ABSTRACT
This study presents new radiometric ages from volcanic ash beds within a c. 1900 m thick, progradational, deep-water clastic slope succession of late Miocene age exposed along the north Taranaki coast of the North Island, New Zealand. The ash beds yield U–Pb zircon ages ranging from 10.63 ± 0.65 Ma to 8.97 ± 0.22 Ma. The new ages are compatible with and provide corroboration of New Zealand Tongaporutuan Stage planktic foraminiferal and bolboformid biostratigraphic events identified in the same section. The close accord between these two age datasets provides a stratigraphically consistent and coherent basis for examining margin evolution. The arrival of a prograding clastic wedge and ensuing upward shoaling is recorded by sedimentation rates c. 2000 m/Ma–1 that are an order of magnitude higher than sedimentation rates on the precursor deep basin floor. This outcrop study provides new constraints for interpreting analogous subsurface deposits in Taranaki Basin and complements the regional late Miocene biostratigraphic dating framework.
Introduction
Achieving higher-resolution dating of strata and a more refined understanding of depositional history in the Taranaki Basin are particularly important because of the region's economic significance as the only petroleum-producing area in New Zealand (; King & Thrasher Citation1996). Foraminiferal biostratigraphy has been used extensively for dating and correlation of ancient marine sediments in Taranaki petroleum exploration wells (Hayward Citation1990; King & Thrasher Citation1996; Cooper et al. Citation2001; Scott et al. Citation2004; Hansen & Kamp Citation2006). Most of the basin's sediments are buried, but an extensive exposure of an upper Miocene deep-water succession occurs along the north Taranaki coast. This succession provides a unique window for studying reservoir stratigraphic architecture as analogues for subsurface petroleum-producing intervals nearby (e.g. Ettema et al. Citation2000). The succession also includes the type section for the Tongaporutu Formation (Fleming Citation1959), a precursor constituent of the now-named Mount Messenger Formation which is also the name-bearer reference section for the New Zealand late Miocene Tongaporutuan Stage (Finlay & Marwick Citation1940). This is therefore an extremely important reference section for regional biostratigraphic correlation. Paradoxically, although the strata exposed along the north Taranaki coastal cliff section have been studied for decades, their correlation and dating have been problematic. This is partly because of stratigraphic uncertainty created by the presence of syn-depositional mass-transport deposits (MTDs) and post-depositional normal faults with unknown offsets in various parts of the section (King et al. Citation1993, Citation2011; Hansen Citation1996). Additionally, various workers have used different criteria to determine biostratigraphic events (e.g. Finlay & Marwick Citation1940; Gibson Citation1967; King et al. Citation1993; Scott Citation1995; Crundwell et al. Citation2004). Previous attempts to radiometrically date volcanic ash beds in the Taranaki outcrop succession have been largely unsuccessful due to limitations in previously available methodologies and widespread diagenetic alteration (e.g. Bergman et al. Citation1990; Hansen Citation1996).
Figure 1. Location of study area and outcrops in north Taranaki, North Island, New Zealand. Samples were collected from Taranaki coastal cliffs and two inland exposures (dots). Outcrop location names are referred to in , and the text. Inset shows location of enlarged map within New Zealand. Contemporary late Miocene volcanism (shaded grey) from King & Thrasher (Citation1996) and Adams et al. (Citation1994).
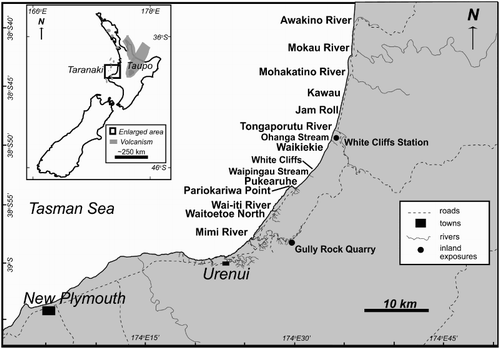
Table 1. Volcanic ash samples and zircon U–Pb ages.
Advances in biostratigraphic dating using new paleomagnetic age-calibrated planktic foraminiferal and bolboformid bioevents from local oceanic sites, ODP Site 1123 (Chatham Rise) and DSDP Site 593 (Challenger Plateau), have since greatly improved chronostratigraphic correlations of upper Miocene rocks in the greater New Zealand region (Crundwell & Nelson Citation2007; Crundwell Citation2014). In addition, recent advances in radiometric dating methods and instruments, such as the sensitive high-resolution ion microprobe-reverse geometry (SHRIMP-RG) and multicollector Noblesse instruments, allow high precision U–Pb and 40Ar/39Ar ages to be measured from individual zircon and feldspar grains, respectively (e.g. Bowring & Schmitz Citation2003; Coble et al. Citation2011; Schaltegger et al. Citation2015).
This study utilises these recent advances in biostratigraphic and radiometric dating to develop a stratigraphically coherent integrated age model for the composite cliff section along the north Taranaki coast, including the reference section for the Tongaporutuan Stage. More precise determination of U–Pb ages has been achieved from carefully sampled, small populations of zircon grains extracted from volcanic ash beds. These new radiometric ages have been compared (in stratigraphic position) with planktic foraminiferal and bolboformid bioevents identified in the section that have their own independent age calibrations.
Background
Regional geology
The Taranaki Basin is located on the western side of North Island, New Zealand, and is a largely offshore basin that covers an area of c. 330,000 km2 (King & Thrasher Citation1992, Citation1996) (). The upper Miocene formations that are the subject of this study are largely confined to the subsurface of the Taranaki Basin, except along the eastern margin of the basin where post-Miocene uplift exposed a thick progradational succession of deep-water basin-floor to shallow-water shelf deposits in coastal cliffs along the north Taranaki coast (King et al. Citation1993, Citation2007a, Citation2007b; Townsend et al. Citation2008) (–).
From oldest (in the north) to youngest (in the south), and over a distance of more than 40 km, the coastal cliff succession spans the Mohakatino, Mount Messenger, Urenui and Kiore formations. A composite measured section, emended after King et al. (Citation1993), has a total stratigraphic thickness of c. 1900 m ().
Figure 2. Simplified north Taranaki coastal stratigraphy. The coastal outcrop exposes the volcaniclastic Mohakatino Formation, the dominantly siliciclastic sandstone Mount Messenger Formation, the dominantly siltstone Urenui Formation and the sandstone and conglomerate Kiore Formation. Locations of dated volcanic ash samples (stars; see ) and bioevents (dots and labelled dashed lines; see ) are indicated. Stratigraphic thicknesses adapted from King et al. (Citation1993). Outcrop locations indicated in bold to the right.
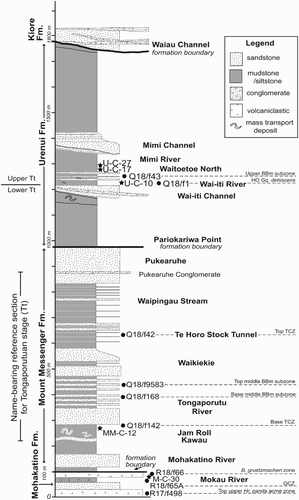
Table 2. Bioevents and biostratigraphic ages.
With the exception of the Kiore Formation, the north Taranaki outcrop succession largely comprises gravity-flow deposits, primarily turbidites, and hemipelagic sediments collectively deposited on the basin floor, base of slope and continental slope (King et al. Citation1993, Citation2007a). Intervals of mass-transport deposits, at bed- to seismic-scale, occur throughout the depositional succession (King et al. Citation1993, Citation2011; Browne et al. Citation2006; Sharman et al. Citation2015).
The Mohakatino Formation is dominated by volcaniclastic sandstone and mudstone re-worked by turbidity currents and submarine landslides from eruptive detritus derived from a coeval volcanic arc located offshore, to the northwest of the present-day coastline (e.g. Nodder et al. Citation1990; King et al. Citation1993; Giba et al. Citation2013; Sharman et al. Citation2015). The overlying formations consist of siliciclastic sediments derived from the eastern and/or southern hinterlands of the basin. The Mount Messenger Formation is sand dominated (King et al. Citation1993; Arnot et al. Citation2007a, Citation2007b; Browne et al. Citation2007a, Citation2007b; Rotzien et al. Citation2014), and the overlying Urenui Formation is mostly siltstone although broad channels within the formation also contain sandstone and conglomerate (King et al. Citation1993, Citation2007a, Citation2007b; Maier Citation2012). The Kiore Formation largely comprises channelised deposits of interbedded sandstone and siltstone, and minor conglomerate (Griffin Citation2001; Vonk et al. Citation2002; Townsend et al. Citation2008).
Volcaniclastic sediments and volcanic ash beds are common throughout the exposed coastal cliff section in north Taranaki, and are the primary source of minerals potentially suitable for radiometric dating (Bergman et al. Citation1990; King et al. Citation1993; Hansen Citation1996). Although the main phase of coeval arc volcanism is reflected in the coarse-grained volcaniclastic sediments of the Mohakatino Formation, eruptions continued episodically for a long period thereafter as evidenced by thin volcanic ash beds and disseminated volcanic ash material preserved within fine-grained, siliclastic intervals of the Mount Messenger and Urenui formations.
Beneath the nearby Taranaki peninsula, a laterally equivalent Mount Messenger and Urenui succession contains various petroleum-producing reservoirs. Although these are not as volumetrically significant as some other reservoirs in the basin, this interval nevertheless constitutes an important exploration play (King & Thrasher Citation1996).
Paleobathymetry
Paleo-water depths generally shoal upwards through the depositional stratigraphy (King et al. Citation1993). Recalibrated key benthic foraminiferal depth markers identified in unpublished GNS file data show that the Mohakatino Formation and the lower and middle intervals of the Mount Messenger Formation, between Awakino and Rapanui Stream, were deposited within deep lower bathyal water depths with a nominal range of 3000–1500 m. Water depths shoal upwards through the overlying upper Mount Messenger interval and Urenui Formation, between Rapanui Stream and Mimi River, from lower bathyal (1500–1000 m) to uppermost bathyal water depths (400–200 m). Reworked faunal material, mostly sourced from higher on the slope, is present; reworked shelfal material is noted in some samples within a slump-disrupted Mount Messenger interval (e.g. Q18/f34 and Q18/f72 in King et al. Citation1993). Reworked shallow-water shelfal material occurs in some Urenui and Kiore formation samples (e.g. Q19/f3 in King et al. Citation1993). Due to faunal reworking, water depth indicators for the Kiore Formation range from uppermost bathyal (400–200 m) to shelfal (200–0 m). We infer that the formation was deposited just below or near the paleo-shelf break.
Previous biostratigraphic correlations and dating
Prior to 1970, biostratigraphic studies of the north Taranaki coastal cliff exposures focused primarily on the upper Miocene Tongaporutu Formation (later renamed and included as a part of an expanded Mount Messenger Formation), the type locality for which was informally designated as ‘the coast between Kawau and Whitecliffs’ (Fleming Citation1959). The particular focus was on identification of benthic foraminiferal and deep-water molluscan species that were potentially useful for the regional correlation and subdivision of the Tongaporutuan Stage (e.g. Allan Citation1933; Finlay & Marwick Citation1940, Citation1947; Gibson Citation1963, Citation1967). At the time, the underlying Mohakatino Formation (north of Kawau) was by default assumed to be middle Miocene (Waiauan or older). In subsequent years, the benthic faunal criteria that Finlay & Marwick (Citation1940) listed for the recognition of the Tongaporutuan Stage were deemed to be biostratigraphically unreliable, except for the appearance of taxa in the Bolivinita quadrilatera group (Hornibrook et al. Citation1989) and of Bolivinita pohana (King et al. Citation1993).
Studies of upper Miocene strata at Kaiti Beach on the North Island's east coast (e.g. Scott Citation1992, Citation1995; Crundwell Citation2004) record the earliest appearance of Bolivinita pohana and other Bolivinita species in New Zealand (Cooper Citation2004). They also revealed the base of the Tongaporutuan was better identified from planktic foraminiferal events, including the highest common occurrence of Paragloborotalia mayeri and the close proximity of the Kaiti Coiling Zone, a discrete interval in which the taxon Globoconella miotumida is dextrally coiled. The base of the Kaiti Coiling Zone is now adopted as the primary faunal criterion for the base of the Tongaporutuan Stage (Crundwell et al. Citation2004). A complicating factor though is that two other younger dextral coiling zones of Globoconella miotumida (Mapiri and Tukemokihi) have been recognised in this lineage in the late Miocene, which poses problems for correlation if the coiling zone cannot be specifically identified. This was resolved with the establishment of an integrated planktic foraminiferal bolboformid zonation that better constrains and discriminates the respective biostratigraphic events (Crundwell et al. Citation2004).
Paragloborotalia mayeri is absent from the north Taranaki coastal cliff section (Gibson Citation1963; Scott Citation1992), but a zone of dextral coiling of Globoconella miotumida is present at the Tongaporutuan reference section. The bolboformids and ancillary planktic foraminiferal criteria demonstrate that this zone correlates with the youngest of the three known dextral coiling zones (Tukemokihi) and is stratigraphically higher (younger) than the Kaiti Coiling Zone. An important consequence of this recognition is that some or all of the deposits within the coastal cliff section below the informal Tongaporutuan reference section are now correlated with the Tongaporutuan Stage.
Previous radiometric dating
Previous attempts to radiometrically date the cropping-out north Taranaki volcanic ashes using K–Ar methods yielded imprecise ages that were generally inconsistent with biostratigraphic dating and the stratigraphic order of beds (e.g. Bergman et al. Citation1990; Hansen Citation1996). Bergman et al. (Citation1990) obtained one K–Ar age from hornblende separates of 22.1 ± 2.4 Ma from a sample in the Mohakatino Formation at the mouth of the Awakino River. Additional samples from inferred stratigraphically equivalent strata to the north (and outside of our study area) yielded ages as low as 14.5 ± 1.6 Ma (Bergman et al. Citation1990). The older age (22.1 ± 2.4 Ma) was considered to reflect incorporation of older hornblendes from a precursor intrusive event, rather than the eruptive episode itself.
Hansen (Citation1996) determined K–Ar ages from hornblende separated from five ash beds in the Mohakatino and Mount Messenger formations and reported ages that ranged from 15.12 ± 0.38 Ma to 6.94 ± 0.17 Ma, with weight percent K2O of 0.23–0.45. Although the K–Ar ages determined by Hansen (Citation1996) were individually more precise than those determined by Bergman et al. (Citation1990), they covered a broad age range. Moreover, the measured ages were not in stratigraphic order and did not agree with biostratigraphic ages from the same section. The results were attributed by Hansen (Citation1996) to be the result of argon loss and/or excess argon, or to entrained material.
Methods
Identification and sampling of volcanic ash layers
Volcanic ash beds in the north Taranaki outcrop section form continuous bands of coarse-grained material which comprise iron-rich volcanic glass and minerals that weather to a yellow to orange colour, in contrast to the typically grey-coloured siltstone within the Urenui and Mount Messenger formations (). On fresh surfaces the volcanic ash layers are light tan to white in colour. They commonly occur in groups of three to ten beds, individually c. 2–20 cm thick (). Although megascopically similar in appearance to coarse-grained sand at outcrop scale, the volcanic ash layers contain altered glass shards, pumice lapilli, euhedral phenocrysts and a general lack of quartz and current structures that distinguishes them from turbidite volcaniclastic sand layers.
Figure 3. Volcanic ash samples. A, Outcrop photograph of volcanic ash sample U-C-10 from the coastal cliff, Wai-iti River mouth, true left bank. Hammer circled for scale. B, Measured section from outcrop in Part A. Star represents sampled ash bed. C, Detail hand sample photograph of sampled ash bed. D, Photomicrograph of a thin section from volcanic ash sample U-C-10 in plain light. Plagioclase feldspar grains are white to grey, and hornblende grains are weakly pleochroic. E, Photomicrograph of the thin section in Part D in cross-polarised light. Plagioclase grains show zoning.
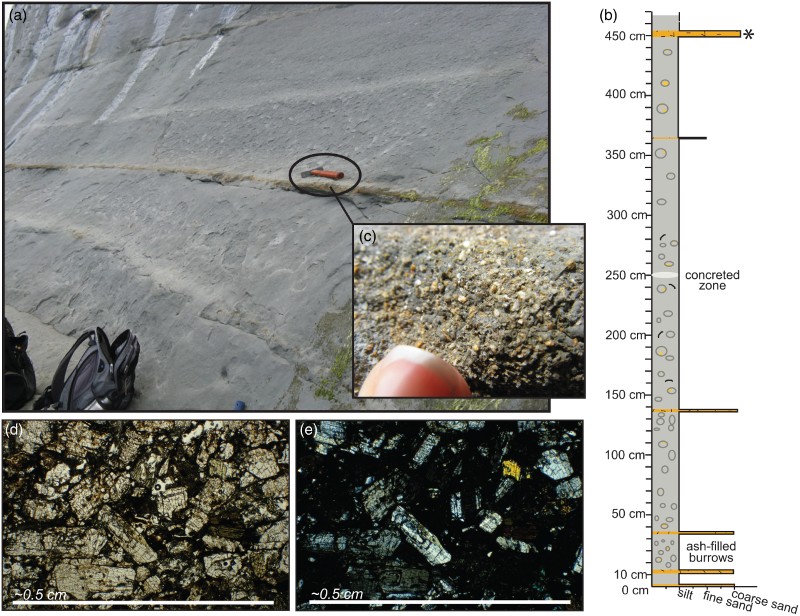
The volcanic ash beds contain abundant coarse-grained phenocrysts, including euhedral plagioclase feldspar, hornblende and pyroxenes that are significantly larger than the surrounding silt and fine- to very-fine-grained sand (). One volcanic ash bed was identified in the volcaniclastic sediments of the Mohakatino Formation (M-C-30; ; ) by the presence of c. 5–20 mm diameter pumice lapilli. Similar-sized pumice lapilli are occasionally present in other ash layers within the lower Mount Messenger Formation. Individual volcanic ash beds are either normally graded or massive and are variably but frequently bioturbated. The mixing of bioturbated detrital material from surrounding sediments made it difficult to sample only pure volcanic material in some instances.
Samples were collected from the thickest accessible volcanic ash beds with the least weathering and bioturbation. Weathered surfaces were removed from each sample to minimise alteration effects and detrital zircon contamination. At least one kilogram of volcanic ash was collected at each site.
In this study, 14 individual volcanic ash beds were sampled at 14 separate locations (; ). Twelve of the samples were taken from the coastal cliff outcrop, spanning from the top of the Mohakatino Formation at the Mokau River mouth in the north through to the middle of the Urenui Formation near the Mimi River mouth in the south. Two additional volcanic ash beds, one each from the Mount Messenger and Urenui formations, were sampled at separate inland exposures. Only five of the twelve coastal outcrop samples yielded useable ages (), but these included the uppermost and lowermost samples and therefore provided a representative span of eruptive events within the section. The stratigraphic positions of these five samples are shown on .
Mineral separations
Zircon grains for U–Pb analysis were separated from each volcanic ash sample generally following the procedures of DeGraaff-Surpless et al. (Citation2002). All samples were initially crushed and disaggregated by grinding only, then sieved and run through a Gemini water-table to concentrate the heavy minerals. The heavy mineral concentrates were then soaked overnight in hydrogen peroxide and acetic acid to dissolve carbonates, clays and organic material, and then were ultrasonically cleaned in de-ionised water for approximately one hour. Less magnetic minerals were concentrated using vertical and slope Frantz magnetic separators, then zircon grains were concentrated from this fraction using methylene iodide (MEI) heavy liquid (ρ = 3.2 g cm–3). To minimise the possibility of detrital contamination, well-preserved euhedral zircon crystals (inferred to be volcanic) were selectively extracted by hand in preference to rounded or sub-rounded zircon grains that showed signs of weathering and traction transport.
Feldspar grains were also separated for 40Ar/39Ar dating using a similar concentration and purification process as that for zircons, on a c. 200 g aliquot reserved from each disaggregated sample of volcanic ash. After sieving to obtain a 0.5–1.0 mm size fraction, followed by the concentration of least magnetic grains, lithium metatungsate (LMT) heavy liquid (ρ = 2.61 g cm–3) was used to separate the feldspar fraction. Most of the 14 volcanic ash samples contained mineral grains that appear megascopically and petrographically similar to plagioclase, rather than potassium feldspar. Compared to plagioclase, biotite or hornblende, potassium feldspar is better suited for 40Ar/39Ar analysis because this mineral has inherently high potassium, argon retention and radiogenic yield (i.e. %40Ar*, the accumulation of radiogenic 40Ar from decay of 40K over time in a closed system) and is less prone to secondary alteration (e.g. McDougall & Harrison Citation1999). In an attempt to isolate any potassium feldspar grains, we selected transparent euhedral grains that were free of visible alteration for chemical analysis to determine their potassium content and dating potential.
Feldspar analyses
Representative mineral grains with a density of 2.60–2.59 g cm–3 were analysed for modal chemistry using energy dispersive X-ray (EDAX) and electron microprobe in the Laboratory for Advanced Materials at Stanford University, California. Initial qualitative EDAX measurements were conducted on two to four grains from five representative samples, and results suggested that some of the transparent, euhedral grains were hydrated minerals (stoichiometrically not feldspar). Subsequently, quantitative analyses of representative grains from seven samples were acquired with the electron microprobe for a total of 33 point analyses (Table S1). Electron microprobe analytical results indicated intermediate albite- to andesine-composition plagioclase based on the orthoclase, albite and anorthosite content, and four of seven samples revealed very low potassium content. There is low potassium content overall in all analysed feldspars (0.01–0.31 wt% K20) (Tables 1, S1).
Due to the pervasive alteration of the Taranaki volcanic ash layers and the possibility of argon loss due to heating during burial and later volcanism in the Taranaki region (e.g. Armstrong et al. Citation1996), 40Ar/39Ar ages determined from the feldspar grains would likely not represent the original eruptive age. The low potassium content of the analysed feldspar grains is unfavourable for high-precision argon analyses due to the small expected 40Ar signals (e.g. McDougall & Harrison Citation1999). Consequently, no 40Ar/39Ar analyses were performed. Instead, U–Pb ages were determined from zircon grains, which are more resistant to secondary alteration and reheating than feldspar minerals (Fedo et al. Citation2003).
Zircon analyses
Uranium–lead zircon ages were analysed using the sensitive high-resolution ion microprobe-reverse geometry (SHRIMP-RG) at the Stanford-US Geological Survey Micro Analytical Center. Individual zircon grains were mounted in c. 2.3 cm epoxy disks, polished to expose the zircon cores and imaged with cathodoluminescence (CL) using a JEOL 5600LV scanning electron microscope (SEM) at Stanford University. The objective of the zircon U–Pb dating strategy was to identify the youngest, volcanically derived zircon from the volcanic ash layers (i.e. the eruption age). To accomplish this, a screening procedure was conducted by measuring preliminary uncorrected 206Pb/238U ages using a rapid analysis routine with 1 s counting times for 90Zr2O, 204Pb, 207Pb, 208Pb, 232Th, 248ThO, 238UO and 238UO2 and 5 s for 206Pb and 238U (<1 minute total analysis time). The screening was concentrated on zircons that exhibited euhedral to subhedral morphology or oscillatory zoning in CL images. This screening approach modified the procedure utilised for identifying Hadean-aged zircons described by Holden et al. (Citation2009), in order to focus only on zircon with 206Pb/238U values lower than c. 64 that would indicate ages of less than c. 100 Ma. Although the error in calculated 206Pb/238U age during the screening process was relatively high (c. 10–50%) due to the short counting times and lack of common Pb correction, it was adequate to differentiate between young volcanic grains and older detrital grains, which are generally >100 Ma in Taranaki samples.
Zircon grains with an uncorrected 206Pb/238U age of less than c. 100 Ma identified during the screening procedure were reanalysed with longer count times on Pb and U to measure U–Pb ages with higher precision. Errors were greatly reduced by reanalysing the young zircon grains with longer counting times and applying common Pb corrections. A total of 49 grains were reanalysed; 4 zircons yielded significantly older ages (112–217 Ma) and 45 analyses on 5 ash samples yielded young ages (c. <15 Ma), allowing a clear differentiation between a population of volcanic grains interpreted to represent the contemporary eruption age and a population of older grains interpreted to represent detrital contamination from surrounding slope sediments (Table S2). Subsequent analyses by laser-ablation inductively coupled plasma mass spectrometry (LA-ICP-MS) of clastic detrital samples from the Urenui Formation reveal an absence of Miocene-aged zircon populations, confirming that the prevalent source of the background slope sediments is unrelated to coeval volcanism (Maier Citation2012).
All reported 206Pb/238U ages were corrected for common-Pb using 207Pb based on a model Pb composition from Stacey & Kramers (Citation1975), and calibrated using the R33 zircon standard (419 Ma, Black et al. Citation2004). Interpreted model ages are reported as weighted mean and 2σ standard error, and calculated using the programme SQUID1.13 (Ludwig Citation2001) and Isoplot3.41 (Ludwig Citation2003). Full data tables as well as Tera–Wasserburg concordia diagrams are available in Table S2. All SHRIMP-RG measurements were made with a primary 16O2– beam ranging 5-6 nA. Errors associated with the U–Pb weighted mean zircon ash ages are calculated with the SQUID1.13 programme, which includes uncertainty in the reproducibility of standard measurements (R33) added in quadrature to the uncertainty of the unknown (Ludwig Citation2001).
Biostratigraphic dating
Planktic foraminiferal and bolboformid bioevents have been identified in the New Zealand region, correlated to magnetostratigraphic data from the Ocean Drilling Programme (ODP) Site 1123 on Chatham Rise, east of New Zealand, and calibrated to the geomagnetic polarity time scale (Crundwell Citation2004; Gradstein et al. Citation2012). A Shaw-plot (Shaw Citation1964) of 33 late Miocene bioevents that are found at ODP Site 1123 and Deep Sea Drilling Project (DSDP) Site 593 on the Challenger Plateau, west of New Zealand (r2 = 0.9908), indicates homotaxial order is maintained and that the bioevents are very reliable (isochronous) biochronological markers in the greater New Zealand region (Crundwell & Nelson Citation2007). We have reviewed and identified equivalent biostratigraphic datums from the upper Miocene north Taranaki coastal section, using micropaleontological samples (mostly faunal slides) archived in the National Paleontological Collections at GNS Science, Lower Hutt, New Zealand. Micropaleontological samples examined include collections by Gibson (Citation1963, Citation1967), G.H. Scott in King et al. (Citation1993) and high-resolution suites of samples collected by M.P. Crundwell (unpublished). The different collections were integrated on the basis of the relative stratigraphic positions of each sample, based on sample locations shown on maps in Gibson (Citation1963) and King et al. (Citation1993) and on aerial photographs. A total of 241 samples have been examined from the coastal outcrop section. Although there is uncertainty concerning the precise stratigraphic position of some of the samples, and parts of the section have been more closely sampled than others, the relative stratigraphic order of the samples enables most bioevents to be located to an accuracy of ≤±8 m. Nine bioevents are recognised from the suite of 241 samples. These bioevents are listed in , with adopted stratigraphic positions and thicknesses based on measured sections in King et al. (Citation1993).
Results
Radiometric ages
Of the 14 volcanic ash samples collected, 10 contained zircon grains after heavy liquid mineral separation. Five of these 10 samples yielded Miocene-age grains (), including a total of 41 zircon grains with individual U–Pb ages ranging from 7.3 ± 1.1 to 11.3 ± 1.4 Ma , 1σ (; Table S2). Ages for most individual single-grain analyses are relatively imprecise due to low U concentration (typically <100 ppm) and 0–25% common Pb contamination. However, sample U-C-10 yielded two analyses with high U concentrations (665 and 727 ppm), although also with high common Pb (42–57% Pbc). Despite these challenges, calculated weighted mean U–Pb zircon ages for each sample are consistent with the observed stratigraphic sequence given the 2σ uncertainty (, 4). The calculated 207Pbc-corrected 206Pb/238U zircon ages from samples U-C-17, MM-C-12 and M-C-30 are statistically distinguishable at 2σ (). The two oldest calculated weighted mean ages are from the Mohakatino Formation (10.63 ± 0.65 Ma; M-C-30) and the lower Mount Messenger Formation (9.66 ± 0.29 Ma; MM-C-12). These ages are differentiable within 2σ standard errors and interpreted to represent two separate eruptive ages recorded in different deposits separated by c. 185 m (; , , ). Ages from the three youngest samples (U-C-27, U-C-17, U-C-10) are stratigraphically separated by less than 100 m in the Urenui Formation and overlap in age at 2σ (, , ). The most precise age (8.97 ± 0.22 Ma; U-C-17) is used to represent depositional age for this part of the section (–). Although there is scatter in individual single-grain zircon ages within all five samples, the weighted mean ages show a progressive younging in age of the three sampled formations ().
Figure 4. 207Pbc-corrected 206Pb/238U ages from volcanic zircon grains in ash samples measured on the SHRIMP-RG. Single-grain ages (dots) are shown with 1σ errors (standard deviation). Thick vertical lines are calculated weighted mean ages for each sample, and the shaded grey areas represent 2σ standard error. Samples are shown in stratigraphic order. See for additional details.
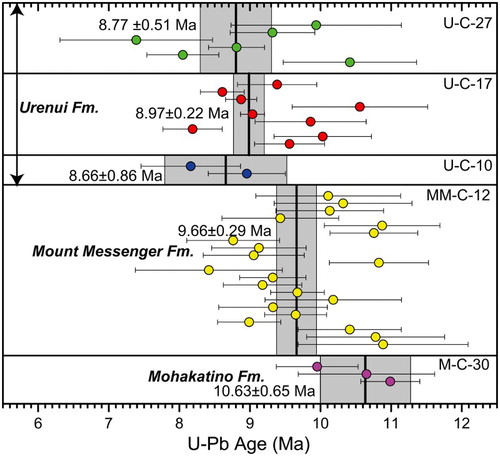
Some detrital zircons with age populations of >100 Ma are present in the volcanic ash separates. These reflect bioturbation and mixing with the host deep-water clastic sediments, which in turn are reworked and derived from other older sources (Maier Citation2012).
Biostratigraphic ages
Virtually the entire outcrop succession from Awakino to west of Urenui is biostratigraphically dated as Tongaporutuan (). Age-calibrated planktic foraminiferal and bolboformid datum levels (biostratigraphic events) used in the dating of the composite north Taranaki coastal cliff section are described in the following, from oldest to youngest. Stratigraphic positions are measured in metres above the base of the composite section (, 5).
Figure 5. Summary of new U–Pb and biostratigraphic ages and calculated sedimentation rates, with previously determined K–Ar ages for comparison. Three ash samples (M-C-30, MM-C-12, U-C-17) whose weighted mean U–Pb ages are differentiable at 2σ standard error are plotted (stars, with 2σ standard error depicted by horizontal lines). Biostratigraphic datums are plotted as solid dots (and in one case also with age ranges depicted by a horizontal line with end ticks). K–Ar ages from Hansen (Citation1996, unpublished MSc thesis) are plotted as grey squares with error bars. Our new volcanic ash and biostratigraphic event ages are independently derived, compatible with each other and generally consistent with inferred stratigraphic superposition. Our results provide much tighter constraints on the age of the north Taranaki coastal cliff section than previously available data, and demonstrate that the original Tongaporutuan reference section has a very narrow age range. Apart from a few metres at the base of the outcrop, the entire measured section is Tongaporutuan. An approximate age–thickness trend is drawn (dashed line) through the new data, revealing two intervals with broadly different sedimentation rates (relatively low in the Mohakatino and lower Mount Messenger formations and an order of magnitude higher in the upper Mount Messenger and Urenui formations).
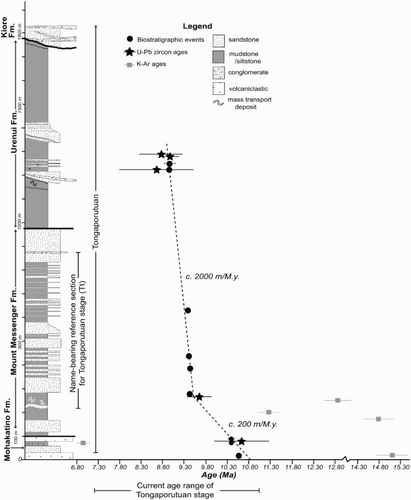
Top upper Hirsutella panda acme zone (10.65 Ma), c. 15 m
The highest common occurrence of Hirsutella panda, where the nominate species comprises 5% or more of the total planktic foraminiferal fauna, is noted in sample R17/f498 (15 m; ) that was collected from a thin volcanogenic sandstone bed on the south head of Awakino River (), approximately 15 m above the base of the Purupuru Tuff (Mohakatino Formation). Notwithstanding the sandy volcanogenic lithology, sample R17/f498 contains a rich planktic foraminiferal fauna with common Hr. panda in conjunction with a sinistrally coiled population of Globoconella miotumida and rare specimens of Paragloborotalia mayeri s.l. This combination of taxa indicates this sample is correlated with the top of the upper Hr. panda acme zone, above the stratigraphic top of the Mapiri Coiling Zone, and that it has a correlated age of 10.65 Ma (Crundwell Citation2014; , 5).
Glomar Coiling Zone (adopted age 10.39 Ma), 74 m
Dextrally coiled specimens of Neogloboquadrina incompta (0S:2D) in sample R18/f65A (74 m), the only specimens of Nq. incompta that have been identified in the lower part of the coastal section, are correlated with the upper part of the Glomar Coiling Zone (GCZ), above the stratigraphic level of the lowest common occurrence of species associated with the Nq. pachyderma-incompta group. This interval has a correlated age of 10.36–10.39 Ma (Crundwell Citation2014; , 5). The presence of Bolboforma gruetzmacheri in an overlying sample (see the following section) restricts the biostratigraphic age of sample R18/f65A to 10.39 Ma, the age adopted in this study.
Bolboforma gruetzmacheri range zone (adopted age 10.39 Ma), 91 m
Specimens of Bolboforma gruetzmacheri that are restricted to the B. gruetzmacheri range zone (10.39–10.55 Ma) are noted in a single sample, R18/f66 (91 m), from the upper part of the lower Mohakatino Formation on the faulted south head of the Mokau River (). The correlation of an underlying sample with the upper part of the GCZ (see previous section) further restricts the age of sample R18/f66 to 10.39 Ma ().
Base Tukemokihi Coiling Zone (9.45 Ma), 279 m
A distinctive 326 m thick interval, where 20% or more of specimens of Globoconella miotumida are dextrally coiled, is noted between Tongaporutu River and the Te Horo stock tunnel (a tunnel located between Waipingau Stream and Whitecliffs that provides access to the beach; King et al. Citation1993). The identification of Bolboforma metzmacheri s.s. in some samples from the lower part of this interval indicates that the dextrally coiled populations of Gc. miotumida are associated with the Tukemokihi Coiling Zone (TCZ), the youngest of three known late Miocene intervals of dextrally coiled Globoconella miotumida (Crundwell et al. Citation2004). Previous studies of the coastal section (e.g. King et al. Citation1993) have incorrectly correlated this interval with the Kaiti Coiling Zone, the oldest of the three known intervals of dextrally coiled Gc. miotumida which occurs at the base of the New Zealand Tongaporutuan Stage (Crundwell et al. Citation2004; Crundwell Citation2014). In the north Taranaki coastal section, the base of the TCZ is identified in sample Q18/f142 (279 m) in slump-folded turbidite beds on the south side of the Tongaporutu River estuary, 8 m below the base of the thick-bedded Tongaporutu River sandstone, and has a calibrated age of 9.45 Ma (Crundwell Citation2014; Figures 2, 5). The presence of sinistrally coiled populations of Gc. miotumida in some samples from the lowermost part of the TCZ introduces a degree of uncertainty regarding the precise level of the base of the TCZ, but as a whole it does not adversely affect the biostratigraphic dating.
Base middle Bolboforma metzmacheri s.s. subzone (9.44 Ma), 387 m
Specimens of Bolboforma metzmacheri s.s. are noted in several samples in the vicinity of Ohanga Stream, within the lower part of the Tukemokihi Coiling Zone (TCZ). These bolboformid occurrences are confined to a relatively thin stratigraphic interval that is correlated with the middle B. metzmacheri (BBm) subzone, one of only three known BBm subzones and the only BBm subzone within the lower part of the TCZ (Crundwell Citation2004). The base of the middle BBm subzone occurs in sample Q18/f168 (387 m), at the base of an interval of thin-bedded compensatory-style alternating sandstone and mudstone described and illustrated in Browne et al. (Citation2007a, figure 12), and has a correlated age of 9.44 Ma (Crundwell Citation2014; , 5).
Top middle Bolboforma metzmacheri s.s. subzone (9.42 Ma), 438 m
As noted above, the middle Bolboforma metzmacheri s.s. subzone is recognised in the vicinity of Ohanga Stream. The top of this BBm subzone occurs in sample Q18/f9583 (438 m), and has a correlated age of 9.42 Ma (Crundwell Citation2014; , 5).
Top Tukemokihi Coiling Zone (9.39 Ma), 632 m
The stratigraphically highest (youngest) population of dextrally coiled Globoconella miotumida marks the top of the Tukemokihi Coiling Zone (TCZ). It occurs in the northern part of Pukearuhe Beach in sample Q18/f42 (632 m) and has a correlated age of 9.39 Ma (Crundwell Citation2014; , 5).
Highest occurrence Globoquadrina dehiscens (8.96 Ma), 1225 m
In the composite coastal section, the highest recorded occurrence of the planktic foraminifera Globoquadrina dehiscens is noted in sample Q18/f1 (1225 m), several hundred metres above the stratigraphic level that King et al. (Citation1993) assign to the boundary between the Mount Messenger and Urenui formations. Previously, the base of the Urenui Formation had been located (in outcrop) stratigraphically lower at the base of the Pukearuhe Conglomerate (e.g. Gibson Citation1963). Conversely, in petroleum exploration wells in the Taranaki Basin the highest occurrence of Gq. dehiscens is often used as a proxy for the top of the Mount Messenger Formation (Crundwell Citation2008; Morgans Citation2008). The highest occurrence of Gq. dehiscens, which has a calibrated age of 8.96 Ma (Crundwell Citation2014; , 5), is also used as the primary biostratigraphic marker for the informal subdivision of the New Zealand Tongaporutuan Stage into lower and upper sub-stages (Raine et al. Citation2015).
Upper Bolboforma metzmacheri s.s. subzone (adopted age 8.85–8.96 Ma), 1251 m
A single specimen of Bolboforma metzmacheri s.s. is noted in sample Q18/f43 (1251 m), in the lower part of the Urenui Formation. The stratigraphic position of this bolboformid occurrence above the highest occurrence of Globoquadrina dehiscens (see above) indicates it is correlated with the upper part of the upper B. metzmacheri s.s. (BBm) subzone and that the biostratigraphic age of the sample is 8.85–8.96 Ma (Crundwell Citation2014; , 5).
Discussion
Comparison of radiometric and biostratigraphic ages
Despite our selection of only euhedral zircon grains to minimise the possibility of detrital contamination, the uranium–lead ages of individual zircon grains are variable for each volcanic ash sample (). This scatter in the data is analytical and largely due to the low U content of the zircon and common Pb contamination. Within uncertainties, the weighted mean averages of the zircon age populations from each dated ash layer in the coastal section show an overall younging trend up-section, as expected from their relative stratigraphic positions. Additionally, the mean radiometric ages are in close agreement to ages inferred for (and interpolated between) biostratigraphic datums identified at comparable stratigraphic positions within the same outcrop succession (). Considering all of these factors, we interpret the zircon crystallisation ages in each Taranaki volcanic ash sample to be from a single population and representative of an eruptive age.
As described above, the radiometric and biostratigraphic ages are independently derived. Due to the agreement of the two types of independent ages the radiometric results provide corroboration of the local foraminifera bioevent dating scheme, which gives confidence for applicability of the latter beyond the study area.
Tongaporutuan reference section
The succession exposed in coastal cliffs between Kawau and Whitecliffs (Pukearuhe conglomerate) is the name-bearing reference section for the Tongaporutuan Stage but is not suitable as a conventional Stratotype Section and Point (SSP) for the base of the stage. The succession is offset by post-depositional faults with uncertain offsets, and is punctuated by intra-formationally slumped and deformed beds with uncertain stratigraphic contiguity. In addition, there are many headlands around which it is impossible to correlate and measure the stratigraphy bed by bed. In these regards, the measured thickness presented here for the interval equivalent to the Tongaporutuan reference section can only be regarded as a close approximation. Nevertheless, our results indicate that this c. 650 m thick interval spans a comparatively short time interval of c. 0.7 Ma (9.8–9.1 Ma, interpolated from our sample ages, ), which corresponds to only a fraction of the Tongaporutuan Stage (11.04–7.2 Ma; Raine et al. Citation2015). Potentially the entire outcrop section along the north Taranaki coast could be considered as the stratotype. However, this would still be unsatisfactory in that the key biostratigraphic criteria that mark the base of the Tongaporutuan Stage have not been recorded in this succession (see above). As it stands, composite biostratigraphic criteria from different geographic localities are used to recognise and subdivide the Tongaporutuan Stage (Crundwell et al. Citation2004). An SSP has yet to be defined for the base of the Tongaporutuan, but will almost certainly be located on the North Island's east coast (Cooper Citation2004). However, the north Taranaki section may well serve as an SSP for subdividing the stage.
Sedimentation rates
The combined high-resolution biostratigraphy and new U–Pb volcanic ash radiometric dating provide a robust basis for calculating sedimentation rates for this ancient progradational continental margin succession. An approximate trend line describing sediment age versus stratigraphic thickness can be drawn through the combined datasets (dashed lines, ). Two distinct intervals are evident with quite different trends in stratigraphic duration versus sediment thickness (i.e. sedimentation rates). Calculated average sedimentation rate (un-decompacted) for the Mohakatino and lower Mount Messenger formations is c. 200 m Ma–1, whereas the rate for the upper Mount Messenger and Urenui formations is an order of magnitude higher, c. 2000 m Ma–1 ().
The boundary between the two intervals represented by the inflection point (change in slope of the sedimentation rate trend) is at c. 280 m stratigraphic height, roughly coincident with the top of a relatively thick mass-transport deposit (MTD) interval within the lower Mount Messenger Formation (). This boundary is nominally dated at c. 9.5 Ma. However, it is possible that a depositional hiatus or erosional unconformity is present at the top of the MTD. If considerable sediment has been eroded, it would imply that the very high sedimentation rates started somewhat earlier, because there is only a maximum period of time of c. 0.23 Ma that may be missing (unrepresented) in the section. Otherwise, the overall sedimentation rate trends would not be unduly affected by a relatively small amount of missing section across the boundary at c. 280 m.
In general, large variations in un-decompacted sedimentation rates observed in deep-water depositional systems have been attributed to changes in depositional environment, climate, proximity to the shelf and sediment flux (e.g. Müller & Suess Citation1979; Johnson-Ibach Citation1982). Likewise, the increase in Taranaki sedimentation rates is associated with an upwards-shoaling section in which the lower Mount Messenger and Mohakatino formations represent deeper-water and more distal depositional environments in which sediments accumulated more slowly than the overlying sediments from shallower depositional settings (King et al. Citation1993, Citation2007a). The higher sedimentation rates are compatible with the interpreted slope and base-of-slope paleo-environments and overall progradation of the depositional system (King et al. Citation1993, Citation2007a, Citation2007b). High sedimentation rates likely contributed to slope destabilisation and mass wasting commonly observed within middle–late Miocene Taranaki Basin deposits (Utley Citation1987; Nodder et al. Citation1990; King et al. Citation1993, Citation2011; Browne et al. Citation2006; Sharman et al. Citation2015). It is possibly no coincidence that the largest mass-transport interval within the studied succession lies just beneath the inferred change in sedimentation rates. It is also possible that syn-depositional mass-transport processes may have physically removed or excised some strata in the lower part of the section, which would contribute to the lower calculated sedimentation rates there and to the marked change in sedimentation rate trend. It is equally possible that the mass wasting was triggered by a large tectonic event, such as movement on the underlying Taranaki Fault-bound basement block or volcanic eruption. The onset of a massive influx of detritus into the area could also have been heralded by an escalation of tectonic activity farther afield.
The frequency and stratigraphic resolution of sampling in this study only allows calculation of sedimentation rates over large intervals. Our calculated un-decompacted sedimentation rates represent time-averaged accumulation rates and do not fully reflect short-term variations in depositional rates. Even higher sedimentation rates representing a range of depositional processes may therefore be represented within some parts of the succession. For example, turbidite sandstone beds, such as climbing ripple-laminated beds recognised in the Mount Messenger Formation (Browne & Slatt Citation2002), have been interpreted to represent very high instantaneous sedimentation rates over timescales of minutes (Jobe et al. Citation2012). Sand-rich stacked-lobe or channel complexes would also be expected to have overall high sedimentation rates, but would be balanced by intervening hemipelagic deposition which can be many orders of magnitude slower (e.g. Müller & Suess Citation1979; Hesse Citation1994).
The averaged, un-decompacted sedimentation rate for the upper interval presented here (c. 2000 m Ma–1) is high but reasonable in comparison with other deep-water depositional systems. Averaged sedimentation rates in other deep-water depositional systems in the Taranaki Basin, offshore the east coast of the North Island, offshore the US Pacific northwest and in the Indus Fan are commonly hundreds of metres per million years and can be up to 1500 m Ma–1 (Barnard Citation1978; Beggs Citation1990; King & Thrasher Citation1996; Hanumantha Rao et al. Citation2002; Orpin Citation2004; Shane et al. Citation2006). A compaction-corrected sedimentation rate of 640 m Ma–1 determined from biostratigraphy in offshore well deposits analogous to the coastal outcrop (King & Thrasher Citation1992; King et al. Citation1993) lies between the range of rates calculated for the upper and lower outcrop sections in this study ().
Interpretation of volcanicity
The volcanic ash layers are distinct from and have significantly larger grain size than the surrounding detrital sediments. The ash layers are inferred to be airfall volcanic ash deposits rather than volcaniclastic sediment gravity-flow deposits, due to the presence of normal grading without current structures, occurrence within fine-grained pelagic or low-energy turbidite intervals and the observation of c. 5–20 mm diameter pumice lapilli (in samples from the lower Mount Messenger and Mohakatino formations) (e.g. Sparks Citation1976; Sheridan Citation1979; Wright & Smith Citation1980; Fisher Citation1984). Following eruption and settling through the water column, the individual volcanic ash beds were minimally reworked, primarily by bioturbation mixing with the underlying and overlying detrital sediments.
Evidently, volcanic eruptions occurred contemporaneously but intermittently throughout deposition of the progradational clastic succession. Each of the individual volcanic ash beds is interpreted to represent a separate episodic volcanic event of relatively short-lived duration. Where the volcanic ash beds occur in groups separated by 1–2 m of fine-grained sediments, they may represent eruptions with recurrence intervals of a minimum of several hundreds of years, based very roughly on the long-term averaged un-decompacted sedimentation rates calculated in this study.
We infer that the mean U–Pb ages are reliable, given their compatibility with biostratigraphic ages and the broadly matched trend of reducing age up-section of both datasets. Overall, our data imply that the volcanic source was active over the period c. 11–8 Ma (). The older age is somewhat younger than that established by K–Ar dating of the Mohakatino Formation (Bergman et al. Citation1990).
The intermediate composition of plagioclase, absence of sanidine and quartz, paucity of volcanic zircon and prevalence of hornblende and clinopyroxene in the volcanic ash layers are consistent with a compositionally intermediate (andesitic) volcanic source. Based on seismic-reflection mapping, petroleum exploration drilling and derivative late Miocene tectonic reconstructions, the now-buried volcanoes of the Mohakatino arc nearby within the Northern Graben of Taranaki Basin (e.g. Bergman et al. Citation1990; Nodder et al. Citation1990; King et al. Citation1993; King Citation2000; Stagpoole & Funnell Citation2001; Giba et al. Citation2013) are the interpreted sources for the volcanic ash beds.
Eruption within the Mohakatino arc began at c. 16 Ma and progressively migrated southwards over time (e.g. Giba et al. Citation2013). During the period 12–8 Ma, many large volcanic edifices were present to the west and northwest of our study area (Giba et al. Citation2013). Individual volcanoes have been drilled by petroleum exploration wells and have been radiometrically dated. The Kora volcano has hornblende K–Ar ages ranging from 8.0 ± 0.9 Ma to 19.5 ± 4.2 Ma (Bergman et al. Citation1992). The age range is broad, and individual sample ages are imprecise. On balance, the data can be interpreted as indicating an earlier intrusive magmatic phase during c. 20–17 Ma and a later eruptive phase during c. 14–7 Ma (Bergman et al. Citation1992). The overall age range of the volcanic ash beds in this study (c. 11–8 Ma) agrees with the age of the buried Mohakatino arc, as determined from seismic stratigraphy and summarised in Giba et al. (Citation2013).
Application of radiometric dating methodology
Any radiometric dating of sedimentary sections has to consider the effect of weathering and alteration on sample composition. For measurements of radiometric depositional ages, it is important to apply screening procedures to identify suitable material for analysis. We recommend undertaking initial quantitative screening of potassium content in feldspar to avoid analysis of low-potassium minerals that would yield low-precision ages. Potential alteration of potassium-bearing minerals such as plagioclase and hornblende should also be carefully evaluated. Alteration effects and low radiogenic 40Ar yield likely explain the large errors and inconsistent ages previously reported for K–Ar analyses from these outcrops.
Zircon has the potential to retain the original eruptive age, even in deeply buried, thermally disturbed and strongly altered sedimentary successions (e.g. Fedo et al. Citation2003). In our study, volcanic ash and detrital zircon grains were difficult to distinguish optically because they were of similar size, unlike the plagioclase and hornblende grains that were significantly larger in the volcanic ash beds than in surrounding detrital material. The initial zircon screening procedure used here allowed volcanic grains to be quickly differentiated from older detrital contaminants. The technique may be especially useful in studies of sediment provenance in New Zealand basins, given that differences in age between the basin-fill strata and volcanic ashes can be c. >100 Ma (Mortimer Citation2004; Adams et al. Citation2009a, Citation2009b; Maier Citation2012).
Most immediately, the dating methodology from this study could be applied to further dating along the north Taranaki coast, especially to the north in the Mohakatino Formation where there have been few dating attempts and where the tectono-stratigraphic evolution in this part of the basin remains enigmatic. Methods similar to those utilised in this study could be applied in other areas with intermediate to silicic ash beds and are most likely to be successful in deposits with large gaps (i.e. tens of millions of years) between the oldest depositional and youngest detrital ages. As in this study, further analyses from surrounding sections might provide new radiometric ages that could also be used to compare, confirm and refine available ages of documented biostratigraphic events.
The Mount Messenger and Urenui formations contain producing oil and gas reservoirs in subsurface Taranaki Peninsula. However, the stratigraphic architecture of this interval is complex, being characterised by a high degree of channelling, lobe switching and progradation. Potentially the identification and dating of other volcanic ash layers in cored exploration drillholes will provide additional markers to aid stratigraphic correlation and understanding of this economically important depositional system.
Conclusions
The entire deep-water clastic succession in the north Taranaki coastal cliff exposures studied here is Tongaporutuan in age, but includes strata above and below the Tongaporutuan reference section. New U–Pb analyses of individual zircon grains from andesitic volcanic airfall ash beds encased within the clastic turbidite deposits indicate intermittent nearby volcanic activity over the period c. 11–8 Ma. Although the radiometric age of individual zircon grains is not always highly precise, their weighted mean ages in each sample show an upwards-younging trend throughout the outcrop succession and have a strong correlation (in relative stratigraphic position) with independently determined high-resolution biostratigraphic ages. This suggests that the radiometric ages are more reliable than any previously published ages and that U–Pb zircon dating of primary ash beds can provide useful results when there is insufficient or unfavourable material for 40Ar/39Ar dating techniques. Systematic screening procedures should also be employed where there is extensive bioturbation or alteration and/or minimal volcanic zircon is available. The combined radiometric and biostratigraphic datasets reveal relatively high un-decompacted averaged sedimentation rates of up to c. 2000 m Ma–1 on the paleo-slope. The high sediment flux is consistent with paleobathymetry data and the suggested progressive infilling of the deep marine sub-basin and progradation of the slope across it.
Supplementary data
Table S1. Electron microprobe data.
Table S2. SHIRMP-RG data.
Table S2. SHIRMP-RG data.
Download Zip (494.1 KB)Table S1. Electron microprobe data.
Download MS Word (19.5 KB)Acknowledgements
The sampling and analysis for radiometric dating were conducted as part of the first author's dissertation at Stanford University. Special thanks to Zane Jobe, Elizabeth Cassel, Anne Bernhardt and Melanie Thompson for assistance during fieldwork in Taranaki; Jim Ingle for helpful discussions; Marty Grove, Joe Wooden, Jorge Vasquez, Bob Jones, Anne Bernhardt and Joe Colgan for assistance with sample preparation and analyses; and Blair Burgreen, Glenn Sharman, Charlie Paull, Don Lowe, Andrea Fildani and George Hilley for reviewing earlier drafts. Andrew Boyes (GNS Science) helped to re-draft some of the figures. We are grateful for USGS internal reviews provided by Christy Till and Elmira Wan and journal reviews provided by Hugh Morgans and Scott Nodder.
Associate Editor: Dr Kari Bassett.
Disclosure statement
No potential conflict of interest was reported by the authors.
ORCID
MP Crundwell http://orcid.org/0000-0002-6047-7017
Additional information
Funding
References
- Adams CJ, Campbell HJ, Griffin WL. 2009a. Tracing the Caples Terrane through New Zealand using detrital zircon age patterns and radiogenic isotope signatures. New Zeal J Geol Geophys. 52:223–245. doi: 10.1080/00288300909509888
- Adams CJ, Graham IJ, Seward D, Skinner DNB, Moore PR. 1994. Geochronological and geochemical evolution of late Cenozoic volcanism in the Coromandel Peninsula, New Zealand. New Zeal J Geol Geophys. 37:359–379. doi: 10.1080/00288306.1994.9514626
- Adams CJ, Mortimer N, Campbell HJ, and Griffin WL. 2009b. Age and isotopic characterization of metasedimentary rocks from the Torlesse Supergroup and Waipapa Group in the central North Island, New Zealand. New Zeal J Geol Geophys. 52:149–170. doi: 10.1080/00288300909509883
- Allan RS. 1933. On the system and stage names applied to divisions of the Tertiary strata in New Zealand. Trans New Zeal Inst. 63:81–108.
- Armstrong PA, Chapman DS, Funnell RH, Allis RG, Kamp PJJ. 1996. Thermal modeling and hydrocarbon generation in an active-margin basin: Taranaki Basin, New Zealand. Am Assoc Petrol Geologist Bull. 80:1216–1241.
- Arnot MJ, Browne GH, King PR. 2007a. Chapter 61: Thick-bedded sandstone facies in a middle basin-floor fan setting, Mount Messenger Formation, Mohakatino Beach, New Zealand. In: Nilsen TH, Shew RD, Steffens GS, Studlick JRJ, editors. Atlas of deep-water outcrops. AAPG Studies in Geology 56. Tulsa, OK: American Association of Petroleum Geologists; p. 241–244.
- Arnot MJ, King PR, Browne GH, Helle K. 2007b. Chapter 63: Channelized, innermost, basin-floor-fan morphologies, Mount Messenger Formation, Waikiekie south beach and inland, New Zealand. In: Nilsen TH, Shew RD, Steffens GS, Studlick JRJ, editors. deep-water outcrops. AAPG Studies in Geology 56. Tulsa, OK: American Association of Petroleum Geologists; p. 249–256.
- Barnard WD. 1978. The Washington continental slope: Quaternary tectonics and sedimentation. Mar Geol. 27:79–114. doi: 10.1016/0025-3227(78)90075-0
- Beggs JM. 1990. Seismic stratigraphy of the Plio-Pleistocene Giant Foresets, Western Platform, Taranaki Basin. In: 1989 New Zealand Oil Exploration Conference proceedings, Ministry of Commerce, Wellington; p. 201–207.
- Bergman SC, Atkinson CD, Talbot J, Gordon TL. 1990. Nature and reservoir potential of Miocene sedimentary and volcanic rocks, western North Island, New Zealand: a reconnaissance field and laboratory study. Open File Report PR 1581.
- Bergman SC, Talbot JP, Thompson PR. 1992. The Kora Miocene submarine andesite stratovolcano hydrocarbon reservoir, Northern Taranaki Basin, New Zealand. In: 1991 New Zealand Oil Exploration Conference Proceedings. Wellington: Ministry of Commerce; p. 178–206.
- Black LP, Kamo SL, Allen CM, Davis DW, Aleninikoff JN, Valley JW, Mundil R, Campbell IH, Korsch RJ, Williams IS, Foudoulis C. 2004. Improved 206Pb/238U microprobe geochronology by the monitoring of trace-element-related matrix effect: SHRIMP, ID-TIMS, ELA-ICP-MS and oxygen isotope documentation for a series of zircon standards. Chem Geol. 205:115–140. doi: 10.1016/j.chemgeo.2004.01.003
- Browne GH, King PR, Arnot MJ, Helle K. 2007a. Chapter 62: A complete middle-to-inner basin-floor-fan cycle, Mount Messenger Formation, Tongaporutu, New Zealand. In: Nilsen TH, Shew RD, Steffens GS, Studlick JRJ, editors. Atlas of Deep-Water Outcrops, American Association of Petroleum Geologists Studies in Geology. 56:245–48.
- Browne GH, King PR, Arnot MJ, Slatt RM. 2007b. Chapter 64: Architecture of base-of-slope fans, Mount Messenger Formation, Pukearuhe Beach, New Zealand. In: Nilsen TH, Shew RD, Steffens GS, Studlick JRJ, editors. Atlas of deep-water outcrops. AAPG Studies in Geology 56. Tulsa, OK: American Association of Petroleum Geologists; p. 257–261.
- Bowring SA, Schmitz MD. 2003. High-precision U-Pb zircon geochronology and the stratigraphic record. Rev Mineral Geochem. 53:305–326. doi: 10.2113/0530305
- Browne GH, Slatt RM. 2002. Outcrop and behind-outcrop characterization of a late Miocene slope fan system, Mt. Messenger Formation, New Zealand. Am Assoc Petrol Geologist Bull. 86:841–862.
- Browne GH, Strachan LJ, King PR, Arnot MJ. 2006. Mass transport complexes from a Late Miocene deep-water succession, Taranaki Basin, New Zealand: scales, styles, and significance in relation to tectonic, eustatic, and autocyclic drivers. AAPG Datapages: search and discovery. Article 50040. [cited 2016 May 18]. Available from: http://www.searchanddiscovery.com/documents/2006/06126browne/images/browne.pdf.
- Coble, MA, Grove M, Calvert AT. 2011. Calibration of Nu-Instruments Noblesse multicollector mass spectrometers for argon isotopic measurements using a newly developed reference gas. Chem Geol. 290:75–87. doi: 10.1016/j.chemgeo.2011.09.003
- Cooper RA, editor. 2004. The New Zealand geological timescale. Institute of Geological & Nuclear Sciences Monograph 22. Lower Hutt: Institute of Geological and Nuclear Sciences.
- Cooper RA, Crampton JS, Raine JI, Gradstein FM, Morgans HEG, Sadler PM, Strong CP, Waghorn D, Wilson GJ. 2001. Quantitative biostratigraphy of the Taranaki Basin, New Zealand: A deterministic and probabilistic approach. Am Assoc Petrol Geologist Bull. 85:1469–1496.
- Crundwell MP. 2004. New Zealand late Miocene biostratigraphy and biochronology—Studies of planktic foraminifers and bolboforms at oceanic Sites 593 and 1123, and selected onland sections [PhD thesis]. Hamilton: University of Waikato.
- Crundwell MP. 2008. Biostratigraphic and paleoenvironmental study of Kanuka-1 offshore well, Taranaki Basin (PEP 38488). GNS Science Consultancy Report 2008/21. Lower Hutt: GNS Science.
- Crundwell MP. 2014. Pliocene to Late Eocene foraminiferal and bolboformid biostratigraphy of IODP Hole 317-U1352C, Canterbury Basin, New Zealand. GNS Science Report 2014/15. Lower Hutt: GNS Science.
- Crundwell MP, Beu AG, Cooper RA, Morgans HEG, Mildenhall DC, Wilson GS. 2004. Miocene (Pareora, Southland and Taranaki Series). In: Cooper RA, editor. The New Zealand geological timescale. Institute of Geological & Nuclear Sciences Monograph 22. Lower Hutt: Institute of Geological & Nuclear Sciences; p. 164–194.
- Crundwell MP, Nelson CS. 2007. A magnetostratigraphically-constrained chronology for late Miocene bolboformids and planktic foraminifers in the temperate Southwest Pacific. Stratigraphy. 4:1–34.
- DeGraaff-Surpless K, Graham SA, Wooden JL, McWilliams MO. 2002. Detrital zircon provenance analysis of the Great Valley Group, California: Evolution of an arc-forearc system. Geol Soc Am Bull. 114:1564–1580. doi: 10.1130/0016-7606(2002)114<1564:DZPAOT>2.0.CO;2
- Ettema P, Mills K, Buchan R, Mathews E. 2000. Ngatoro Field – a middle-aged turbidite reservoir. 2000 New Zeal Petrol Conference Proc. 1:210–222.
- Fedo CM, Sircombe KN, Rainbird RG. 2003. Detrital zircon analysis of the sedimentary record. Rev Miner Geochem. 53:277–303. doi: 10.2113/0530277
- Finlay HJ, Marwick J. 1940. The divisions of the Upper Cretaceous and Tertiary in New Zealand. Trans Roy Soc New Zeal. 70:77–135.
- Finlay HJ, Marwick J. 1947. New divisions of the New Zealand Upper Cretaceous and Tertiary. New Zeal J Sci Technol. B28:228–236.
- Fisher RV. 1984. Submarine volcaniclastic rocks. Geol Soc London Special Publication. 16:5–27. doi: 10.1144/GSL.SP.1984.016.01.02
- Fleming CA, editor. 1959. Lexique stratigraphique international. Volume VI, Oceania. Fascicule 4, New Zealand. Paris: Centre National de la Recherche Scientifique.
- Giba M, Walsh JJ, Nicol A, Mouslopoulou V, Seebeck H. 2013. Investigation of the spatio-temporal relationship between normal faulting and arc volcanism on million-year timescales. J Geol Soc London. 170:951–962. doi: 10.1144/jgs2012-121
- Gibson GW. 1963. The Tongaporutuan Stage. [PhD thesis]. Wellington: Victoria University of Wellington.
- Gibson GW. 1967. Foraminifera and stratigraphy of the Tongaporutuan Stage in the Taranaki coastal and six other section. Part I.—Systematics and distribution. Trans Roy Soc New Zeal, Geol. 5:1–70.
- Gradstein FM, Ogg JG, Schmitz M, editors. 2012. The geologic time scale 2012. 2 Volumes. Oxford: Elsevier.
- Griffin AG. 2001. Late Cenozoic subsurface geology of the Taranaki Peninsula region based on analysis of geophysical well logs [Masters thesis]. Hamilton: University of Waikato.
- Hanumantha Rao Y, Subrahmanyam C, Rastogi A, Deka B. 2002. Slope failures along the western continental margin of India: A consequence of gas-hydrate dissociation, rapid sedimentation rate and seismic activity? Geo-Marine Lett. 22:162–169. doi: 10.1007/s00367-002-0107-9
- Hansen RJ. 1996. Stratigraphy, sedimentology and paleomagnetism of a late Miocene succession, eastern Taranaki Basin margin. [Masters thesis]. Hamilton: University of Waikato.
- Hansen RJ, Kamp PJJ. 2006. An integrated biostratigraphy and seismic stratigraphy for the late Neogene continental margin succession in northern Taranaki Basin, New Zealand. New Zeal J Geol Geophys. 49:39–56. doi: 10.1080/00288306.2006.9515146
- Hayward BW. 1990. Use of foraminiferal data in analysis of Taranaki Basin, New Zealand. J Foramin Res. 20:71–83. doi: 10.2113/gsjfr.20.1.71
- Hesse PP. 1994. The record of continental dust from Australia in Tasman Sea sediments. Quaternary Sci Rev. 13:257–272. doi: 10.1016/0277-3791(94)90029-9
- Holden P, Lanc P, Ireland TR, Harrison TM, Foster JJ, Bruce Z. 2009. Mass-spectrometric mining of Hadean zircons by automated SHRIMP multi-collector and single-collector U/Pb zircon age dating: The first 100,000 grains. Int J Mass Spectrom. 286:53–63. doi: 10.1016/j.ijms.2009.06.007
- Hornibrook NdeB, Brazier RC, Strong CP. 1989. Manual of New Zealand Permian to Pleistocene foraminiferal biostratigraphy. New Zeal Geol Surv Paleontol Bull. 56.
- Jobe AR, Lowe DR, Morris WR. 2012. Climbing-ripple successions in turbidite systems: depositional environments, sedimentation rates and accumulation times. Sedimentology. 59:867–898. doi: 10.1111/j.1365-3091.2011.01283.x
- Johnson-Ibach LE. 1982. Relationship between sedimentation rate and total organic carbon content in ancient marine sediments. Am Assoc Petrol Geologist Bull. 66:170–188.
- King PR. 2000. Tectonic reconstructions of New Zealand: 40 Ma to the Present. New Zeal J Geol Geophys. 43:611–638. doi: 10.1080/00288306.2000.9514913
- King PR, Browne GH, Arnot MJ, Slatt RM, Helle K, Strømsøyen I. 2007a. An overview of the Miocene Mount Messenger-Urenui formations, New Zealand: a 2-D, oblique-dip outcrop transect through an entire third-order progradational, deep-water clastic succession. In: Nilsen TH, Shew RD, Steffens GS, Sudlick JRJ, editors. Atlas of deep-water outcrops. AAPG Studies in Geology 56. Tulsa, OK: American Association of Petroleum Geologists; p. 238–240.
- King PR, Browne GH, Arnot MJ, Strømsøyen I. 2007b. Slope feeder channels, Urenui Formation, Wai-iti and Mimi beaches, Taranaki Basin. In: Nilsen TH, Shew RD, Steffens GS, Sudlick JRJ, editors. deep-water outcrops. AAPG Studies in Geology 56. Tulsa, OK: American Association of Petroleum Geologists; p. 262–264.
- King PR, Ilg BR, Arnot M, Browne GH, Strachan LJ, Crundwell M, Helle K. 2011. Outcrop and seismic examples of mass transport deposits from a Late Miocene deep-water succession, Taranaki Basin, New Zealand. In: Shipp C, Weimer P, Posamentier HW, editors. Tulsa, OK: Society for Sedimentary Geology; 311–350.
- King PR, Scott GH, Robinson PH. 1993. Description, correlation and depositional history of Miocene sediments outcropping along the North Taranaki coast. Institute of Geological and Nuclear Sciences Monograph 5. Lower Hutt: Institute of Geological and Nuclear Sciences.
- King PR, Thrasher GP. 1992. Post-Eocene development of the Taranaki Basin, New Zealand: Convergent overprint of a passive margin. In: Watkins JS, Zhiqiang F, McMillen KJ, editors. Geology and geophysics of continental margins. Tulsa, OK: American Association of Petroleum Geologists; p. 93–118.
- King PR, Thrasher GP. 1996. Cretaceous-Cenozoic geology and petroleum systems of the Taranaki Basin, New Zealand. Institute of Geological and Nuclear Sciences Monograph 13. Lower Hutt: Institute of Geological and Nuclear Sciences.
- Ludwig KR. 2001. SQUID, a user's manual. Berkeley Geochronology Center, Special Publication 2.
- Ludwig KR. 2003. Isoplot (3.41d), a geochronological toolkit for Excel. Berkeley Geochronology Center Special Publication 4.
- Maier KL. 2012. Depositional architecture of deep-water slope systems: Examples from the Quaternary Lucia Chica channel system, offshore central California and the upper Miocene Urenui Formation, New Zealand [PhD thesis]. Stanford, CA: Stanford University.
- McDougall I, Harrison TM. 1999. Geochronology and Thermochronology by the 40Ar/39Ar Method. 2nd ed. New York: Oxford University Press.
- Morgans HEG. 2008. Foraminiferal biostratigraphy of the Urenui-Mount Messenger-Urenui interval (1000–1800 m). Stratford-1 well, onshore Taranaki. GNS Science Consultancy Report 2008/79, April 2008. Lower Hutt: GNS Science.
- Mortimer N. 2004. New Zealand's geological foundations. Gondwana Res. 7:261–272. doi: 10.1016/S1342-937X(05)70324-5
- Müller PJ, Suess E. 1979. Productivity, sedimentation rate, and sedimentary organic matter in the oceans—I. Organic carbon preservation. Deep-Sea Res. 26A:1347–1362. doi: 10.1016/0198-0149(79)90003-7
- Nodder SD, Nelson CS, Kamp PJJ. 1990. Mass-emplaced siliciclastic-volcaniclastic-carbonte sediments in Middle Miocene shelf-to-slope environments at Waikawau, northern Taranaki, and some implications for Taranaki Basin development. New Zeal J Geol Geophys. 33:599–615. doi: 10.1080/00288306.1990.10421378
- Orpin AR. 2004. Holocene sediment deposition on the Poverty-slope margin by the muddy Waipaoa River, East Coast New Zealand. Mar Geol. 209:69–90. doi: 10.1016/j.margeo.2004.06.001
- Raine JI, Beu AG, Boyes AF, Campbell HJ, Cooper RA, Crampton JS, Crundwell MP, Hollis CJ, Morgans HEG. 2015. Revised calibration of the New Zealand Geological Timescale: NZGT2015/1. GNS Science Report 2012/39. Lower Hutt: GNS Science.
- Rotzien JR, Lowe DR, King PR, Browne GH. 2014. Stratigraphic architecture and evolution of a deep-water slope channel-levee and overbank apron: the Upper Miocene Upper Mount Messenger Formation, Taranaki Basin. Mar Petrol Geol. 52:22–41. doi: 10.1016/j.marpetgeo.2014.01.006
- Schaltegger U, Schmitt AK, Horstwood MSA. 2015. U–Th–Pb zircon geochronology by ID-TIMS, SIMS, and laser ablation ICP-MS: Recipes, interpretations, and opportunities. Chem Geol. 402:89–110. doi: 10.1016/j.chemgeo.2015.02.028
- Scott GH. 1992. Planktonic foraminiferal biostratigraphy (Altonian-Tongaporutuan Stages, Miocene) at DSDP Site 593, Challenger Plateau, Tasman Sea. New Zeal J Geol Geophys. 35:501–513. doi: 10.1080/00288306.1992.9514544
- Scott GH. 1995. Coiling excursions in Globorotalia miotumida; high resolution bioevents at the Middle-Upper Miocene boundary in southern temperate water masses? J Foramin Res. 25:299–308. doi: 10.2113/gsjfr.25.4.299
- Scott GH, King PR, Crundwell MP. 2004. Recognition and interpretation of depositional units in a late Neogene progradational shelf margin complex, Taranaki Basin, New Zealand: foraminiferal data compared with seismic facies and wireline logs. Sediment Geol. 164:55–74. doi: 10.1016/j.sedgeo.2003.09.002
- Shane P, Sikes EL, Guilderson TP. 2006. Tephra beds in deep-sea cores off northern New Zealand: implications for the history of Taupo Volcanic Zone, Mayor Island and White Island volcanoes. J Volcanol Geoth Res. 154:276–290. doi: 10.1016/j.jvolgeores.2006.03.021
- Sharman GR, Graham SA, Masalimova LU, Shumaker LE, King PR. 2015. Spatial patterns of deformation and paleoslope estimation within the marginal and central portions of a basin-floor mass-transport deposit, Taranaki Basin, New Zealand. Geosphere. 11:266–306. doi: 10.1130/GES01126.1
- Shaw AB. 1964. Time in stratigraphy. New York: McGraw-Hill Book Company. 365 p.
- Sheridan MF. 1979. Emplacement of pyroclastic flows: a review. Geol Soc Am Special Paper. 180:125–136. doi: 10.1130/SPE180-p125
- Sparks RSJ. 1976. Grain size variations in ignimbrites and implications for the transport of pyroclastic flows. Sedimentology. 23:147–188. doi: 10.1111/j.1365-3091.1976.tb00045.x
- Stacey J, Kramers JD. 1975. Approximation of terrestrial lead isotope evolution by a two-stage model. Earth Planet Sci Lett. 26:207–221. doi: 10.1016/0012-821X(75)90088-6
- Stagpoole V, Funnell R. 2001. Arc magmatism and hydrocarbon generation in the northern Taranaki Basin, New Zealand. Petrol Geosci. 7:255–267. doi: 10.1144/petgeo.7.3.255
- Townsend D, Vonk A, Kamp PJJ. 2008. Geology of the Taranaki area: scale 1:250,000. Institute of Geological & Nuclear Sciences 1:250,000 Geological Map 7. Lower Hutt: GNS Science.
- Utley JP. 1987. The middle to late Miocene Mohakatino Group in the coastal Herangi region, northern Taranaki, New Zealand: Punctuated sedimentation in continental slope-slope basin environments [Master's thesis]. Hamilton: University of Waikato.
- Vonk AJ, Kamp PJJ, Hendy AJW. 2002. Outcrop to subcrop correlations of late Miocene-Pliocene strata, eastern Taranaki Peninsula. In: New Zealand Petroleum Conference Proceedings; 2002 February 24–27; Auckland, New Zealand. Wellington: Ministry of Economic Development; p. 234–255.
- Wright JV, Smith AL. 1980. A working terminology of pyroclastic deposits. J Volcanol Geoth Res. 8:315–336. doi: 10.1016/0377-0273(80)90111-0