7. Whole-rock geochemical variation diagrams showing the McKnee Intrusives and Mt Cann Pluton compared to the Separation Point Suite, Rahu Suite and Darran Suite: A, Al2O3 versus SiO2; B, Na2O versus SiO2; C, Sr versus SiO2; D, Y versus SiO2; E, F, Chondrite-normalised REE plots. Data sources in addition to those listed for : Graham & White (Citation1990); Muir et al. (Citation1997); Waight et al. (Citation1998); Sagar & Palin (Citation2011). TR, Triassic; K, Cretaceous.
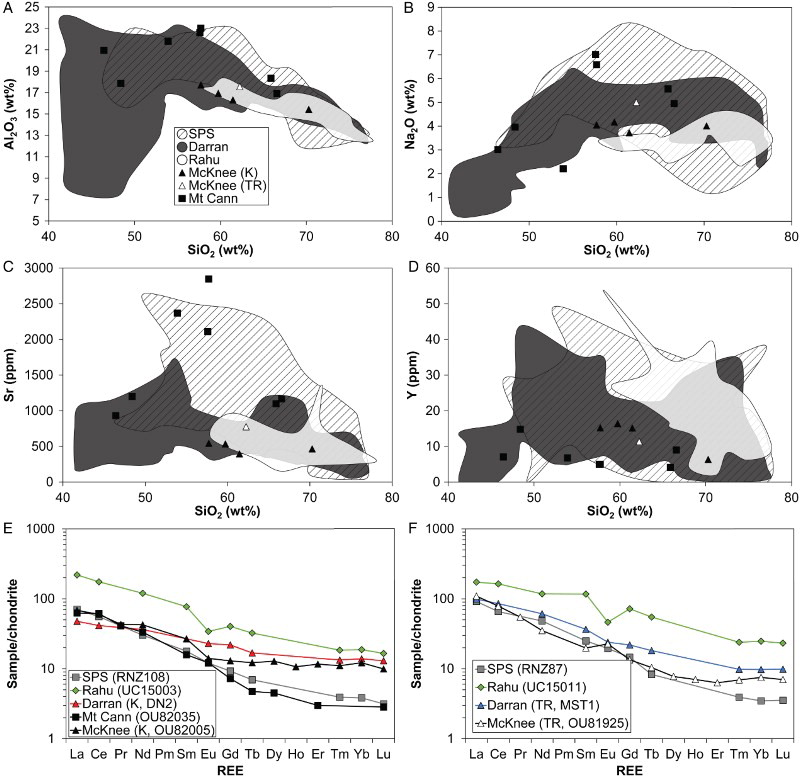
Mt Cann Pluton
TiO2, Al2O3, Fe2O3t, MnO, MgO and P2O5 decrease with increasing SiO2 in the Mt Cann Pluton (A). There is no clear correlation between K2O and SiO2. A hump-shaped trend with a maximum at c. 57 wt% SiO2 characterises the Na2O Harker diagram (B).
The trends shown by trace elements when plotted against SiO2 are weak or non-existent. Sr shows no overall correlation with SiO2, and ranges from 932 ppm to 2847 ppm (C). Rb, Zr and Ba generally increase with SiO2, while Y is consistently low (4–15 ppm) and decreases (D). Sr/Y of all samples is > 40, and ranges from 81–569 ppm. The REE pattern of the type specimen (OU82035) is characterised by the lack of an Eu anomaly and low HREE concentrations (LuN = 2.8), giving it a steep slope (La/LuN = 22) (E). Two samples (OU81953 and OU82039) have positive Eu anomalies (Eu/Eu* = 1.1–1.7).
Petrogenetic suite assignment
Woodham Orthogneiss
On many Harker diagrams the Woodham Orthogneiss is indistinguishable from the SPS (including WFO), Darran Suite and ARC (A–C). However, the negative trend on the log(Sr/Y) versus Y diagram (Defant & Drummond Citation1990) is similar to and overlaps that of the SPS but is different to the Darran Suite and ARC, the former of which shows no obvious trend (D). Moreover, the majority (74%) of the samples classify as HiSY, which is a defining characteristic of the SPS (Tulloch & Kimbrough Citation2003). In contrast, only 55% of the Darran Suite have Sr/Y ratios > 40. The typically steep REE patterns (La/LuN > 10) are also similar to that of the SPS (E), which has a mean La/LuN ratio of c. 14. In contrast, the Darran Suite and ARC have shallow REE trends (E) and mean La/LuN ratios of only 4 and 5, respectively. These geochemical ratios and trends suggest the Woodham Orthogneiss is best correlated with the SPS.
Despite their many geochemical similarities, there are some differences between the Woodham Orthogneiss and SPS. Firstly, Rb ranges to higher values (C), which may be due to the paucity of data for WFO hornblende–biotite orthogneisses (see table 4 in Allibone et al. Citation2009b) that dominate the Woodham Orthogneiss. If this is the case, it suggests amphibolite facies retrogression was not isochemical. Alternatively, it may indicate a different petrogenesis for the magmas such as melting or assimilation of a relatively fertile or hydrous rock. Secondly, the HREE abundances are greater than those of the SPS (E). This may be explained by relatively low degrees of partial melting of the source rock. Thirdly, the REE patterns of OU82032, OU82033 and OU81937 are distinct from those of not only the SPS, but also the majority of the Woodham Orthogneiss (E). The concave-up shape of the OU81937 pattern is indicative of either residual hornblende in the source rock or hornblende fractionation. The SPS West Arm Leucogranite and WFO sample FM-90B also shows similar patterns (E) (McCulloch et al. Citation1987; Scott et al. Citation2011a). The negative Eu anomaly and relative enrichment in HREE/shallow slope characterising the OU82032 and OU82033 REE patterns (E) suggests removal of plagioclase and a garnet-free source rock, respectively. The geochemistry of these three samples is also atypical in other respects. For example, their relatively high SiO2 and Y and low Al2O3, Rb and Sr/Y, is shared by some granitic enclaves in the WFO Worsley Pluton (A, C, D) (Bradshaw & Kimbrough Citation1991). These three samples may therefore also be enclaves within or intrusions with a different petrogenesis to the bulk of the Woodham Orthogneiss. They also are volumetrically (c. 1%) very minor compared to the typical rocks, and therefore should not be used to assess the affinity of the Woodham Orthogneiss.
The magmatic ages of the monzodioritic (OU81949, 126.6 ± 1.3 Ma; P51298, 122.99 ± 0.32 Ma) and granitic (OU81937, 121.8 ± 1.1 Ma) samples are indistinguishable (within uncertainty) from some WFO and Fiordland SPS plutons (A) (Gibson et al. Citation1988; Muir et al. Citation1998; Tulloch & Kimbrough Citation2003; Hollis et al. Citation2004; Klepeis et al. Citation2004; Allibone et al. Citation2009b), but fall outside the error limits of dated Darran Suite and ARC samples (Kimbrough et al. Citation1994; Hollis et al. Citation2003; Klepeis et al. Citation2004; Marcotte et al. Citation2005; Tulloch et al. Citation2011). The geochronology therefore corroborates a geochemical correlation with the SPS.
Figure 8. Plots showing the age ranges of A, Woodham Orthogneiss (OU81949, OU81937 and P51298) and WFO and Fiordland SPS plutons; B, Late Triassic McKnee Intrusives (OU81925) and Darran Suite plutons; C, Early Cretaceous McKnee Intrusives (OU82005) and Darran Suite plutons; D, Mt Cann Pluton (OU82035) and Nelson and Fiordland SPS and Rahu Suite plutons. Data sources: Kimbrough et al. (Citation1994); Muir et al. (Citation1994, Citation1997, Citation1998); Waight et al. (Citation1997); Blattner & Graham (Citation2000); Tulloch & Kimbrough (Citation2003); Hollis et al. (Citation2004); Klepeis et al. (Citation2004); Bolhar et al. (Citation2008); Scott & Palin (Citation2008); Allibone et al. (Citation2009a); Turnbull et al. (Citation2010b); Stowell et al. (Citation2014).
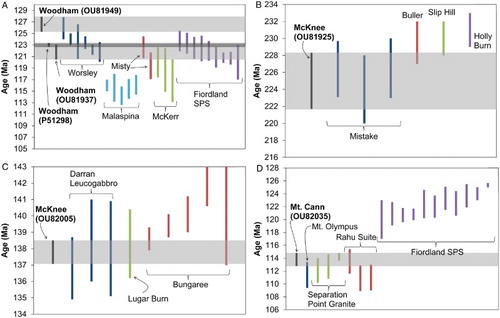
The rock types comprising the bulk of the Woodham Orthogneiss – two-pyroxene−hornblende and hornblende−biotite monzodioritic orthogneisses – are very similar to the WFO (Oliver Citation1977; Bradshaw Citation1989b, 1990; Allibone et al. Citation2009b). This indicates that, within the SPS, the Woodham Orthogneiss may be specifically correlated with the WFO. The magmatic ages obtained for the Woodham Orthogneiss overlap those of the Worsley and Misty plutons and McKerr Intrusives (A). However, ages of ≥ 125 Ma have only been reported for the northern Fiordland Worsley Pluton (A). The age of 126.6 ± 1.3 Ma for sample OU81949 therefore allows the Woodham Orthogneiss to be even more specifically correlated with the northern part of the WFO. This is supported by the geochemistry of the Worsley Pluton which, based on published analyses ranges to lower Sr/Y values (minimum of < 5 v. 11), and comprises a higher percentage of LoSY rocks compared to the rest of the WFO (F). The location of some LoSY samples within the Worsley Pluton are shown on . The analysed Woodham Orthogneiss samples also range to low Sr/Y ratios (minimum of 3), and include a similar proportion of LoSY rocks to the Worsley Pluton (F). The inclusion of three samples in the Woodham Orthogneiss with geochemistry that is similar to Worsley Pluton enclaves (A–D; locations also shown on ) lends further support to this correlation. Additionally, the Woodham Orthogneiss commonly comprises intergrown Fe-Ti oxides that were used by Bradshaw (Citation1990) to distinguish the Worsley Pluton from the similar McKerr Intrusives and Lake Wade Diorite.
McKnee Intrusives
The McKnee Intrusives are mapped as part of the SPS (Rattenbury et al. Citation2006). However, the whole-rock geochemistry of the McKnee Intrusives is distinct from the SPS. At equivalent values of SiO2, the McKnee Intrusives have lower Al2O3, Na2O and Sr than the SPS, similar to the trend shown by the Darran Suite (A–D). The McKnee Intrusives and Rahu Suite trends are similar on Al2O3 and Sr Harker diagrams (A, C). However, on Na2O and Y Harker diagrams, the McKnee Intrusives and Rahu Suite diverge by c. 58–62 wt% SiO2; the McKnee Intrusives trend towards higher Na2O and lower Y values (B, D).
The REE patterns have shallow trends and minor Eu anomalies that are similar to those of the Darran Suite, which have La/LuN values of 10–13 (E, F). On the other hand, the REE patterns of the SPS are typically steeper (La/LuN = 22) and those of the Rahu Suite are characterised by more pronounced negative Eu anomalies (Eu/Eu* = 0.49–0.58) (E, F). Accordingly, the McKnee Intrusives are re-assigned to the Darran Suite.
The Late Triassic age (225.0 ± 2.7 Ma) for McKnee Intrusives sample OU81925 is the same, within uncertainty, as the Darran Suite Mistake and Slip Hill diorites in northern Fiordland and the related Buller Diorite near Lake Rotoiti, Nelson (B) (Kimbrough et al. Citation1994; Muir et al. Citation1998; Blattner & Graham Citation2000). There are also significant lithological similarities between the McKnee Intrusives, Mistake Diorite and Buller Diorite; all three units are composed of variably metamorphosed quartz-bearing diorites (Williams & Harper Citation1978; Bradshaw Citation1990; Johnston Citation1990; Blattner & Graham Citation2000). This suggests that the Late Triassic part of the McKnee Intrusives is a correlative of the Late Triassic Darran Suite plutons in northern Fiordland.
The U–Pb zircon age of 137.8 ± 0.7 Ma for McKnee Intrusives sample OU82005 overlaps the ages of several Darran Suite plutons (C) (Mattinson et al. Citation1986; Kimbrough et al. Citation1994; Muir et al. Citation1997, Citation1998; Tulloch & Kimbrough Citation2003; Turnbull et al. Citation2010b). Massive biotite leucogabbro and hornblende diorite, which shows magmatic replacement of pyroxene by hornblende that in turn has been altered to biotite, make up most of the Darran Leucogabbro (Blattner Citation1978; Bradshaw Citation1990; Blattner & Graham Citation2000). The lithological similarity and overlapping ages of the Early Cretaceous McKnee Intrusives and Darran Leucogabbro suggest they may be correlatives. Furthermore, the Early Cretaceous and Late Triassic parts of the McKnee Intrusives are in close proximity to each other in the same way as the Early Cretaceous Darran Leucogabbro and Late Triassic Darran Suite plutons in northern Fiordland () (Turnbull Citation2000).
Mt Cann Pluton
The most recent geological map covering the Glenroy area shows the Mt Cann Pluton as part of the SPS (Rattenbury et al. Citation2006). The Mt Cann Pluton is characterised by the high Al2O3, Na2O and Sr, and low Y geochemistry typical of the SPS (Tulloch & Rabone Citation1993; Muir et al. Citation1995), and clearly plots within the SPS field rather than the area defined by the Darran or Rahu suite on Harker diagrams (A–D). The REE pattern closely follows that of the Separation Point Granite, which is characteristically steep (La/LuN = 22) and lacks an Eu anomaly (E). In contrast, relatively shallow REE patterns are typical of the Darran (La/LuN = 4) and Rahu (La/LuN = 13) suites and, in the case of the latter, pronounced Eu anomalies (Eu/Eu* = 0.58) are common (E). The geochemical data corroborates assignment to the SPS.
LA-ICP-MS U–Pb zircon data are consistent with this. The magmatic age of the Mt Cann Pluton (113.8 ± 1.0 Ma) is indistinguishable, within error, from those of the Separation Point Granite and Mt Olympus Pluton in northwest Nelson (D) (Muir et al. Citation1994; Bolhar et al. Citation2008). Separation Point Suite plutons in the Fiordland region are generally older than their counterparts in northwest Nelson (Kimbrough et al. Citation1994; Muir et al. Citation1994, Citation1998; Bolhar et al. Citation2008; Scott & Palin Citation2008), and their ages do not overlap that of the Mt Cann Pluton (D). Together, the whole-rock geochemistry and age of the Mt Cann Pluton indicate it belongs to the SPS, and shows strong affinity to the Separation Point Granite.
Origin, timing of metamorphism and affinity of the Davis Creek Paragneiss
The high SiO2 content () and abundance of K-feldspar and quartz, and paucity of mafic minerals in the quartzofeldspathic gneisses suggest that their protoliths were sedimentary rocks. The zircon age spectra of sample OU81996 is consistent with a sedimentary origin because of the numerous age populations present (B). The position of the quartzofeldspathic gneisses on a F1–F2 plot suggests that intermediate and felsic igneous and quartzose sedimentary rocks were the sources of detritus for their protoliths (A). The trend toward high Zr/Sc ratios (C) and positive Hf and Zr anomalies (D) are interpreted to reflect concentration of zircon during sedimentary processes. Positive Pb and negative P spidergram anomalies characterise average continental crust (D) (McLennan Citation2001), and are indicative of moderate degrees of weathering. Spidergram patterns of the quartzofeldspathic gneisses have these same anomalies (D), which also suggests their protoliths were sediments. This is also suggested by their horizontal trend above the Ab-Or join on the ACNK triangle (B).
The mafic mineralogy of the hornblende gneisses indicates that they may have been mafic lava flows, intrusions, volcaniclastic (e.g. tuffs) and/or volcanogenic sedimentary rocks prior to metamorphism. Their geochemistry is consistent with this. The position on the ACNK and F1–F2 diagrams is consistent with an igneous protolith (A, B). The Th/Sc ratio, which is unaffected by chemical weathering and reflects igneous processes (Roser & Korsch Citation1999), suggests a petrogenesis different to calc-alkaline basalt and andesite (C). The high Sr/Y ratio allows classification as HiSY, possibly indicative of a plagioclase-free garnetiferous source rock for the protolith magmas.
The relatively aluminous and potassic composition () of the micaceous gneiss (muscovite–biotite–garnet–plagioclase) is suggestive of a mudstone protolith. This is consistent with the pronounced negative P anomaly (D) and position near the Ab-Or tie line on the ACNK triangle (B), both of which are indicative of moderate degrees of chemical weathering of sediments. The F1–F2 plot and Th/Sc and Zr/Sc ratios both suggest an intermediate composition igneous source rock for the sedimentary protolith (A,C). The high HREE abundance (D) probably reflects preferential preservation of relatively unreactive and physically resistant minerals such as zircon during weathering, erosion and sedimentary processes.
The youngest zircon population (120.6 ± 0.8 Ma) is probably of metamorphic origin due to their low Th/U ratios and lack of oscillatory zoning (A, D) (Corfu et al. Citation2003; Hoskin & Schaltegger Citation2003). The age of the metamorphic overgrowths overlaps the youngest magmatic age for the Woodham Orthogneiss. Since the Woodham Orthogneiss intruded the Davis Creek Paragneiss, this is interpreted to show that intrusion of the Woodham Orthogneiss protolith facilitated metamorphism of the Davis Creek Paragneiss. The Triassic populations have Th/U ratios similar to magmatic zircon ( > 0.5, D) and are therefore thought to be detrital. The age of the younger Triassic population (227.1 ± 2.5 Ma) is tentatively considered as the maximum depositional age. Although there are also Jurassic zircons in this sample, their origin (e.g. Pb-loss or metamorphic) and statistical significance is uncertain due to the limited number of analyses.
The U–Pb zircon geochronology of sample OU81996 also sheds some light on the affinity of the Davis Creek Paragneiss. Similar age spectra are reported for the George Sound Paragneiss (GSP), which occurs as several large xenoliths in the WFO Worsley Pluton (Bradshaw Citation1990; Hollis et al. Citation2004; Clarke et al. Citation2009), the Parapara Group in northwest Nelson, which is interpreted as remnants of the paleo-Pacific Gondwana cover sequence deposited on the Takaka Terrane (Wysoczanski et al. Citation1997), and a micaceous paragneiss xenolith (Borland paragneiss) from within the OMB in southeast Fiordland (Scott et al. Citation2009b) (). The age spectra of these rocks are distinctive for metasediments in the Western Province and Median Batholith in that they are characterised by the presence of several significant Permian–Jurassic zircon populations and, in some cases, apparently lack pre-Silurian zircons that are typical of Western Province metasedimentary rocks () (Ireland Citation1992; Gibson & Ireland Citation1996; Wysoczanski et al. Citation1997; Ireland & Gibson Citation1998; Hollis et al. Citation2004; Clarke et al. Citation2009). The quartzofeldspathic lithologies that comprise the Davis Creek Paragneiss are also similar to those of the GSP (Bradshaw Citation1990; Clarke et al. Citation2009). On the basis of their similar distinctive Permian–Jurassic detrital zircon populations, the Davis Creek Paragneiss is considered to be a correlative of, or at least to have had the same source rocks as, the GSP, Parapara Group and Borland paragneiss, and may also represent a fragment of the paleo-Pacific Gondwana margin cover sequence. Furthermore, the spatial association with the Worsley Pluton-correlative Woodham Orthogneiss, and the similarity of their constituent lithologies, suggests that the Davis Creek Paragneiss may be the direct correlative of the GSP. The Triassic Topfer Formation, which consists of well-indurated sedimentary rocks and is also considered to be a remnant of the paleo-Pacific Gondwana cover sequence (Mortimer & Smale Citation1996), may also be related to the Davis Creek Paragneiss. Unfortunately, no geochemical or zircon geochronological data are available to test this.
Tectonic implications
Correlation of the Glenroy Complex and McKnee Intrusives with similar rocks in northern Fiordland leaves open the possibility that they were exhumed during at least two discrete stages. The first stage of exhumation may have occurred in the Cretaceous prior to being displaced from their correlatives by movement on the Alpine Fault that has occurred since c. 23 Ma, when the modern plate boundary formed (Kamp Citation1986; Cooper et al. Citation1987). Partial exhumation of the WFO Malaspina Pluton during mid-Cretaceous continental extension was shown by Davids (Citation1999) using 40Ar/39Ar thermochronology. Limited zircon fission-track cooling ages (closure temperature = 240 ± 20 °C) up to c. 45 Ma for the Worsley Pluton (Sutherland et al. Citation2009) also hint at significant exhumation prior to inception of the modern plate boundary. The subsequent exhumation stage(s) may have taken place after Alpine Fault offset of the Nelson and Fiordland regions, during tectonic emplacement of the blocks in the Matakitaki foreland thrust system. Alternatively, the Glenroy Complex may have been entirely exhumed during Miocene–Holocene times as a result of localised shortening and development of the Matakitaki foreland thrust system associated with the Alpine Fault Big Bend. The Glenroy, McKnee, Matakitaki and Station blocks in the Matakitaki foreland thrust system crop out c. 10 km along the strike of the Alpine Fault (). The relative present-day positions of these blocks matches that of their correlatives (Worsley Pluton/GSP, Darran Suite plutons, Dun Mountain–Maitai Terrane and Caples Terrane, respectively) in northern Fiordland and West Otago, which are exposed over c. 100 km alongstrike of the Alpine Fault (). This suggests that the Glenroy area has been tectonically shortened during Alpine Fault movement and/or development of the Big Bend.
Figure 9. Simplified geological maps showing the tectonostratigraphic basement rock units of A, the South Island; B, the Glenroy area, southeast Nelson; and C, northern Fiordland. Fault-bound blocks of many of the basement units in northern Fiordland, including the WFO, GSP and Late Triassic and Early Cretaceous Darran Suite, that are exposed c. 100 km along the strike of the Alpine Fault crop out over only c. 10 km parallel to the Alpine Fault in the Glenroy River valley, southeast Nelson. The circled numbers indicate plutonic/gneissic basement units in northern Fiordland and southeast Nelson that have similar geochemistry and geochronology, and equivalent Eastern Province Terranes.
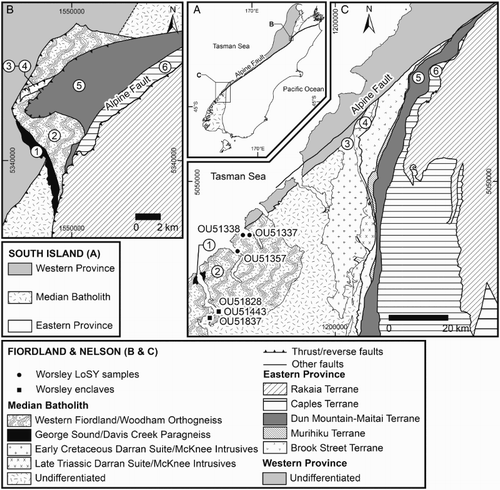
Figure 10. Gaussian-summation probability density distributions of zircon ages in the Davis Creek Paragneiss (OU81996) with those in the George Sound Paragneiss (GSP), Borland paragneiss and Parapara Group (PG). Data sources: Wysoczanski et al. (Citation1997); Clarke et al. (Citation2009); Scott et al. (Citation2009b).
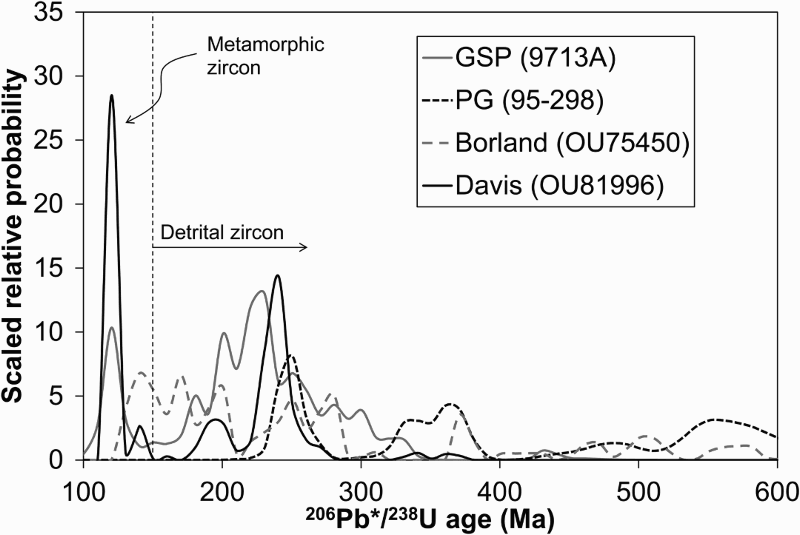
The geographic position and approximate north–south trend of the Mt Cann Pluton suggests that it is the southwards continuation of the Separation Point Batholith. This is consistent with their similar geochemistry and ages. It is therefore concluded that the Mt Cann Pluton is essentially autochthonous basement. Intrusive contacts between Mt Cann Pluton and Takaka Terrane rocks at Fyfe Peak are consistent with this interpretation (Campbell Citation1992).
Conclusions
The crystalline basement rocks of the Glenroy area are formally divided into three main units: Glenroy Complex, McKnee Intrusives and Mt Cann Pluton. Together with rocks of Takaka, Dun Mountain–Maitai and Caples terrane affinity, they form fault-bound blocks in the Matakitaki foreland thrust system that has developed in the Australia Plate adjacent to the Alpine Fault Big Bend. The Glenroy Complex consists of the Davis Creek Paragneiss and Woodham Orthogneiss. The Davis Creek Paragneiss comprises quartzofeldspathic, hornblende, micaceous and probable meta-igneous granitic gneisses, while two-pyroxene–hornblende monzodioritic orthogneisses that have been largely retrogressed to hornblende–biotite mineral assemblages dominate the Woodham Orthogneiss. The mineralogy, geochemistry (e.g. negative P anomaly) and U–Pb zircon age spectrum of a quartzofeldspathic gneiss sample (OU81996) from the Davis Creek Paragneiss are suggestive of sedimentary protoliths and indicate a maximum sedimentation age of c. 227 Ma. U–Pb zircon dating indicates that the Woodham Orthogneiss protoliths were intruded between 126.6 ± 1.3 Ma and 121.8 ± 1.1 Ma, which facilitated metamorphism of the Davis Creek Paragneiss that is dated to 120.6 ± 0.9 Ma. Similarities in age, lithology and geochemical composition indicate that the Woodham Orthogneiss belongs to the SPS, and is specifically correlated with the WFO Worsley Pluton in northern Fiordland. The Davis Creek Paragneiss is also matched with similar rocks in northern Fiordland – the George Sound Paragneiss – due to their similar constituent lithologies, distinctive U–Pb zircon age spectra with significant Permian–Jurassic populations and lack of any pre-Silurian age peaks.
The McKnee Intrusives comprise hornblende–biotite quartz monzodiorite and biotite granodiorite. Emplacement of the McKnee Intrusives occurred in two discrete episodes: Late Triassic (225.0 ± 2.7 Ma) and Early Cretaceous (137.8 ± 0.7 Ma). Together, the lithologies, geochemistry and ages indicate that the McKnee Intrusives are correlatives of Late Triassic (e.g. Mistake Diorite) and Early Cretaceous (e.g. Darran Leucogabbro) Darran Suite plutons, which are also located in northern Fiordland. The Mt Cann Pluton is dominated by porphyritic biotite−epidote quartz monzodiorite that was intruded at 113.8 ± 1.0 Ma. The Mt Cann Pluton is correlated with the Separation Point Granite on the basis of their similar ages and HiSY geochemistry.
Correlation of the Glenroy Complex and McKnee Intrusives with rocks of northern Fiordland means it is possible that they were exhumed during at least two stages. The first stage may have occurred in the Cretaceous, prior to post-Oligocene displacement along the Alpine Fault, and the second stage may be related to subsequent tectonic emplacement in the Matakitaki foreland thrust system. The Glenroy, McKnee, Matakitaki and Station blocks crop out along c. 10 km parallel to the Alpine Fault, while their correlatives in northern Fiordland are exposed over c. 100 km alongstrike of the Alpine Fault. In contrast, correlation of the Mt Cann Pluton with the Separation Point Granite strongly suggests that it is an essentially autochthonous part of the basement in the Glenroy area.
Supplementary data
Summary S1. Analytical methods.
Table S1. LA-ICP-MS U–Th–Pb zircon data for Woodham two-pyroxene−hornblende monzodioritic orthogneiss (OU81949).
Table S2. ID-TIMS U–Pb–Th zircon data for Woodham hornblende−biotite monzodioritic orthogneiss (P51298).
Table S3. LA-ICP-MS U–Th–Pb zircon data for Woodham biotite monzogranitic orthogneiss (OU81937).
Table S4. LA-ICP-MS U–Th–Pb zircon data for Davis Creek quartzofeldspathic gneiss (OU81996).
Table S5. LA-ICP-MS U–Th–Pb zircon data for McKnee Intrusives hornblende−biotite quartz monzodiorite (OU81925).
Table S6. LA-ICP-MS U–Pb–Th zircon data for McKnee Intrusives hornblende−biotite quartz monzodiorite (OU82005).
Table S7. LA-ICP-MS U–Th–Pb zircon data for Mt Cann Pluton biotite–epidote quartz monzodiorite (OU82035).
Tables S1–S7.
Download Zip (4.8 MB)Summary S1. Analytical methods.
Download MS Word (19.5 KB)Acknowledgements
James Scott and Jelte Keeman are thanked for their comments on earlier versions of this manuscript, which greatly improved the final product. Reviews by Mark Rattenbury and Nathan Daczko, and the comments of Associate Editor Richard Jongens, also helped to significantly improve the manuscript. Brent Pooley, Damian Walls and Charlotte Allen helped with sample preparation and analyses. Virginia Toy provided invaluable guidance during field mapping. Dave Kimbrough is thanked for allowing publication of the ID-TIMS U–Pb zircon data.
Associate Editor: Dr Richard Jongens.
Disclosure statement
No potential conflict of interest was reported by the authors.
Additional information
Funding
References
- Adamson RG. 1966. Stratigraphy and structure in the northern part of the area between the Glenroy and Matakitaki Rivers, South Nelson, New Zealand [Unpublished thesis]. Christchurch: University of Canterbury.
- Allibone AH, Jongens R, Scott JM, Tulloch AJ, Turnbull IM, Cooper AF, Powell NG, Ladley EB, King RP, Rattenbury MS. 2009a. Plutonic rocks of the Median Batholith in eastern and central Fiordland, New Zealand: field relations, geochemistry, correlation, and nomenclature. New Zeal J Geol Geophys. 52:101–148.
- Allibone AH, Jongens R, Turnbull IM, Milan IM, Daczko NR, De Paoli MC, Tulloch AJ. 2009b. Plutonic rocks of western Fiordland, New Zealand: field relations, geochemistry, correlation, and nomenclature. New Zeal J Geol Geophys. 52:379–415. doi: 10.1080/00288306.2009.9518465
- Benson WN. 1952. Meeting of the Geological Division of the Pacific Science Congress in New Zealand, 1949. Interim Proceedings of the Geological Society of America 1950, 1; p. 11–13.
- Berryman KR, Beanland S, Cooper AF, Cutten HNC, Norris RJ, Wood PR. 1992. The Alpine Fault, New Zealand: variation in Quaternary structural style and geomorphic expression. Ann Tectonicae. 6:126–163.
- Blattner P. 1978. Geology of the crystalline basement between Milford Sound and the Hollyford Valley, New Zealand. New Zeal J Geol Geophys. 21:33–47. doi: 10.1080/00288306.1978.10420720
- Blattner P. 2006. Chemical and isotopic trends and tectonics of the Milford Gneiss Complex and Darran batholith of northern Fiordland, New Zealand. GNS Science Report 2006/36. Lower Hutt: GNS Science.
- Blattner P, Graham IJ. 2000. New Zealand's Darran Complex and McKay Intrusives—Rb-Sr whole-rock isochrons in the Median Tectonic Zone. Am J Sci. 300:603–629. doi: 10.2475/ajs.300.8.603
- Bolhar R, Weaver SD, Palin JM, Cole JW, Paterson LA. 2008. Systematics of zircon crystallisation in the cretaceous separation point suite, New Zealand, using U/Pb isotopes, REE and Ti geothermometry. Contrib Mineral Petrol. 156:133–160. doi: 10.1007/s00410-007-0278-5
- Bradshaw JD. 1989. Cretaceous geotectonic patterns in the New Zealand region. Tectonics. 8:803–820. doi: 10.1029/TC008i004p00803
- Bradshaw JY. 1989. Origin and metamorphic history of an early cretaceous polybaric granulite terrain, Fiordland, southwest New Zealand. Contrib Mineral Petrol. 103:346–360. doi: 10.1007/BF00402921
- Bradshaw JY. 1990. Geology of crystalline rocks of northern Fiordland: details of the granulite facies Western Fiordland Orthogneiss and associated rock units. New Zeal J Geol Geophys. 33:465–484. doi: 10.1080/00288306.1990.10425702
- Bradshaw JY, Kimbrough DL. 1991. Mid-Paleozoic age of granitoids in enclaves within early cretaceous granulites, Fiordland, southwest New Zealand. New Zeal J Geol Geophys. 34:455–469. doi: 10.1080/00288306.1991.9514483
- Burgess AJ. 2004. Emplacement mechanism and geochemical zoning of the separation point Batholith, Northwest Nelson, New Zealand [Unpublished thesis]. Christchurch: University of Canterbury.
- Campbell JK. 1992. The Wairau and Alpine Faults—structure and tectonics of the S-bend junction. In: Campbell J, editor. Field trip guides: Joint Annual Conference of the Geological Society of New Zealand and New Zealand Geophysical Society. Geological Society of New Zealand Miscellaneous Publication 63B. Christchurch: University of Canterbury; p. 10–30.
- Campbell JK, Cutten HNC. 1985. Foreland structure west of the Alpine Fault in the region of the Glenroy valley, south Nelson. Christchurch Conference Programme and Abstacts booklet. Miscellaneous Publication 32A. Takaka: Geological Society of New Zealand.
- Challis GA, Johnston MR, Lauder WR, Suggate RP. 1994. Geology of the Lake Rotoroa area, Nelson Sheet M29BD. Scale 1:50,000. Institute of Geological and Nuclear Sciences geological map 8. Lower Hutt: Institute of Geological & Nuclear Sciences.
- Clarke GL, Fitzherbert JA, Milan IM, Daczko NR, Degeling HS. 2009. Anti-clockwise P-T paths in the lower crust: an example from a kyanite-bearing regional aureole, George Sound, New Zealand. J Metamorph Geol. 28:77–96. doi: 10.1111/j.1525-1314.2009.00854.x
- Cooper AF, Barreiro BA, Kimbrough DL, Mattinson JM. 1987. Lamprophyre dike intrusion and the age of the Alpine fault, New Zealand. Geology. 15:941–944. doi: 10.1130/0091-7613(1987)15<941:LDIATA>2.0.CO;2
- Corfu F, Hanchar JM, Hoskin PWO, Kinny P. 2003. Atlas of zircon textures. Rev Miner Geochem. 53:469–500. doi: 10.2113/0530469
- Cutten HNC. 1979. Rappahannock Group: Late Cenozoic sedimentation and tectonics contemporaneous with Alpine Fault movement. New Zeal J Geol Geophys. 22:535–553. doi: 10.1080/00288306.1979.10424165
- Cutten HNC. 1987. Geology of the bends area of the Alpine Fault. In: Beanland S, editor. Field guide to sites of active earth deformation, South Island, New Zealand. Lower Hutt: New Zealand Geological Survey, DSIR; p. 36–39.
- Daczko NR, Emami S, Allibone AH, Turnbull IM. 2012. Petrogenesis and geochemical characterisation of ultramafic cumulate rocks from Hawes Head, Fiordland, New Zealand. New Zeal J Geol Geophys. 55:361–374
- Davids C. 1999. A thermochronological study of southern Fiordland, New Zealand [Unpublished thesis]. Canberra, ACT: Australian National University.
- Defant MJ, Drummond MS. 1990. Derivation of some modern arc magmas by melting of young subducted lithosphere. Nature. 347:662–665. doi: 10.1038/347662a0
- Dodson MH, Compston W, Williams IS, Wilson JF. 1988. A search for ancient detrital zircons in Zimbabwean sediments. J Geol Soc. 145:977–983. doi: 10.1144/gsjgs.145.6.0977
- Fedo CM, Nesbitt HW, Young GM. 1995. Unraveling the effects of potassium metasomatism in sedimentary rocks and paleosols, with implications for paleoweathering conditions and provenance. Geology. 23:921–924. doi: 10.1130/0091-7613(1995)023<0921:UTEOPM>2.3.CO;2
- Fyfe HE. 1968. Geology of the Murchison subdivision. New Zeal Geol Surv Bull. 36:51.
- Gibson GM, Ireland TR. 1996. Extension of Delamerian (Ross) orogen into western New Zealand: evidence from zircon ages and implications for crustal growth along the Pacific margin of Gondwana. Geology. 24:1087–1090. doi: 10.1130/0091-7613(1996)024<1087:EODROI>2.3.CO;2
- Gibson GM, McDougall I, Ireland TR. 1988. Age constraints on metamorphism and the development of a metamorphic core complex in Fiordland, southern New Zealand. Geology. 16:405–408. doi: 10.1130/0091-7613(1988)016<0405:ACOMAT>2.3.CO;2
- Graham IJ, White PJ. 1990. Rb-Sr dating of Rahu Suite granitoids from the Paparoa Range, North Westland, New Zealand. New Zeal J Geol Geophys. 33:11–22. doi: 10.1080/00288306.1990.10427568
- Grew E. 1977. First report of kyanite-sillimanite association in New Zealand. New Zeal J Geol Geophys. 20:797–802. doi: 10.1080/00288306.1977.10430733
- Hattori H. 1967. Occurence of sillimanite-garnet-biotite gneisses and their significance in metamorphic zoning in the South Island, New Zealand. New Zeal J Geol Geophys. 10:269–299. doi: 10.1080/00288306.1967.10428197
- Hiess J, Ireland TR, Rattenbury MS. 2010. U-Th-Pb zircon and monazite geochronology of Western Province gneissic rocks, central-south Westland, New Zealand. New Zeal J Geol Geophys. 53:241–269. doi: 10.1080/00288306.2010.495450
- Hollis JA, Clarke GL, Klepeis KA, Daczko NR, Ireland TR. 2003. Geochronology and geochemistry of high-pressure granulites of the Arthur River Complex, Fiordland, New Zealand: Cretaceous magmatism and metamorphism of the palaeo-Pacific Margin. J Metamorph Geol. 21:299–313. doi: 10.1046/j.1525-1314.2003.00443.x
- Hollis JA, Clarke GL, Klepeis KA, Daczko NR, Ireland TR. 2004. The regional significance of cretaceous magmatism and metamorphism in Fiordland, New Zealand, from U-Pb geochronology. J Metamorph Geol. 22:607–627. doi: 10.1111/j.1525-1314.2004.00537.x
- Hoskin PWO, Schaltegger U. 2003. The composition of zircon and igneous and metamorphic petrogenesis. Rev Miner Geochem. 53:27–62. doi: 10.2113/0530027
- Ireland TR. 1992. Crustal evolution of New Zealand: evidence from age distributions of detrital zircons in Western Province paragneisses and Torlesse greywacke. Geochemica et Cosmochimica Acta. 56:911–920. doi: 10.1016/0016-7037(92)90036-I
- Ireland TR, Gibson GM. 1998. SHRIMP monazite and zircon geochronology of high-grade metamorphism in New Zealand. J Metamorph Geol. 16:149–167. doi: 10.1111/j.1525-1314.1998.00112.x
- Johnston MR. 1990. Geology of the St Arnaud District, Southeast Nelson (Sheet N29). New Zealand Geological Survey Bulletin 99. Lower Hutt: New Zealand Geological Survey.
- Kamp PJJ. 1986. The mid-cenozoic challenger rift system of western New Zealand and its implications for the age of Alpine fault inception. Geol Soc Am Bull. 97:255–281. doi: 10.1130/0016-7606(1986)97<255:TMCRSO>2.0.CO;2
- Kimbrough DL, Tulloch AJ, Geary E, Coombs DS, Landis CA. 1993. Isotopic ages from the Nelson region of South Island New Zealand: crustal structure and definition of the Median Tectonic Zone. Tectonophysics. 225:433–448. doi: 10.1016/0040-1951(93)90308-7
- Kimbrough DL, Tulloch AJ, Coombs DS, Landis CA, Johnston MR, Mattinson JM. 1994. Uranium-lead zircon ages from the median tectonic zone, New Zealand. New Zeal J Geol Geophys. 37:393–419. doi: 10.1080/00288306.1994.9514630
- Klepeis KA, Clarke GL, Gehrels G, Vervoort J. 2004. Processes controlling vertical coupling and decoupling between the upper and lower crust of orogens: results from Fiordland, New Zealand. J Struct Geol. 26:765–791. doi: 10.1016/j.jsg.2003.08.012
- Mann P. 2007. Global catalogue, classification and tectonic origins of restraining- and releasing bends on active and ancient strike-slip fault systems. Geol Soc London Spec Publ. 290:13–142. doi: 10.1144/SP290.2
- Marcotte SB, Klepeis KA, Clarke GL, Gehrels G, Hollis JA. 2005. Intra-arc transpression in the lower crust and its relationship to magmatism in a Mesozoic magmatic arc. Tectonophysics. 407:135–163. doi: 10.1016/j.tecto.2005.07.007
- Mattinson JM, Kimbrough DL, Bradshaw JY. 1986. Western Fiordland orthogneiss: early cretaceous arc magmatism and granulite facies metamorphism, New Zealand. Contrib Mineral Petrol. 92:383–392. doi: 10.1007/BF00572167
- McCoy-West AJ, Mortimer N, Ireland TR. 2014. U–Pb geochronology of Permian plutonic rocks, Longwood range, New Zealand: implications for Median Batholith–Brook Street Terrane relations. New Zeal J Geol Geophys. 57:65–85. doi: 10.1080/00288306.2013.869235
- McCulloch MT, Bradshaw JY, Taylor SR. 1987. Sm-Nd and Rb-Sr isotopic and geochemical systematics in Phanerozoic granulites from Fiordland, Southwest, New Zealand. Contrib Mineral Petrol. 97:183–195. doi: 10.1007/BF00371238
- McLennan SM. 2001. Relationships between the trace element composition of sedimentary rocks and upper continental crust. Geochem Geophys Geosy. 2: 2000GC000109. doi: 10.1029/2000GC000109
- Mortimer N, Gans P, Calvert A, Walker NW. 1999a. Geology and thermochronometry of the east edge of the Median Batholith (Median Tectonic Zone): a new perspective on Permian to Cretaceous crustal growth of New Zealand. Island Arc. 8:404–425. doi: 10.1046/j.1440-1738.1999.00249.x
- Mortimer N, Rattenbury MS, King PR, Bland KJ, Barrell DJA, Bache F, Begg JG, Campbell HJ, Cox SC, Crampton JS and others. 2014. High-level stratigraphic scheme for New Zealand rocks. New Zeal J Geol Geophys. 57:402–419. doi: 10.1080/00288306.2014.946062
- Mortimer N, Smale D. 1996. Petrology of the Topfer Formation: First Triassic Gondwana sequence from New Zealand. Aust J Earth Sci. 43:467–477. doi: 10.1080/08120099608728268
- Mortimer N, Tulloch AJ, Spark RN, Walker NW, Ladley EB, Allibone AH, Kimbrough DL. 1999b. Overview of the Median Batholith, New Zealand: a new interpretation of the geology of the Median Tectonic Zone and adjacent rocks. J Afr Earth Sci. 29:257–268. doi: 10.1016/S0899-5362(99)00095-0
- Muir RJ, Ireland TR, Weaver SD, Bradshaw JD. 1994. Ion microprobe U-Pb zircon geochronology of granitic magmatism in the western province of the South Island, New Zealand. Chem Geol. 113:171–189. doi: 10.1016/0009-2541(94)90011-6
- Muir RJ, Ireland TR, Weaver SD, Bradshaw JD, Evans JA, Eby GN, Shelley D. 1998. Geochronology and geochemistry of a Mesozoic magmatic arc system, Fiordland, New Zealand. J Geol Soc London. 155:1037–1053. doi: 10.1144/gsjgs.155.6.1037
- Muir RJ, Ireland TR, Weaver SD, Bradshaw JD, Waight TE, Jongens R, Eby GN. 1997. SHRIMP U-Pb geochronology of Cretaceous magmatism in northwest Nelson–Westland, South Island, New Zealand. New Zeal Geol Geop. 40:453–463. doi: 10.1080/00288306.1997.9514775
- Muir RJ, Weaver SD, Bradshaw JD, Eby GN, Evans JA. 1995. The cretaceous separation point Batholith, New Zealand: granitoid magmas formed by melting of mafic lithosphere. J Geol Soc London. 152:689–701. doi: 10.1144/gsjgs.152.4.0689
- Nathan S, Rattenbury MS, Suggate RP. 2002. Geology of the Greymouth area. Institute of Geological & Nuclear Sciences 1:250,000 geological map 12. Lower Hutt: New Zealand, Institute of Geological & Nuclear Sciences Limited.
- Oliver GJH. 1977. Feldspathic hornblende and garnet granulites and associated anorthosite pegmatites from Doubtful Sound, Fiordland, New Zealand. Contrib Mineral Petrol. 65:111–121. doi: 10.1007/BF00371051
- Oliver GJH. 1980. Geology of the granulite and amphibolite facies gneisses of Doubtful Sound, Fiordland, New Zealand. New Zeal Geol Geop. 23:27–41. doi: 10.1080/00288306.1980.10424190
- Price RC, Spandler C, Arculus RJ, Reay AJ. 2011. The Longwood Igneous Complex, Southland, New Zealand: a Permo-Jurassic, intra-oceanic, subduction-related, I-type batholithic complex. Lithos. 126:1–21. doi: 10.1016/j.lithos.2011.04.006
- Rattenbury MS, Townsend DB, Johnston MR. 2006. Geology of the Kaikoura area. Institute of Geological & Nuclear Sciences geological map 13. Lower Hutt: New Zealand, GNS Science.
- Reay A, Sipiera P. 1987. Mantle xenoliths from the New Zealand region. In: Nixon P, editor. Mantle xenoliths. Chichester, UK: John Wiley & Sons Ltd.; p. 347–358.
- Reid D, Bailey D, French D. 1972. Orbicular texture in boulders from the Glenroy Valley (S39) south-east Nelson. New Zeal J Geol Geophys. 15:643–648. doi: 10.1080/00288306.1972.10423989
- Roser BP, Korsch RJ. 1988. Provenance signatures of sandstone-mudstone suites determined using discriminant function analysis of major-element data. Chem Geol. 67:119–139. doi: 10.1016/0009-2541(88)90010-1
- Roser BP, Korsch RJ. 1999. Geochemical characterisation, evolution and source of a Mesozoic accretionary wedge: the Torlesse Terrane, New Zealand. Geol Mag. 136:493–512. doi: 10.1017/S0016756899003003
- Sagar MW, Palin JM. 2011. Emplacement, metamorphism, deformation and affiliation of mid-Cretaceous orthogneiss from the Paparoa Metamorphic Core Complex lower-plate, Charleston, New Zealand. New Zeal J Geol Geophys. 54:273–289. doi: 10.1080/00288306.2011.562904
- Sambridge MS, Compston W. 1994. Mixture modeling of multi-component data sets with application to ion-probe zircon ages. Earth Planet Sci Lett. 128:373–390. doi: 10.1016/0012-821X(94)90157-0
- Scott JM. 2013. A review of the location and significance of the boundary between the Western Province and Eastern Province, New Zealand. New Zeal J Geol Geophys. 56:276–293. doi: 10.1080/00288306.2013.812971
- Scott JM, Cooper AF, Palin JM. 2009a. Beehive Diorite: a late Jurassic two-pyroxene pluton at Lake Manapouri, Fiordland. New Zeal J Geol Geophys. 52:59–65. doi: 10.1080/00288300909509878
- Scott JM, Cooper AF, Palin JM, Tulloch AJ, Kula J, Jongens R, Spell TL, Pearson NJ. 2009b. Tracking the influence of a continental margin on growth of a magmatic arc, Fiordland, New Zealand, using thermobarometry, thermochronology, and zircon U-Pb and Hf isotopes. Tectonics. 28:TC6007. doi: 10.1029/2009TC002489
- Scott JM, Cooper AF, Tulloch AJ, Spell TL. 2011a. Crustal thickening of the early cretaceous paleo-pacific Gondwana margin. Gondwana Res. 20:380–394. doi: 10.1016/j.gr.2010.10.008
- Scott JM, Muhling J, Fletcher I, Billia M, Palin JM, Elliot T, Günter C. 2011b. The relationship of Palaeozoic metamorphism and S-type magmatism on the paleo-Pacific Gondwana margin. Lithos. 127:522–534. doi: 10.1016/j.lithos.2011.09.008
- Scott JM, Palin JM. 2008. LA-ICP-MS U-Pb zircon ages from Mesozoic plutonic rocks in eastern Fiordland, New Zealand. New Zeal J Geol Geophys. 51:105–113. doi: 10.1080/00288300809509853
- Stowell H, Parker KO, Gatewood M, Tulloch AJ, Koenig A. 2014. Temporal links between pluton emplacement, garnet granulite metamorphism, partial melting and extensional collapse in the lower crust of a Cretaceous magmatic arc, Fiordland, New Zealand. J Metamorph Geol. 32:151–175. doi: 10.1111/jmg.12064
- Sun S-s, McDonough WF. 1989. Chemical and isotopic systematics of oceanic basalts: implications for mantle composition and processes. In: Saunders AD, Norry MJ, editors. Magmatism in the ocean basins. London: Geological Society Special Publication 42; p. 313–345.
- Sutherland R, Gurnis M, Kamp PJJ, House MA. 2009. Regional exhumation history of brittle crust during subduction initiation, Fiordland, southwest New Zealand, and implications for thermochronologic sampling and analysis strategies. Geosphere. 5:409–425. doi: 10.1130/GES00225.SA2
- Tulloch AJ. 1981. The glenroy metamorphic complex. New Zealand Geological Survey Conference. Turangi, New Zealand Geological Survey; p. 29.
- Tulloch AJ. 1983. Granitoid rocks of New Zealand—a brief review. Geol Soc Am Mem. 159:5–20. doi: 10.1130/MEM159-p5
- Tulloch AJ. 1988. Batholiths, plutons, and suites: nomenclature for granitoid rocks of Westland-Nelson, New Zealand. New Zeal J Geol Geophys. 31:505–509. doi: 10.1080/00288306.1988.10422147
- Tulloch AJ, Brathwaite RL. 1986. Granitoid rocks and associated mineralisation of Westland–Nelson, New Zealand. In: Houghton B, Weaver S, editor. South Island igneous rocks: tour Guides A3, C2 and C7. Lower Hutt: Department of Scientific and Industrial Research; p. 65–92.
- Tulloch AJ, Campbell JK. 1993. Clinoenstatite-bearing buchites possibly from combustion of hydrocarbon gases in a major thrust zone: Glenroy Valley, New Zealand. J Geol. 101:404–412. doi: 10.1086/648233
- Tulloch AJ, Challis GA. 2000. Emplacement depths of Paleozoic–Mesozoic plutons from western New Zealand estimated by hornblende-Al geobarometry. New Zeal J Geol Geophys. 43:555–567. doi: 10.1080/00288306.2000.9514908
- Tulloch AJ, Ireland TR, Kimbrough DL, Griffin WL, Ramezani J. 2011. Autochthonous inheritence of zircon through cretaceous partial melting of carboniferous plutons: the Arthur River Complex, Fiordland, New Zealand. Contrib Mineral Petrol. 161:401–421. doi: 10.1007/s00410-010-0539-6
- Tulloch AJ, Kimbrough DL. 2003. Paired plutonic belts in convergent margins and the development of high Sr/Y magmatism: peninsular ranges batholith of Baja-California and median batholith of New Zealand. In: Johnson S, Patterson S, Fletcher J, Girty G, Kimbrough D, Martín-Barajas A, editors. Tectonic evolution of northwestern Mexico and the southwestern USA. Boulder, CO: Geological Society of America; p. 275–295.
- Tulloch AJ, Rabone SDC. 1993. Mo-bearing granodiorite porphyry plutons of the early cretaceous separation point suite. New Zeal J Geol Geophys. 36:401–408. doi: 10.1080/00288306.1993.9514586
- Tulloch AJ, Ramezani J, Kimbrough DL, Faure K, Allibone AH. 2009. U-Pb geochronology of mid-Paleozoic plutonism in western New Zealand: Implications for S-type granite generation and growth of the east Gondwana margin. Geol Soc Am Bull. 121:1236–1261. doi: 10.1130/B26272.1
- Turnbull IM. 2000. Geology of the Wakatipu area. Institute of Geological & Nuclear Sciences. 1:250, 000 geological map 18. Lower Hutt: Institute of Geological & Nuclear Sciences.
- Turnbull IM, Allibone AH, Jongens R. 2010a. Geology of the Fiordland area. Institute of Geological & Nuclear Sciences geological map 17. Lower Hutt: GNS Science.
- Turnbull R, Weaver SD, Tulloch AJ, Cole J, Handler M, Ireland TR. 2010b. Field and geochemical constraints on mafic-felsic interactions, and processes in high-level arc magma chambers: an example from the Halfmoon Pluton, New Zealand. J Petrol. 51:1477–1505. doi: 10.1093/petrology/egq026
- Waight TE, Weaver SD, Ireland TR, Mass R, Muir RJ, Shelley D. 1997. Field characteristics, petrography, and geochronology of the Hohonu Batholith and the adjacent Granite Hill Complex, North Westland, New Zealand. New Zeal J Geol Geophys. 40:1–17. doi: 10.1080/00288306.1997.9514736
- Waight TE, Weaver SD, Muir JM, Mass R, Eby GN. 1998. The Hohonu Batholith of North Westland, New Zealand: granitoid compositions controlled by source H2O contents and generated during tectonic transition. Contrib Mineral Petrol. 130:225–239. doi: 10.1007/s004100050362
- Whitney DL, Evans BW. 2010. Abbreviations for names of rock-forming minerals. Am Mineral. 95(1):185–187. doi: 10.2138/am.2010.3371
- Williams J, Harper C. 1978. Age and status of the Mackay Intrusives in the Eglinton-upper Hollyford area. New Zeal J Geol Geophys. 21:733–742. doi: 10.1080/00288306.1978.10425202
- Wysoczanski RJ, Gibson GM, Ireland TR. 1997. Detrital zircon age patterns and provenance in late Paleozoic-early Mesozoic New Zealand Terranes and development of the paleo-Pacific Gondwana margin. Geology. 25:939–942. doi: 10.1130/0091-7613(1997)025<0939:DZAPAP>2.3.CO;2
- Yeats RS, Berryman KR. 1987. South Island, New Zealand, and transverse ranges, California: a seismotectonic comparison. Tectonics. 6:363–376. doi: 10.1029/TC006i003p00363
Appendix
Stratigraphic nomenclature and geology
The stratigraphic units of metamorphic and granitic rocks in the Glenroy area are ill-defined at the formation level. Many different names have been used for the same groupings of rocks, often without being clearly defined or even described. Formal definitions for the gneissic and granitic rocks in the Glenroy area are proposed in this section. provides a summary of the dominant lithologies and type localities/sections.
Glenroy Complex
Adamson (Citation1966) mapped the gneissic rocks in the Glenroy area as the Woodham Granite, which was considered a formation of the Rotoroa Complex (Fyfe Citation1968). Tulloch (Citation1981) first proposed that the high-grade gneisses of the Glenroy area should be considered as a separate unit to the layered mafic–intermediate intrusives of the Rotoroa Complex; consequently he proposed the name Glenroy Metamorphic Complex as a group-level replacement for Woodham Granite. Glenroy gneiss (Cutten Citation1987) and Glenroy Metamorphics (Campbell Citation1992) have also been used as synonyms for the Glenroy Metamorphic Complex. Rattenbury et al. (Citation2006) shortened Glenroy Metamorphic Complex to Glenroy Complex.
The Glenroy Complex includes all mid-Cretaceous high-grade gneissic rocks that crop out over c. 100 km2 between the lower Glenroy River and Nardoo range and in the low forested hills north of the middle Matakitaki River () (Rattenbury et al. Citation2006). Two formations make up the Glenroy Complex (Tulloch Citation1981; Cutten 1987; Campbell Citation1992; Tulloch & Campbell Citation1993), but neither has been formally defined. These two formations are here named Davis Creek Paragneiss and Woodham Orthogneiss. These names were chosen to avoid confusion that would be caused by introducing new geographic formation prefixes. Davis Creek Paragneiss is a replacement of ‘Davis Creek Granulites’ and ‘Davis Creek Formation’ used by previous authors. Woodham Orthogneiss supersedes ‘Woodham Gneiss’, ‘Woodham monzodiorite’ and ‘Woodham Formation’.
Marble, schist, quartzite and amphibolite intruded by the Mt Cann Pluton at Fyfe Peak (E1545671/N5328668) were included in the Glenroy Complex as Mt Cann Formation by Tulloch (Citation1981), and designated as Fyfe Complex and Fyfe Metamorphics by Campbell & Cutten (Citation1985) and Campbell (Citation1992), respectively. These rocks are probably metamorphosed equivalents of the Late Cambrian–Ordovician Sluice Box Limestone and/or Alfred Formation (both part of the Mt Arthur Group, Takaka Terrane) (Rattenbury et al. Citation2006), and are therefore excluded from the Glenroy Complex.
Davis Creek Paragneiss
The Davis Creek Paragneiss groups together a wide variety of lithologies that are intercalated at the centimetre- to metre-scale and form the western margin of the Glenroy Complex (). Type sections are nominated as lower Davis Creek and a small tributary of Pedro Creek, because they provide exposures of typical quartzofeldspathic gneiss and a diverse range of lithologies, respectively (). The dominant lithology is inequigranular medium- to coarse-grained quartzofeldspathic gneiss. The quartzofeldspathic gneisses are dominated by K-feldspar and quartz with variable amounts of biotite, garnet, plagioclase and epidote. Sillimanite and kyanite have previously been reported in a number of samples from the Davis Creek section (Hattori Citation1967; Grew Citation1977), but upon re-examination of the same samples sillimanite and kyanite were found only in P11473. Disequilibrium textures are common. For example, retrogressive biotite has formed at the expense of garnet, and biotite has been partially replaced by muscovite. Garnetiferous leucosomes in some quartzofeldspathic gneisses in the Pedro Creek and Double Creek catchments indicate partial melting occurred during metamorphism.
The other rocks can be grouped into three lithological types: micaceous gneiss (biotite–muscovite–garnet–plagioclase–quartz), hornblende gneiss (hornblende–biotite–plagioclase–garnet) and suspected meta-igneous biotite and biotite–hornblende granitic gneiss. Hornblende gneisses typically occur as lenses or pods that are c. 0.1 m to > 1 m in length within quartzofeldspathic gneiss and are characteristically fine-grained compared to their host. The only distinctly micaceous gneiss was observed in the Pedro Creek tributary type section, although several micaceous layers intercalated with quartzofeldspathic gneisses in Davis Creek were reported by Hattori (Citation1967). Plagioclase porphyroblasts characterise the granitic gneisses.
Woodham Orthogneiss
The Woodham Orthogneiss dominates the Glenroy Complex and includes interlayered ultramafic–granitic orthogneisses in the Glenroy area east of the Davis Creek Paragneiss, including hornblende gabbro and hornblendite in Cascade Creek that were mapped by Reid et al. (Citation1972) as ‘Cascade ultrabasics’. The dominant lithology making up the Woodham Orthogneiss is inequigranular medium-grained hornblende–biotite monzodioritic orthogneiss. Subordinate lithologies include hornblende–biotite gabbroic, plagioclase-bearing hornblendite and biotite–muscovite–hornblende granodioritic–monzogranitic orthogneisses. The granitic orthogneisses are distinguished from those in the Davis Creek Paragneiss on the presence of conspicuous K-feldspar porphyroblasts that are absent from the latter. Some of the monzogranitic orthogneisses in the Double Creek catchment contain garnetiferous leucosomes. Double Creek (E1546107/N5343658) is proposed as the type section as it is relatively accessible and contains a semi-continuous profile through the Woodham Orthogneiss with many different rock types represented.
All the different rock types occur as distinct, generally concordant sill-like intrusions, which range from a few centimetres to tens of metres thick. Boundaries between intrusions range from sharp to gradational over a few decimetres. It is common in the Woodham Orthogneiss for adjacent intrusions to comprise different mineral assemblages; one layer may comprise a two-pyroxene–hornblende assemblage, while an adjacent layer may either lack clinopyroxene and orthopyroxene entirely or contain only a few relict grains that have been mostly replaced by hornblende and quartz (e.g. OU81949, OU81950). In a few localities (e.g. Cascade Creek, E1548071/N5340999) contact relations between rock types are suggestive of mingling of ultramafic–mafic and intermediate composition magmas. This indicates that the hornblende gabbro and plagioclase hornblendite were molten at the same time as the dioritic lithologies, and suggests that the intermediate composition rocks could have been derived from the ultramafic–mafic lithologies by fractional crystallisation and/or magma mixing.
McKnee Intrusives
The McKnee Intrusives include all massive, unmetamorphosed monzodioritic–granodioritic rocks in the lower East Branch Glenroy River McKnee Intrusives () (new name, after Cutten Citation1987; Campbell Citation1992; Tulloch & Campbell Citation1993). The outcrop exposed in the bed of the lower Glenroy River East Branch (E1546244/N5346603) is nominated as the type locality. The McKnee Intrusives are separated from the Dun Mountain–Maitai Terrane and Matiri Formation (which together form the Matakitaki block) to the southeast by a fault zone (Campbell Citation1992). The northwestern contact with the Neogene Rappahannock Group is marked by a discrete steeply southeast-dipping fault. Intrusion of the McKnee Intrusives occurred in at least two discrete episodes. ‘Intrusives’ is therefore preferred over ‘Pluton’ or a rock type formation suffix (e.g. ‘Granite’) terminology because rocks of widely differing age and composition are included in this unit (following Allibone et al. Citation2009a). McKnee Intrusives replaces ‘McKnee Granite’ and ‘McKnee granodiorite’ used by Cutten (Citation1987) and Campbell (Citation1992), and Tulloch and Campbell (Citation1993), respectively.
Mt Cann Pluton
The Mt Cann Pluton includes all massive–weakly foliated, medium- to coarse-grained, granitic (sensu lato) rocks that occur in the middle–upper Glenroy River valley south of Cascade Creek (). Previously these rocks have been mapped as undifferentiated hornblende−biotite granite and granodiorite belonging to the Separation Point Suite (Rattenbury et al. Citation2006). The high abundance of magmatic epidote is characteristic of the various rock types making up the Mt Cann Pluton. Field evidence for magma mingling suggests that the range of rock types (hornblende gabbro to biotite granite) that make up the Mt Cann Pluton are cogenetic, hence the use of ‘pluton’ as the formation suffix (following Allibone et al. Citation2009a). The summit of Mt Cann is nominated as the type locality because it consists of the dominant porphyritic biotite–epidote quartz monzodiorite.