ABSTRACT
The composition of 20 modern sand samples (18 streams, one beach, and one lakeshore) collected from the northern South Island provide a dataset for assessing detrital signatures of Paleozoic-Mesozoic basement terranes that may have served as sediment sources for reservoir sandstones in the Taranaki Basin. Non-plutonic terranes produce lithic-rich sand dominated by metamorphic fragments. Samples derived from erosion of the Rakaia and Caples terranes are more quartz-rich than the other non-plutonic terrane samples. Sand from the Dun Mountain-Maitai and Brook Street terranes has the lowest quartz content and is further distinguished by detrital serpentine. Pervasive pumpellyite-bearing metamorphic lithic fragments characterise the Caples, Dun Mountain-Maitai and Brook Street terranes. Feldspar is rare in Eastern province terrane sand. Sand from the Median Batholith tends to have less quartz than sand from the Karamea Batholith and a higher percentage of plagioclase, while sand from the Karamea Batholith has a higher proportion of potassium feldspar. Petrographic analyses were compared with published reports describing the composition of offshore sandstone reservoirs to evaluate their likely provenance.
Introduction
Sandstone reservoir quality is one of the primary factors affecting prospectivity in New Zealand petroleum provinces (Martin et al. Citation1994; Higgs et al. Citation2007; Higgs et al. Citation2010; Higgs and King Citation2018; Rotzien et al. Citation2018). Variables that determine reservoir quality are diverse and include primary depositional factors, such as grain size, sorting, and composition of the sand, as well as secondary or diagenetic factors associated with sand composition, such as development of secondary porosity by grain dissolution, porosity reduction by quartz overgrowth or clay mineral cementation, retardation of quartz cementation by grain coats and grain rims, or compaction of labile constituents such as micas and clay-rich lithic fragments (e.g. Bjørlykke Citation1984; Boggs and Adams Citation1992; Boggs et al. Citation1992; Smale et al. Citation1999; Higgs et al. Citation2007, Citation2010). These primary and secondary factors have been previously described from Cretaceous to Pliocene sandstone reservoirs in the Taranaki Basin (e.g. Higgs et al. Citation2010; Higgs and King Citation2018), New Zealand’s only producing petroleum basin (). The most economically significant are Paleocene-Eocene fluvial to shoreface units (Higgs et al. Citation2007, Citation2012, Citation2017; Higgs and King Citation2018).
Figure 1. Map shows the basement geology of New Zealand, modified from Higgs and King (Citation2018), who in turn modified it from Rattenbury et al. (Citation1998), using data from Turnbull and Allibone (Citation2003) and Mortimer (Citation2004). Our knowledge of the distribution of basement terranes within the offshore Taranaki Basin is aided by numerous petroleum well penetrations into basement rocks and these distributions have been described by Tulloch (Citation1983) and Mortimer et al. (Citation1997) and subsequent revisions (A. Tulloch pers. comm. 2017).
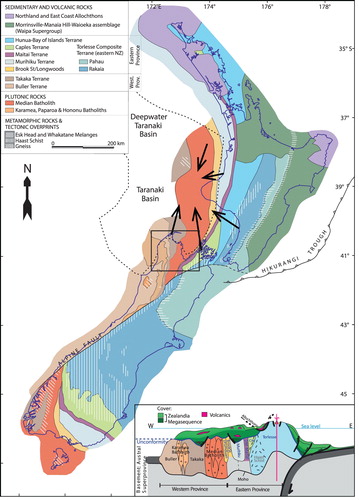
We add another dimension to the assessment of reservoir quality through an investigation of modern sand eroded from known basement lithologies (). This approach provides new insight to the determination of reservoir quality with respect to composition. Firstly, it adds increased certainty regarding the source of sand mineralogy and lithic content, and secondly, shows how compositional variation is influenced by grain size and grain transport. The Taranaki Basin is ideal for such a study because it has a diverse range of potential sediment source area rock types, from plutonic to metasedimentary and metamorphic.
Figure 2. Map shows basement terranes that crop out today in the Nelson area of the northern South Island of New Zealand and locations of all sand sample sites collected in the Nelson area for this study. Figure adapted from Rattenbury et al. (Citation1998).
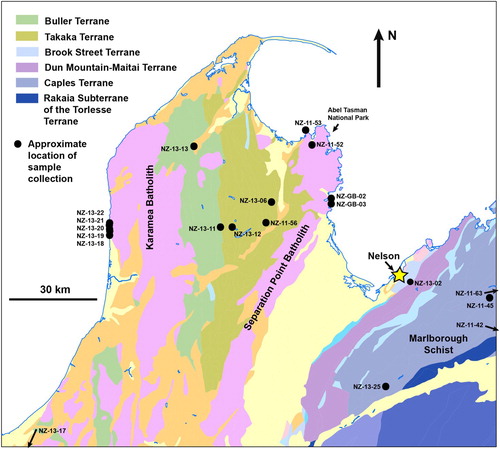
When we apply these results to ancient sandstone reservoirs in Taranaki Basin, taking into consideration paleogeographic reconstructions of the area through the Cretaceous (e.g. Strogen Citation2011), we assume that the terranes that crop out in modern catchments are similar to those that cropped out in ancient drainage systems that were feeding sand into the Taranaki Basin. This, in turn, implies that the detrital modes of modern river sediments should be comparable to ancient equivalents. We attempt to isolate the fingerprints of individual terranes, as their proportion in ancient catchments may have varied. An added dimension is that sand grains may have been recycled, sometimes on multiple occasions, from older formations into younger equivalents, with attendant changes in reservoir sand mineralogy (e.g. Smale Citation1990; Higgs et al. Citation2007; Higgs and King Citation2018). Few studies have examined the mineralogy and provenance of modern stream sediments in New Zealand, but they provide considerable insight to interpreting ancient equivalents (e.g. Smale Citation1991a, Citation1993; Mortimer and Wopereis Citation1997; James et al. Citation2007).
Geological setting and basement geology
The geology of the northern South Island is varied, with basement rocks comprising metasedimentary, volcanic, and plutonic rocks that extend north, from outcrops in the northern South Island, into Taranaki Basin (; Mortimer et al. Citation1997). Basement rocks of the area are divided into Western and Eastern provinces of Cambrian to Jurassic age, separated by the Carboniferous-Cretaceous Median Batholith ( and ; Roser et al. Citation1996; Mortimer et al. Citation1997; Rattenbury et al. Citation1998; Mortimer et al. Citation1999; Cox and Sutherland Citation2007; Mortimer et al. Citation2012). Detailed petrographic descriptions of these rocks are contained in a number of publications (Laird and Shelley Citation1974; Tulloch Citation1988; Cooper and Tulloch Citation1992; Pound Citation1993; Roser et al. Citation1996; Mortimer et al. Citation1999; Münker and Cooper Citation1999; Jongens Citation2006; Tulloch et al. Citation2009) and are briefly summarised below, emphasising specific aspects of basement mineralogy and texture as they pertain to derived sediment composition.
In the northwest Nelson area (), Western Province terranes include the Paleozoic Buller and Takaka terranes. The regionally metamorphosed Buller Terrane comprises mostly Middle to Late Ordovician metagreywacke turbidite successions (Laird and Shelley Citation1974; Roser et al. Citation1996; Rattenbury et al. Citation1998; Mortimer Citation2009; Mortimer et al. Citation2013), while the Takaka Terrane comprises a more varied suite of Middle Cambrian to Early Devonian arc-related ultramafic, volcanic, sedimentary, metasedimentary, and metamorphic rocks (Pound Citation1993; Rattenbury et al. Citation1998; Münker and Cooper Citation1999; Jongens Citation2006).
The Eastern Province comprises Late Paleozoic (Permian) to Cretaceous non-marine and marine metasedimentary to sedimentary rocks that crop out east of Nelson City and to the east and south. Greywacke sandstone dominates over basic igneous rock types in the Eastern Province, with less voluminous conglomerate, limestone, and chert (MacKinnon Citation1983). Eastern Province rocks were broadly deposited or erupted within an arc-trench system between the Permian to Jurassic, and include, from west to east, the Brook Street, Murihiku, Dun Mountain-Maitai, and Caples terranes (e.g. MacKinnon Citation1983; Rattenbury et al. Citation1998; Tulloch et al. Citation1999; Briggs et al. Citation2004). The easternmost terrane is the Torlesse Composite Terrane of Carboniferous to earliest Cretaceous age, which is further subdivided into the Rakaia (older Torlesse) and Pahau (younger Torlesse) subterranes (Begg and Johnston Citation2000; Mortimer Citation2004). Much of the Torlesse Composite Terrane comprises thick successions of greywacke (lithic-rich) sandstone and argillite and their metamorphosed chlorite-schist equivalents, known in the northern South Island as the Marlborough Schist (Smale Citation1990; Kimbrough et al. Citation1992; Mortimer Citation1993; Mortimer and Little Citation1998; Rattenbury et al. Citation1998; Skinner and Brathwaite Citation2002).
These terranes have been intruded by the Karamea and Median batholiths. The Median Batholith, so named because it conveniently separates Western from Eastern Province rocks, is a varied suite of Late Devonian-Carboniferous to Cretaceous intrusive granitic rocks that include the Riwaka Igneous Complex (RIC) and the Late Cretaceous Separation Point Suite (SP; Johnston Citation1974; Smale Citation1990; Muir et al. Citation1995; Muir et al. Citation1996; Rattenbury et al. Citation1998; Mortimer et al. Citation1999). Because the Darran Complex plutonic rocks, which are part of the Median Batholith in Fiordland and offshore Taranaki Basin (Jurassic), have limited outcrop in the northern South Island study area, they were not included in the stream surveys undertaken in this study. Outcrops of the Jurassic Rotoroa Complex (Median Batholith) that occur further south were not sampled in our study. In this regard, our data for the Median Batholith is thus biased towards the Separation Point Suite. The Karamea Batholith (KB) and associated Paparoa Batholith (Devonian-Carboniferous) are represented in outcrop on the western side of South Island (Bishop et al. Citation1985; Tulloch et al. Citation2009).
The basement geology of the northern South Island and of the offshore Taranaki Basin contrasts with the much more uniform, greywacke-dominated, basement geology exposed in the North Island (e.g. Kear Citation1971), which includes the Murihiku Supergroup, Waipapa and Torlesse Composite terranes (and other less volumetrically significant terranes: Begg and Johnston Citation2000; Mortimer Citation2004; Edbrooke et al. Citation2015). All these basement rocks represent terranes that were progressively accreted onto eastern Gondwana during Paleozoic and Mesozoic time (Bishop et al. Citation1985; Begg and Johnston Citation2000; Tulloch et al. Citation2019).
Late Cretaceous and Cenozoic landscape evolution has included exhumation of basement rock in the western South Island, exhumation of a Mesozoic forearc basin and accretionary wedge in eastern South Island, 480 km of dextral displacement of the Western and Eastern provinces along the Alpine Fault since the Early Miocene, plate boundary rotation between the Australian and Pacific plates, and Neogene shearing and clockwise rotation of the Marlborough region of northeastern South Island. With increasingly oblique convergence of the Pacific and Australian plates over time, deformation and uplift has occurred throughout the northern South Island and southern North Island during the Neogene (e.g. Walcott Citation1998; Sutherland Citation1999; Barnes Citation2009; Randall et al. Citation2011), with deposition of this eroded sediment into basins such as Taranaki in the Neogene.
Previous sand provenance work in New Zealand
David Smale worked extensively on the use of heavy minerals to better understand the provenance of Cenozoic and Mesozoic sandstones across New Zealand sedimentary basins, linking them to potential basement sources by studying basement mineralogy as well (Smale Citation1988, Citation1990, Citation1991b, Citation1992, Citation1997, Citation2007; Hayward and Smale Citation1992). Other workers have focused on light mineralogy of selected sandstone units (e.g. Martin et al. Citation1994; Mortimer Citation1994; Higgs et al. Citation2010; Higgs and King Citation2018). In contrast, few workers have looked specifically at the provenance signatures of New Zealand stratigraphic units and basement terranes by studying sediments from modern fluvial systems draining those units. Exceptions are the work on the Wanganui River Smale (Citation1991a), Bay of Plenty (Smale Citation1993), on the Waipaoa river system by James et al. (Citation2007), and on various South Island rivers by Mortimer and Wopereis (Citation1997), Shapiro et al. (Citation2007) and Bender-Whitaker et al. (Citation2018). The Waipaoa and South Island river system studies were aimed at understanding the light mineralogy and provenance of sand deposited in offshore shelf and deep-marine sedimentary systems as discussed in Bender-Whitaker et al. (Citation2018), Parra et al. (Citation2012) and Marsaglia et al. (Citation2010, Citation2011). Some of the key findings from these works are the detrital signatures of sediment derived from recycling of Cenozoic and Cretaceous clastic rocks of the North Island, metasedimentary units (Torlesse to Haast Schist) of the South Island, and application in source-to-sink models. Our study builds on these results and represents the first work focused on determining the detrital fingerprints of a diverse range of New Zealand basement units by examining the characteristics of sand from streams draining them.
Methodology
Twenty-nine sand samples were collected, one to four per geologic unit, and petrographically reviewed in this study, and 20 of those samples were point counted to determine their detrital modes. These were collected during March 2013 from rivers and streams draining plutonic, metamorphic, and sedimentary terranes, augmented by sand samples collected between 2006 and 2012 from northern South Island streams. A few of the collected samples are from beaches (marine and lacustrine) with sand derived from these terranes. Samples are located in , with location information and descriptions in . In general, sites selected were along larger streams and rivers (flowing at >10 m3/sec), but for some samples, smaller streams were sampled. One of the caveats of the sampling strategy was to identify unique detrital ‘fingerprints’ by collecting from sites that were associated with a specific terrane, and for this reason, we attempted to only sample from streams draining one basement terrane, each of which are characterised by one or more rock types. Although this was achieved in most situations, it was difficult to find a catchment that exclusively drained either the Dun Mountain-Maitai Terrane or the Brook Street Terrane, and these basement rock types are grouped together in our results. Murihiku rocks crop out in a narrow belt in the Nelson region and therefore, this terrane is not represented by samples in our study. Local geology was determined using the 1:250,000 scale geologic map for the Nelson area (Rattenbury et al. Citation1998). Wherever possible, obvious signs of human disturbance in the stream were avoided. Further details can be found in Doran (Citation2014).
Table 1. Sample locations and terrane descriptions.
Individual sand samples weighed between 250 and 300 g (dry weight). Samples were air-dried, sieved into five sand fractions from very fine- to very coarse-grained, made into thin sections, and stained for recognition of potassium- and calcium-bearing feldspar after Marsaglia and Tazaki (Citation1992). Thin sections for each grain size were prepared and point counted, using a microscope equipped with an automated point-counting stepper stage. In all, 61 thin sections were point counted. Four hundred grains per thin section were point counted using the Gazzi-Dickinson method (after Ingersoll et al. Citation1984) to determine compositional modes estimating mineral and lithic populations. Using this method, mineral grains >62.5 microns (4ø) were counted in mineral categories, including mineral grains and sand-sized components in lithic fragments. More than 100 monomineralic grain and lithic fragment categories were initially recognised and then simplified to 73 categories, as summarised in , where recalculated parameters are defined, and Supplementary Tables 3 and 4, where data and recalculated parameters are presented (https://doi.org/10.1080/00288306.2019.1618881).
Table 2. Definitions of counted categories and recalculated parameters.
A modified version of the lithic classification scheme developed by Garzanti and Vezzoli (Citation2003) was used, whereby metamorphic lithic fragment classification is based on the degree of recrystallisation and foliation. By this method, clay-rich metasedimentary grains showing incipient alignment of clays without complete foliation and segregation of mica planes are classified as metapelites (Lmp1) or slate lithic fragments. Silt-rich metasedimentary lithics are classified as metasiltstones (Lmf1) if micas are starting to surround and segregate silt-sized grains without achieving strong cleavage. This study classifies all micaceous, polycrystalline grains simply as polycrystalline mica (Lmm), and adds an intermediate rank of 1.5 (Lmp1.5, Lmf1.5, or Lmv1.5) for clasts containing aggregates of pumpellyite – cloudy, semi-opaque, microcrystalline or cryptocrystalline aggregates as described by Smale (Citation1988) –replacing detrital grains (e.g. plagioclase) and/or associated matrix, as reported in the literature (Turnbull Citation1979; Skinner and Brathwaite Citation2002). Finally, lithic serpentine fragments in sand associated with the Brook Street and Dun Mountain-Maitai volcanic terranes are classified by their texture, deformed vs. undeformed (see details in Doran Citation2014).
As the field-based classification scheme of Turnbull et al. (Citation2001) is commonly used to classify New Zealand metamorphic rocks, we review it here and relate it to the Garzanti and Vezzoli (Citation2003) scheme used in this study. Turnbull et al. (Citation2001) discussed field-based classification schemes of schist in New Zealand based on textural and mineralogical criteria and then proposed their own classification that includes mineral grain size. They related their field-based criteria for sedimentary protolith and metamorphic textural zones (I, IIA, IIB, III, IV) to petrographic attributes. Turnbull et al.’s (Citation2001) zones in thin section are shown by progressive pressure-solution, textural changes and neometamorphic mineral growth as foliation develops. A key indicator is the thickness/length of white mica, with a similar progressive increase in mica size in schists with mudstone and sandstone protoliths. We based our sand grain classification scheme on that of Garzanti and Vezzoli (Citation2003); it is similar to Turnbull et al.’s (Citation2001) rock classification (Zones I-IV) but more detailed and covers a broader range of metamorphic protoliths (e.g. Ls-Lm varieties). The following is our attempt to correlate between the two schemes: Textural Zone I (Lsp, Garzanti and Vezzoli) – detrital texture with no preferred orientation of metamorphic mica; Textural Zone IIA (Lmf1, Garzanti and Vezzoli) – weakly oriented metamorphic mica (foliated greywacke, semi-schist, slate); Textural Zone IIB (Lmf2, Garzanti and Vezzoli) – strong orientation of metamorphic mica, quartz subgrains (foliated greywacke, semi-schist, slate, phyllite); Textural Zone III (Lmf3, Garzanti and Vezzoli) – nondetrital equigranular mica and quartz, with the latter in sub-mm segregations; and Textural Zone IV (Lmf4, Rmf5, sand-sized mineral grains including mica, Garzanti and Vezzoli) – segregated quartz and feldspar. The petrographic images for grain categories in Garzanti and Vezzoli (Citation2003) do show progressive increase in mica width and length with increasing grade of metamorphism. Note that the recognition of foliation in sand grains is a function of orientation, which is not uniform in the loose sand samples petrographically analysed in this study. Therefore we added another category (Lma) to cover aggregates of quartz, feldspar and mica without foliation that we also relate to high-grade metamorphic or igneous intrusive sources.
Recalculated parameters were determined for each counted sample; the resulting parameter data set was analysed to determine trends in grain size, trends among samples from the same terrane, and trends among the various terranes. Results were plotted on ternary diagrams for analysis after Dickinson (Citation1985).
Results
Point-count results and recalculated parameters for sand samples presented in Figures 5 to 8 and Supplementary Tables 3 and 4 (https://doi.org/10.1080/00288306.2019.1618881) are summarised below according to each of the major basement terranes from which they were sourced. Images of selected grain types found in the sand samples are presented in and .
Figure 3. Sand photomicrographs under plane-polarised light on left, with polars crossed on right: In A, and B, Karamea Batholith fine-grained plutonic stream sand shows yellow-stained microcline (K) and potassium feldspar (K), quartz (Q), biotite (B), and pink-stained calcium plagioclase (P). In C, and D, Median Batholith fine-grained plutonic stream sand shows red-stained potassium plagioclase, quartz, yellow-stained potassium feldspar, and higher-birefringence epidote. In E, and F, Paparoa Batholith fine-grained plutonic stream sand contains plentiful quartz along with yellow-stained potassium feldspar and red-stained calcium plagioclase. In G. and H., medium-grained stream sand from the Dun Mountain-Maitai and Brook Street terranes shows bastite texture of red-stained serpentine replacing a mafic mineral (Dser bt) next to a grain of epidote (E), Sample number NZ-13-02.
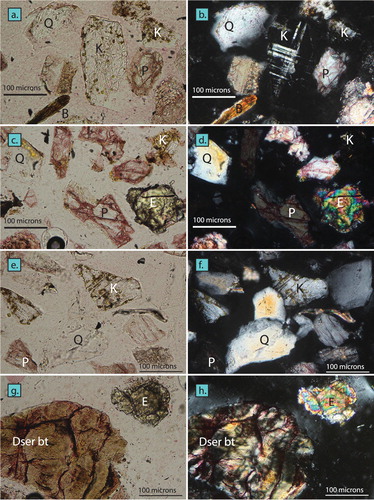
Figure 4. Sand photomicrographs under plane-polarised light on left, with polars crossed on right: In A, and B, a metasiltstone lithic fragment (Lmf1.0; after Garzanti and Vezzoli Citation2003) from the Buller Terrane stream sand (sample NZ-13-13) exhibits rough cleavage, with sand-sized quartz grains in a matrix of micas aligned around larger grains. In C, and D, Takaka Terrane fine-grained stream sand shows metasiltstone (Lmp1.0) and metasiltstone (Lmf1.0) fragments. In E, and F, a slate lithic fragment (Lmp1.0) from the Buller Terrane stream sand shows rough cleavage and a brown matrix. In G, and H, a slate lithic fragment (Lmp1.5) from the Caples Terrane (sample NZ-13-25) has rough cleavage and brownish pumpellyite-aggregate recrystallization of the matrix.
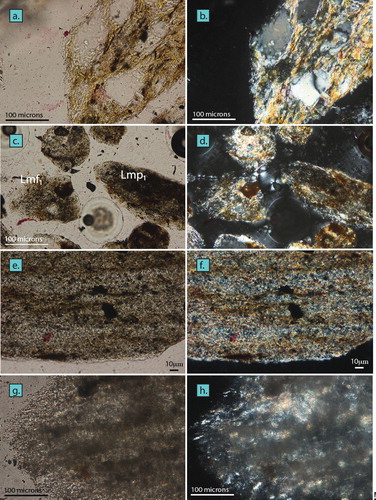
Western Province – Buller Terrane
Two sand samples were point counted from the Buller Terrane in size fractions that included coarse, medium, fine, and very fine sand. Sand derived from catchments of the Buller Terrane has more lithic fragments than quartz or feldspar, with a QFL%L ranging from 65 to 90%. Quartz dominates over feldspar, with a QmKP%Qm of 85 to 100% (). The one exception is a very fine sand fraction from Sample NZ-13-11, in which lithic classification is largely obscured by reddish oxide grain coatings, making it difficult to classify lithic types. Potassium feldspar is rare in all Buller Terrane sand samples, reaching a maximum QmKP%K of 1.4%. Volcanic lithics were not recognised and sedimentary lithics (siltstone, argillite, or dirty chert fragments) are rare (). Metamorphic rock fragments (Lm) are the dominant lithic type and their grade is weighted a bit more toward metasiltstones and metamudstones with poorly developed cleavage than toward quartz-mica tectonites with strong cleavage (). There is no clear trend in metamorphic grade among grain sizes as determined by lithic type proportions.
Figure 5. A, Ternary diagrams show the proportion of Q (total quartz), F (total feldspar), and L (total lithic fragments) for stream sand derived from various terranes, from left: Marlborough Schist, Dun Mountain-Maitai Brook Street, Takaka, and Buller units. B, Ternary diagrams show the proportion of Qm (monocrystalline quartz), K (potassium feldspar), and P (plagioclase – stained and unstained feldspar), for stream sand from the same terranes pictured in A. See and Supplementary Tables 3 and 4 for recalculated parameter definitions and results plotted in the ternary diagrams.
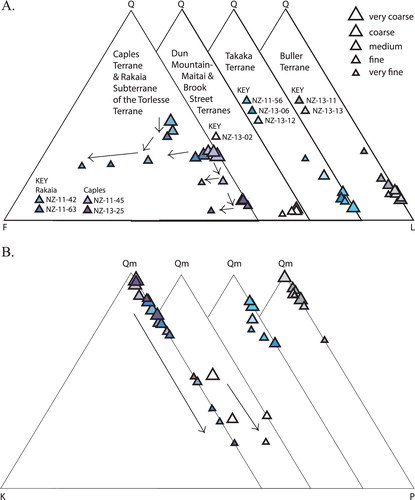
Figure 6. A, Ternary diagram shows the proportion of LmLvLs in stream sand derived from, from left: Dun Mountain-Maitai and Brook Street, Marlborough Schist, Takaka, and Buller units. B, Ternary diagram shows Lmt (total quartz-mica tectonite), Ls (total mudstone/siltsone), Lmp-Lmf (total metamudstone to siltsone) for stream sand from the same terranes pictured in A. See and Supplementary Tables 3 and 4 for recalculated parameter definitions and results plotted in the ternary diagrams.
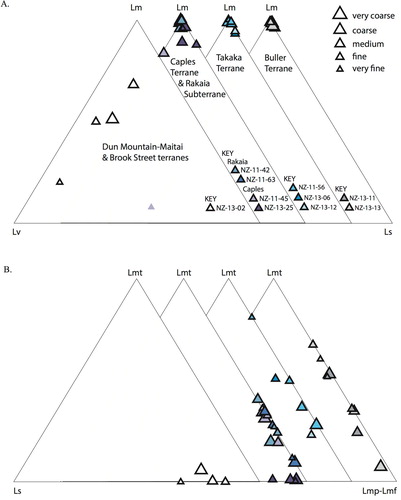
What stands out about sand detritus from the Buller Terrane is that most quartz is polycrystalline, even when individual crystals are large enough to be counted as sand-sized monocrystalline grains. Polycrystalline quartz frequently shares stylolitic intergrowths and grain boundary migration with neighbouring quartz crystals, as sketched by Stipp et al. (Citation2002). An exception is sample NZ-11-13, which contains monocrystalline quartz mostly undeformed and with straight extinction.
Western Province – Takaka Terrane
Three sand samples were point counted from the Takaka Terrane, two from streams and one from a lake. The lake sand sample and one of the stream sand samples were counted in the medium size fraction; one stream sand sample was counted in size fractions from coarse to very fine. Sand samples from streams draining the Takaka Terrane have rare feldspar (QFL%F of 1–7.4%) and more lithic fragments than quartz grains (), with a QFL%L ranging from about 64 to 93%. Monocrystalline quartz is more common than either potassium feldspar or plagioclase (), with QmKP%Qm ranging from 69 to 89%. Lithic fragments are almost entirely metamorphic (); sedimentary lithics make up to ∼6% of lithic fragments. It is noteworthy that the Takaka Terrane is lithologically diverse, with mafic to siliciclastic to carbonate units but the samples representing this terrane show similar QFL, monomineralic and lithic proportions.
Although the Takaka Terrane is described as volcaniclastic in outcrop, alteration and metamorphic overprinting has resulted in recognisable volcanic lithics making up less than 1% of the lithic population in associated sand samples (). Detrital serpentine replaces mafic minerals similar to textures pictured in G–H from the Dun Mountain-Maitai and Brook Street terrane sand, with an occasional, still intact pyroxene. Fragments of metamudstone and metasiltstone make up one-half to three quarters of lithic fragments, with the remainder being quartz-mica tectonites and unmetamorphosed argillites and siltstones (). An exception to this trend is the very fine-sand fraction of Sample NZ-11–56, in which quartz-mica tectonite fragments are more common than metamudstone and metasiltstone fragments. A significant proportion of quartz-mica tectonite, metasiltstone and metamudstone fragments exhibit fibrous aggregates of pumpellyite, which are common in samples that have experienced prehnite-pumpellyite metamorphism (Coombs Citation1960; Smale Citation1988). C–D shows an example of one such grain from the Caples Terrane sand. Metamorphic origin of polycrystalline quartz is indicated by stylolitic contacts exhibiting grain boundary migration.
Eastern Province – Dun Mountain-Maitai and Brook Street terranes
One sand sample from a stream draining both the Dun Mountain-Maitai and Brook Street terranes was point counted in size fractions from coarse to very fine sand. In the sand sample representing the Dun Mountain-Maitai and Brook Street terranes (NZ-13-02), lithic fragments dominate over quartz and feldspar and make up about 90% of the sand (). Overall counts show a trend toward slightly higher proportions of feldspar with decreasing grain size. Potassium feldspar is uncommon, ranging from 0 to about 13%, and plagioclase is more abundant than monocrystalline quartz except in the coarse-sand fraction, in which monocrystalline quartz is more abundant (). Monocrystalline quartz ranges from about 25% in the very fine sand fraction to slightly more than 50% in the coarse sand fraction. In the fine- and very fine-grained sand fractions, calcium plagioclase increases in relative abundance but remains a minor component. This sample has a diverse assemblage of lithic components ().
The main lithic components are subrounded to well-rounded fragments of metasiltstone, metapelite, and metabasite/metavolcanic rocks, often with pumpellyite that make it difficult to differentiate metavolcanic from metasiltstone clasts. Metamorphic and volcanic lithic fragments with microlitic textures are widely variable in proportion (), from subequal in coarse- and fine-grained sand fractions, to higher fractions of metamorphic clasts in the medium sand fraction and higher volcanic clasts in the very fine sand fraction. Sedimentary lithic fragments are uncommon, ranging from 8 to 15% of the total lithic population (LmLvLs%Ls). Metamorphic lithic fragments are almost entirely metamudstone and metasiltstone fragments. Quartz-mica tectonite fragments are nearly absent and only significant in the coarse sand fraction, where they make up 5% of metamorphic lithics (). In the fine- and very fine-grained sand fractions, pumpellyite-bearing metapelite fragments are less common than in the medium sand fractions.
Dense minerals include unaltered clinopyroxene (mostly augite) and orthopyroxene, and represent approximately 2–5% of total points counted (). Other dense minerals include epidote, garnet, opaques, and clinozoisite/zoisite. Accessory minerals include chlorite and serpentine. Serpentine often retains the texture of the olivine and pyroxene it has replaced. In the very fine-sand fraction, serpentine makes up more than a quarter of all points counted; it is least abundant (approximately 12%) in the fine sand fraction.
Eastern Province – Caples Terrane and Rakaia subterrane (Marlborough Schist)
Four sand samples derived from rocks of varying metamorphic grade were selected for detailed analysis from the Marlborough Schist (Caples Terrane and Rakaia subterrane of the Torlesse Terrane). Two sand samples were point counted from each of these terranes in size fractions that ranged from coarse to very fine; one of the samples, NZ-11-45, also produced very coarse sand, which was point counted. Sand samples from the Marlborough region typically came from streams carrying sediment from more than one metamorphic grade of rock according to geologic maps of their catchments. The samples are from regions with increasing order of metamorphic grade based on degree of foliation, as ranked in the field by Mortimer (Citation1993) and shown in Rattenbury et al. (Citation1998): NZ-13-25 (Caples; TZ IIA and IIB), NZ-11-45 (Caples and Rakaia; TZ III and IV), NZ-11-63 (Caples and Rakaia; TZ III and IV), and NZ-11-42 (Rakaia). Evaluation of Sample NZ-11-45 may have been complicated by Pleistocene drainage reversal (Mortimer and Wopereis Citation1997) as discussed below.
Lithics dominate over quartz and feldspar in the Caples Terrane samples. The proportion of lithic fragments (QFL%L) varies from 64 to 92%, with quartz making up most of the rest of the samples in the medium sand and coarser sand fractions. Overall quartz abundance, both as a percentage of QFL and as a percentage of QmKP (), is higher in samples collected farther east (NZ-11-45, NZ-11-63, and NZ-11-42). In Caples Terrane sample NZ-13-25 (E–F), the main lithic components are subrounded to well-rounded, pumpellyite-bearing metamudstone and metasiltstone, followed by metamudstone and metasiltstone fragments without pumpellyite (). Relict microlitic textures in a brownish to black groundmass help define metavolcanic clasts. In Sample NZ-11-45, from the Caples Terrane at the boundary with the Rakaia subterrane, the main components are pumpellyite-bearing metamudstone lithic fragments, followed by monocrystalline quartz, quartz-biotite tectonite fragments, metasiltstone fragments with and without pumpellyite, and metamudstone fragments without pumpellyite. Sodium-rich plagioclase is more abundant than calcium plagioclase. In addition to quartz-biotite tectonite fragments, this sample has quartz-chlorite tectonite fragments. Dense minerals include epidote and clinozoisite/zoisite, especially in finer-grained sand fractions, with rare sphene and garnet.
In the very fine-sand fraction, feldspar, which is rare in the coarser size fractions, increases to QFL%F of 14–16% (). A similar trend is apparent in QmKP proportions, with quartz dominating over plagioclase in the coarser sand fractions and plagioclase nearly equal to or dominating over quartz in the very fine-sand fraction, with a QmKP% plagioclase of nearly 80% in sample NZ-13-25 and no potassium feldspar. Metamorphic lithic fragments are more abundant than volcanic lithic fragments or sedimentary lithic fragments (), and these tend to be lower grade metamudstones and metasiltstones.
In the two sand samples from the Rakaia subterrane, NZ-11-63 and NZ-11-42, lithic fragments dominate over quartz and feldspar in most grain fractions (). Feldspar dominates over quartz and lithic fragments in the very fine-grained sand fractions. The subangular to well-rounded feldspar is almost entirely albite. Monocrystalline quartz is most abundant in the medium- to coarse-sand fractions (). Metamorphic lithic fragments, mostly metamudstone and metasiltstone, are more abundant than volcanic lithic fragments or sedimentary lithic fragments (). Both samples contain some foliated metasiltstone lithics with no pumpellyite. Other common lithic components are various metamorphic aggregates of quartz, plagioclase, mica, and dense minerals with no clear evidence of foliation, along with foliated metasiltsones with no pumpellyite and sodic (unstained) plagioclase. In sample NZ-11-63, some plagioclase has myrmekitic intergrowths. Lithic clasts include highly schistose, quartz-biotite tectonite fragments and metavolcanic grains with microlites or laths recrystallised to mica.
In all the Marlborough Schist-derived sand samples examined in this study, biotite increases in abundance in fine- and very fine-sand fractions and is more likely to be altered than unaltered (). Some of the counted biotite could instead be stilpnomelane, a mineral similar in appearance to biotite, that has been reported as a minor constituent in Otago and Marlborough schist rocks (Brown Citation1971; Turnbull Citation1979; Craw Citation1984; Skinner and Brathwaite Citation2002), where biotite is rare (Mortimer and Little Citation1998). Muscovite and chlorite are minor stand-alone accessory minerals. The dense minerals clinozoisite/zoisite and to a lesser extent epidote are more common in the two Rakaia subterrane sand samples and are most abundant in the very fine-sand fraction. Opaques, sphene, garnet, and corundum are uncommon or rare ().
Other differences are related to the percentages of quartz and types of lithic fragments. Of the two Caples Terrane samples, Sample NZ-11-45 exhibits higher proportions of monocrystalline quartz, quartz-biotite tectonite fragments, pumpellyite-bearing metasiltstones in the finer sand fractions, and quartz-chlorite tectonite fragments, along with a decrease in the proportion of pumpellyite-bearing metamudstone fragments. This is consistent with the observations of Turnbull (Citation1980) who identified pumpellyite-actinolite and prehnite-pumpellyite metamorphic facies in the Caples terrane in northern Southland, New Zealand. Sample NZ-13-25 provides the clearest example of fragments of low-grade, prehnite-pumpellyite-facies metamorphic rocks. It has the most pumpellyite, the largest number of unmetamorphosed argillite and siltstone fragments, the lowest number of quartz-mica tectonite fragments, and almost no feldspar except for highly altered plagioclase grains in the very fine-sand fraction (Supplementary Tables 3 and 4, https://doi.org/10.1080/00288306.2019.1618881). Many individual clasts in metasiltstone fragments appear to have been replaced by pumpellyite and sericite. On occasion, the colourful birefringence of epidote is visible through the pumpellyite. Relict microlitic textures define some metavolcanic clasts.
We should point out that although the sample from the Kaituna River (NZ-11-45) was taken where the catchment consists of higher grade (TZIII and IV) schist, the north-flowing drainage in this valley only developed in the Pleistocene and the valley fill contains gravel derived from lower grade (TZI, IIA, IIA) Caples and Dun Mountain Ophiolite Belt rocks fed by a formerly south-flowing river (Mortimer and Wopereis Citation1997). Nevertheless, Sample NZ-11-45 is compositionally similar to the other higher-grade samples, with only a hint of potential Dun Mountain Ophiolite Belt contribution being a volcanic component in one size fraction. We also can’t rule out anthropogenic effects such as widespread deforestation in this region (visible on Google Earth), which might have enhanced local sediment contribution to the river rather than cannibalisation of valley fill.
Karamea Batholith (KB) and Paparoa Batholith
Five sand samples from streams draining the Karamea Batholith and one from a stream draining the Paparoa Batholith were point counted. Two of the Karamea samples and the Paparoa sample were point counted in size fractions from coarse to very fine; three of the Karamea samples were point counted in only the medium sand fraction. Compositional modes from the six sand samples derived from KB and Paparoa streams are shown in and Supplementary Tables 3 and 4 (https://doi.org/10.1080/00288306.2019.1618881). Overall, stream sand is composed mainly of quartz and feldspar, with the percentage of lithic grains (QFL%L) <14%. Proportions of monocrystalline quartz, potassium feldspar, and plagioclase show considerable variation (). Medium-sand fractions collected from two different parts of KB – a set of northerly samples (NZ-13-21 and NZ-13-22) and southerly samples (NZ-13-18, NZ-13-19, and NZ-13-20) – cluster together in different areas of the QFL and QmKP diagrams (). The more northerly samples are more feldspathic and on QmKP plots have less monocrystalline quartz and nearly subequal amounts of potassium feldspar and plagioclase. The more southerly samples are more quartzose overall and on a QmKP plot have more monocrystalline quartz, more potassium feldspar, and less plagioclase. In contrast, the Paparoa Batholith sand sample farther south (NZ-13-17) is compositionally distinct, with lower potassium feldspar and higher monocrystalline quartz ().
Figure 7. A, Ternary diagrams show the proportion of Q (total quartz), F (total feldspar), and L (total lithic fragments) for sand derived from the Karamea Batholith (left) and the Median Batholith (right). B, Ternary diagrams show the proportion of Qm (monocrystalline quartz), K (potassium feldspar), and P (plagioclase – stained and unstained feldspar), for the same batholith-derived stream sand pictured in A. See and Supplementary Tables 3 and 4 for results plotted in the ternary diagrams.
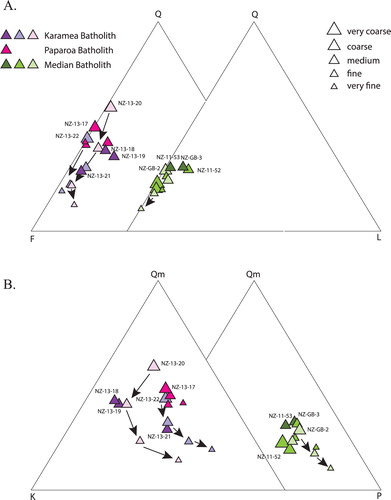
The KB-derived samples () contain the highest percentage of biotite of all the samples examined in this study. It is usually brown to greenish-brown, well rounded, and locally altered. Biotite in the Paparoa Batholith sand sample is sometimes a deep, clear green colour. Muscovite is uncommon. Dense minerals are rare (, from <1% to <6% of total grains) and are most often found in the very fine-grained sand fraction; they include zircon, sphene, apatite, garnet, corundum, clinozoisite, epidote, and opaques. Other workers have reported these in KB rocks along with magnetite, iron oxide, epidote, monazite, andalusite, sillimanite, staurolite, and cordierite (Smale Citation1990; Muir et al. Citation1996; Rattenbury et al. Citation1998).
Figure 8. Charts show relative abundances of micas (mostly biotite with minor muscovite and chlorite) and dense minerals as a percentage of total sand grains, by size fraction, counted from streams draining each terrane and batholith.
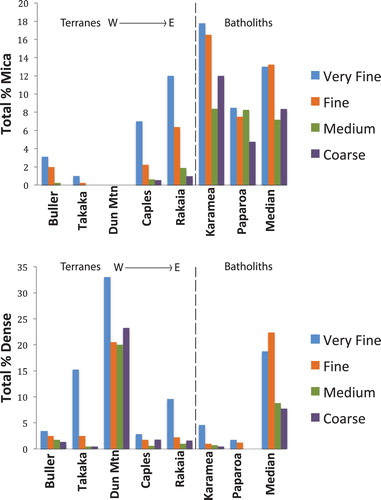
Three representative samples (one northern, one southern, and the Paparoa) were selected for a more complete size fraction analysis. In the two KB sand samples (NZ-13-20, NZ-13-21), coarser sand fractions show a general trend toward higher percentages of quartz, and fine- and very fine-grained sand fractions have higher proportions of feldspar on QFL plots (). The one consistent trend among all three samples is that the QmKP proportion of plagioclase increases in the very fine sand fraction (). Sample NZ-13-20 shows an unusual trend from monocrystalline quartz dominance in the coarse-sand fraction to potassium feldspar dominance in the medium-sand and fine sand fractions, and finally to plagioclase dominance in the very fine-grained sand fraction. Sample NZ-13-22 shows a similar, less pronounced trend, but with overall less potassium feldspar.
The Paparoa (NZ-13-17) sand size fractions cluster more tightly than the other KB sand samples in both QFL and QmKP diagrams (). In smaller grain sizes from the KB, biotite becomes more common, though in the Paparoa sand sample, biotite remains fairly constant ().
Separation Point Suite (SP), Median Batholith
Four sand samples were point counted from three streams and one beach draining the Separation Point Batholith – two in the medium sand fraction only, one in size fractions from very coarse to very fine, and one in size fractions from coarse to very fine. Separation Point-derived sand is richer in feldspars combined than either quartz or lithic clasts (). Most sand samples, particularly the medium sand, plot together fairly tightly and contain QFL% feldspar of 62–72%, and QFL% lithics of <10% (). In QmKP diagrams, samples cluster for the most part near the plagioclase end member (primarily calcium plagioclase), with QmKP% plagioclase of 49–65% for all sand fractions. Well-developed myrmekite fragments are fairly common. Three of the four medium-grained sand samples cluster fairly tightly on a QmKP diagram and contain around QmKP% monocrystalline quartz of about 30% ().
The population of dense minerals is greater in Sample NZ-11-52 (), from the northern exposure of the pluton, than in Sample NZ-GB-02, from the southern exposure. Hornblende in particular is more common in samples from the north and rare to absent in samples from the south.
Biotite grains are tan to dark brown in colour, often altered, and sometimes occur as components with plagioclase or quartz in coarser (Lma) lithic fragments. Dense minerals include sphene, epidote, opaques, pleochroic green to green-brown to blue–green hornblende, zoisite, clinozoisite, and rutile. Allanite and the contact-metamorphic minerals andalusite, sillimanite, and staurolite reported by Smale (Citation1990) were not identified in this study.
One of these (sample NZ-11-52) was chosen to perform a more detailed size fraction analysis along with the most compositionally distinct sample, NZ-GB-02, which has a slightly lower monocrystalline quartz proportion. Grain size-dependent changes include an increase in the proportion of plagioclase in fine- and very fine-grained sand fractions, especially in Sample NZ-GB-02, which also shows a trend of overall increased QFL% feldspar content in the finer grain sizes (). The same sample shows a size-fraction QmKP trend from highest monocrystalline quartz in the coarse-sand fraction to slightly higher potassium feldspar in the medium-sand fraction to plagioclase dominance in the finer-sand fractions ().
Discussion
Our results indicate a clear petrographic difference between sediment derived from the two major basement rock types, plutonic and metasedimentary. There are distinct differences in modal QFL data, as well as proportions of monomineralic (QmKP) and lithic (LmLvLs) constituents (). Subtle differences in composition help differentiate detritus from individual terranes within each group. We discuss below how sand sources may be discriminated.
Takaka and Buller Terrane sand
The Western Province’s Buller and Takaka terranes both produce lithic-rich sand with similar QFL, QmKP, and LmLvLs modal compositions ( and ). The vast majority of the lithics are metamorphic, typically pumpellyite-bearing metamudstone and metasiltstone fragments, and the dominant feldspar is plagioclase. Our limited data show that Buller sand contains more mica, and less dense mineral content than Takaka sand, and the Takaka sand contains a more diverse suite of dense minerals, as recognised by Smale (Citation1990; i.e. mostly opaque minerals and rare garnet and allanite in the Buller versus the Takaka, which contains epidote, hornblende, and detrital serpentine). Polycrystalline quartz is likely a product of metamorphic overprinting and recrystallization of the Buller rock matrix as described by Blatt and Christie (Citation1963), whereas undeformed quartz may be recycled detrital grains from the original quartz-rich sandstone units (A–B). Although the Takaka Terrane contains several carbonate units, we did not recognise detrital carbonate lithic fragments, likely because such units are prone to dissolution in temperate climates and indeed these Takaka units presently form karst landscapes (e.g. Mueller Citation1991). The ages of detrital zircons in the Takaka and Buller terranes overlap (Adams et al. Citation2015; Higgs and King Citation2018), so they would not be good discriminators. In sum, it is difficult to distinguish sand derived from each of these two terranes using our limited data set; however, sand samples derived from these terranes are compositionally distinct from other terranes.
Caples and Rakaia subterrane (Marlborough Schist) sand
Sand samples from streams draining the Eastern Province’s Caples and Rakaia subterranes also produce lithic-rich sand with a compositional range that overlaps that of the Buller and Takaka terranes. These samples represent catchments that typically have more than one metamorphic grade and this may in part account for their widely distributed modal composition in and . Some sand fractions derived from this basement grouping range to higher percentages of QFL%Q and QFL%F () than those of the Takaka and Buller terranes, but there are no distinct trends among Marlborough Schist samples except that the feldspar content is often highest in the very fine-grained fraction. This feldspar is almost entirely plagioclase (), mostly albitic or altered (Supplementary Tables 3 and 4, https://doi.org/10.1080/00288306.2019.1618881). As with the Takaka and Buller terranes, metamorphic lithic fragments dominate, specifically metamudstones and metasiltstones (), but a few samples have traces of volcanic lithic fragments. These lithics may be recycled from volcaniclastic sandstones described by Turnbull et al. (Citation2001) in the Caples Terrane.
These results are in agreement with published reports that describe Rakaia rocks as well foliated, pelitic schist and metagreywacke (Mortimer Citation1993; Rattenbury et al. Citation1998) with metamorphic albite (Coombs Citation1960; Adams and Kelley Citation1998). Caples Terrane outcrops range from weakly metamorphosed sandstone and siltstone to higher-grade schist near the boundary with the Torlesse Terrane (Rattenbury et al. Citation1998). The mineralogy of the Marlborough Schist, the metamorphosed equivalent of the Torlesse Composite Terrane, is similar to the Torlesse as would be expected, and it generates mostly metamorphic rather than volcanic or sedimentary lithic fragments (). Bender-Whitaker et al. (Citation2018) were able to differentiate sand derived from metasedimentary Torlesse, Torlesse-schist transition, and schist lithologies based on the proportions of lower-grade and higher-grade lithic fragments and mica. Overall, Marlborough Schist sand samples had slightly greater amounts of lower-grade metamorphic lithics and mica than either the Buller and Takaka terranes; furthermore, the samples derived from Marlborough Schist were surprisingly similar to sand derived from their protolith (Torlesse) counterparts in terms of these parameters, rather than sand derived from other South Island semi-schist to schist terranes. This difference would suggest a lower degree of metamorphism in the Marlborough Schist than the southern terranes but it may instead be related to the metamorphic mineralogy and textural attributes of the schist.
Eastern Province – Dun Mountain-Maitai and Brook Street terranes
The Dun Mountain-Maitai and Brook Street terranes together yield sand with the most distinct lithic composition of the nonplutonic terranes ( and ). All sand-sized fractions from this basement grouping have a very similar modal mineralogy, with high lithic, moderate plagioclase, and low quartz contents, and a range of metamorphic to volcanic lithic proportions with minor sedimentary lithic components. These are all consistent with source terrane lithologies that include pyroxene gabbro with phenocrysts of augite and plagioclase, as well as ophiolite, mélange, argillite, basalt, pillow lava, serpentinite, greywacke, mudstone, and siltstone (e.g. Kimbrough et al. Citation1992; Rattenbury et al. Citation1998; Rahl et al. Citation2011). Perhaps the most distinct grain types are the common calcium-rich serpentine pseudomorphs of mafic igneous minerals such as olivine and augite, with bastite, mesh, and polygonal mesh-core textures. Though these fingerprints are easily recognisable, they may not survive transport over great distances, because detrital serpentine readily alters to carbonate and clay minerals (e.g. Yoshida et al. Citation2003). Basaltic to andesitic volcanic lithics (metabasites) are recognisable on the basis of altered microlitic texture. Other volcanic to metavolcanic lithics include some combination of quartz, potassium feldspar, muscovite/sericite, or recrystallised potassic glass with more mafic minerals such as augite, hornblende, or biotite in a dark groundmass. Higher-grade metavolcanic lithics, with some combination of plagioclase, epidote, and chlorite, appear to have later undergone retrograde metamorphism to prehnite-pumpellyite grade, resulting in formation of pumpellyite aggregates that partially obscure the volcanic clasts.
Plutonic Terrane sand comparison – Karamea Batholith, Paparoa Batholith, and Median Batholith (Separation Point Suite)
Sand from streams draining Karamea Batholith, Paparoa Batholith and Median Batholith plutonic rocks is similar in terms of QFL proportion, but has compositional differences: Median Batholith sand (note that we sampled only the Separation Point Suite as a constituent part of the Median Batholith in our study) contains fewer lithic fragments than the Paparoa or Karamea sand, and the Median Batholith QFL sand compositions cluster more tightly than the others. Furthermore, in terms of monomineralic QmKP proportions (), the Median Batholith sand has consistently higher proportions of plagioclase feldspar except in the finest sand fractions. Conversely, a higher proportion of potassium feldspar discriminates Karamea from Medium Batholith sand. The Paparoa Batholith within the Karamea Batholith generates sand of uniform QmKP composition in all size fractions that is distinctly more quartzose than all Median Batholith samples. Two Median Batholith samples show distinct size-fraction trends in QmKP proportions from highest monocrystalline quartz in the coarse-sand fraction to slightly higher potassium feldspar in the medium-sand fraction to plagioclase dominance in the finer-sand fractions ().
Comparison plots of feldspar types (calcium plagioclase, sodium plagioclase, and potassium feldspar) show that the Karamea Batholith-derived sand is generally richer in potassium feldspar while the Median Batholith-derived sand is richer in calcium plagioclase (). This is in keeping with the sodic, adakitic, I-type igneous suite of the Separation Point Batholith recently reported by Tulloch et al. (Citation2019). Also, microcline exhibiting tartan twinning is less abundant in the Median Batholith sand, making up 1 to 9% of grains counted, compared with 1% to 9% in the northernmost Karamea Batholith sample (NZ-13-20; this does not include sand from the Paparoa Batholith, which has <1%). Microcline grains are 2 to 7 times more numerous in the Karamea Batholith-derived sand (excluding the Paparoa sample) than in the Meridian Batholith-derived sand, particularly in the medium sand fraction. Feldpsar grains in particular are known to be affected by weathering and preferential abrasion during transport (Nesbitt et al. Citation1997; Garzanti et al. Citation2009). Size differences are also evident in other studies. Nesbitt et al. (Citation1997), for example, found that finer-grained sand fractions contained higher proportions of potassium feldspar grains than coarser-grained fractions, while Kautz and Martin (Citation2007) found that plagioclase proportions were higher in fine-grained sand than in medium- and coarse-grained sand.
Figure 9. A, Ternary diagram shows proportions of fresh and altered varieties of calcium feldspar (Ca), sodium feldspar (Na; unstained), and potassium feldspar (K) in very fine to very coarse sand from streams draining the Karamea and Median Batholiths. B, Ternary plot highlights the medium sand fraction data set shown in A. See and Supplementary Tables 3 and 4 for recalculated parameter definitions and results plotted in the ternary diagrams.
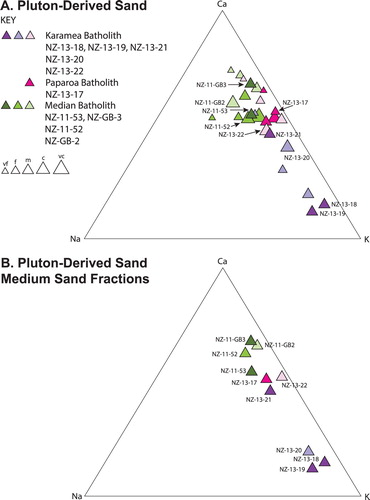
An additional difference is the presence of hornblende and epidote in the Median Batholith sand, grain types not present in Karamea Batholith sand (see Supplementary Table 3, https://doi.org/10.1080/00288306.2019.1618881). Mica abundances () are comparable in both batholiths, though somewhat higher in the Karamea Batholith.
Dense minerals () constitute <2% of the Karamea Batholith-derived samples, including those from the Paparoa Batholith, and range from 1 to 23% of grains counted in the Median Batholith sand, with the highest percentages in the fine- to very fine-sand fractions from the northernmost sample (NZ-13-20). Of course, the concentration of mica and denser grains may not be related only to relative abundances in the source rocks but also to hydrodynamics and settling equivalence, which has been known to concentrate dense minerals in finer-sand fractions over quartz and feldspar (e.g. Garzanti et al. Citation2008, Citation2009).
Lastly, the minor lithic fragment components associated with these plutoniclastic samples are various aggregates of feldspar, quartz, mica and/or dense minerals (Lma), a significantly different lithic population than that produced by the metamorphic terranes described above.
Results of this study are in general agreement with outcrop descriptions of the Karamea Batholith as a high-potassium, calc-alkaline suite (S-type granites) with distinctive, pink, white and black, medium- to coarse-grained granite with megacrysts of potassium feldspar, including orthoclase and microcline, in a groundmass of quartz, plagioclase, biotite ± muscovite, and a paucity of hornblende (Smale Citation1990; Muir et al. Citation1996; Rattenbury et al. Citation1998). Results are also in agreement with outcrop descriptions of the Paparoa Range (Tulloch and Palmer Citation1990; White Citation1994) as comprising brown and green-brown biotite granite to leucocratic granite along with metasedimentary rocks and gneisses, and the sodic, adakitic I-type igneous suite of the Separation Point Batholith (Tulloch et al. Citation2019).
As noted earlier, compositional differences do exist within the samples collected from within individual batholith terranes. Differences in the Karamea Batholith samples from the north to south could be attributed to several factors as described by Garzanti et al. (Citation2009): local mineral proportion variability in the source plutons, preferential removal of sand-sized plagioclase feldspar during weathering, or preferential abrasion during transport.
The provenance of Taranaki Basin sandstone reservoirs
This work provides the first systematic petrographic description and documentation of the detrital mineralogy of modern sands derived from basement terranes in northern South Island, and if our assumptions are correct, these data are the best approximation of the nature of the sand-sized detritus that these terranes are producing and have produced in the geological past. As such, the data have applications to the provenance of ancient petroleum-bearing Taranaki Basin reservoir sandstones where detrital composition is known to exert a first-order control on reservoir quality (e.g. Higgs and King Citation2018).
The petrology of Cretaceous to Cenozoic sandstone reservoirs has been the focus of several studies (e.g. Martin et al. Citation1994; Smale et al. Citation1999; Higgs et al. Citation2007, Citation2010; Browne et al. Citation2008; Higgs and King Citation2018; Rotzien et al. Citation2018). Work by Browne et al. (Citation2008), Higgs et al. (Citation2010), and Higgs and King (Citation2018) showed Late Cretaceous sandstone reservoirs to be quartzofeldspathic in composition (lithic feldsarenite to feldsarenite using Folk et al. Citation1970, nomenclature). They emphasised the role of first-cycle plutonic sources, either Karamea or Median batholiths, in determining their mineralogy but could not specify whether reservoir sand was derived from a specific or mixture of plutonic sources based on modal mineralogy alone. They also proposed input to the Rakopi and North Cape formations from other terranes, e.g. Buller Terrane by Browne et al. (Citation2008) and Rakaia and Takaka Terranes by Higgs et al. (Citation2010). More provenance information using detrital zircon geochronology allowed them to speculate on dominant sources and relative mixtures of sources for individual samples. We can only broadly compare the Cretaceous sandstones in the Taranaki Basin with the modern sand data because of nonuniform methodology (e.g. Gazzi-Dickinson vs. traditional point-counting vs. QEMSCAN techniques) to demonstrate potential application of our results.
In , we plot our data (mean values for sand from the various terranes) and several Cretaceous data sets, as well as a spectrum of data collected by Smale et al. (Citation1999) for Cretaceous to Cenozoic reservoirs. The QFL and QmKP proportions of Late Cretaceous reservoir sandstones in the Taranaki Basin reported in Higgs and King (Citation2018) are most similar to the modal compositions determined in this study for the Karamea Batholith sand, which we demonstrate tends to have more quartz (QFL and QmKP) than Median Batholith sand. Higgs and King’s (Citation2018) reservoir feldspar proportions are also more consistent with our data from the Karamea Batholith. It is important to note that Higgs and King (Citation2018) and others have tried to reconstruct original mineralogy by seeing through and compensating for the effects of burial diagenesis. Still, feldspar dissolution and alteration may explain some of the data spread in . Younger Paleogene sandstone reservoirs are even more quartzose, with greater compositional maturity, reflecting either recycling to enhance quartz abundance, or alteration and loss of more labile plagioclase feldspar and lithic grains during weathering (cf. Higgs and King Citation2018).
Figure 10. Composite of ternary diagrams compares QFL proportions for modern stream sands from this study with those of Taranaki Basin sandstone units, including Ternary diagrams showing average proportions of quartz (Q), feldspar (F), and lithic fragments (L) point counted in this study and individual data points for Taranaki Basin petroleum-bearing sandstones from Smale (Citation1991b), including the Rakopi, North Cape, Farewell, Kaimiro, Mangahewa, Tikorangi, Moki and Mount Messenger formations. Black symbols (X’s) represent individual well data from the Cretaceous Rakopi and Cretaceous North Cape formations, from Higgs et al. (Citation2010). The trend in QFL proportions over time suggests that transport and weathering of plutonic sand from the Karamea and Median batholiths initially led to feldspar- and quartz-rich sandstones of the Cretaceous Rakopi and North Cape formations and Paleocene Farewell Formations, followed by somewhat more quartz-rich sandstones of the Eocene Kaimiro Formation. By Late Eocene time, input from other terranes added more lithics to Taranaki Basin sandstones (Mangahewa, Moki, and Mount Messenger formations), as well as to sandstones in the Tikorangi limestone formation, a deep-water province to the northwest.
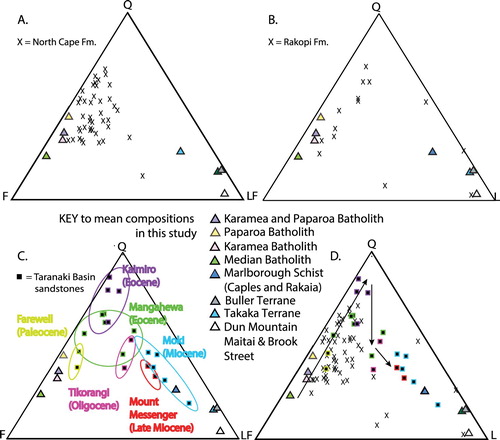
The trend in QFL proportions over time suggests that weathering and transport of plutonic sand from the Karamea, Paparoa, and Median batholiths may initially have produced the feldspar- and quartz-rich sandstones of the Cretaceous Rakopi and North Cape formations and Paleocene Farewell Formation, followed by more quartz-rich sandstones of the Eocene Kaimiro Formation. After that, input from other terranes may have added more lithics to younger Taranaki Basin sandstones, such as the Mangahewa, Moki, and Mount Messenger formations.
In our study, we also show distinct differences among sand fractions separated from some modern sand samples, suggesting finer sandstones may be more feldspathic, which has implications for reservoir quality. This may be related to mineral size variability within the source rocks, to fragmentation during transport owing to cleavage, or to effects of hydraulic sorting.
Toward an actualistic sand provenance model
The concept of using modern sand composition to determine ancient source has been widely used in the literature. Ingersoll (Citation1990) was the first to advance the concept, using the modern sand composition determined from known provenance areas, to statistically discriminate compositions, which he referred to as actualistic petrofacies. This approach is now widely used (Ingersoll Citation1990; Ingersoll and Eastmond Citation2007; Ingersoll et al. Citation2016; Vezzoli et al. Citation2017) but has been an under-utilised technique in New Zealand sedimentary basins. Although it is beyond the scope of this study to present the discriminate analysis of the larger data set required for an actualistic sand provenance model, the data presented here will contribute to such a model in the future.
Conclusion
We have demonstrated that the modal composition of modern fluvial (and beach) sands reflects basement rock compositions from which they were derived. The following are some key observations from this study:
In general, sand derived from metamorphic or metasedimentary basement can be distinguished from plutonic-derived sand based on modal composition and lithic content.
Sand from the Karamea Batholith has a higher proportion of monomineralic potassium feldspar, including microcline, as well as monocrystalline quartz.
Overall, sand derived from the Median Batholith tends to have less quartz than sand derived from the Karamea Batholith. Median Batholith-derived samples have a higher monomineralic percentage of plagioclase except in the finest sand fractions, and also hornblende and epidote.
Feldspar is rare in all the Eastern Province metamorphic terranes: Caples, Torlesse, and Dun Mountain-Maitai and Brook Street terranes. Dun Mountain-Maitai and Brook Street terrane sand has the lowest quartz content and is recognisable by distinctive Ca-rich, serpentine pseudomorphs after olivine and pyroxene, and pumpellyite-bearing, metavolcanic lithic fragments.
Marlborough Schist-derived sand is distinctive in having pervasive pumpellyite in lower-grade metamorphic lithic fragments, while higher-grade, greenschist rocks generate sand with more albite and rare potassium feldspar. Marlborough Schist-derived sand also contains epidote plus clinozoisite/zoisite.
Sand rich in lithic fragments may be sourced from the Eastern Province or the Takaka terranes; further differentation of the Takaka source may be possible on the basis of monocrystalline quartz vs. monocrystalline plagioclase proportions.
Metamorphic overprinting and alteration has rendered volcanic-arc sands from the Takaka Terrane mostly unrecognisable as volcanic lithics; however, these metasiltstone and metamudstone fragments with aggregates of pumpellyite are mostly absent in the Buller Terrane sand.
We suggest that the sand compositions from modern streams and beaches are reliable indicators of the composition of the paleostreams that may have sourced ancient sandstone reservoirs in the Taranaki Basin. Some sandstones may have been sourced from purely plutonic terranes, others from metamorphic terranes, others from mixtures of these. Previous provenance interpretations of reservoir rocks have emphasised monomineralic components (e.g. feldspar, dense minerals), which may be overprinted by diagenesis. We suggest that the lithic fractions may also be diagnostic but more detailed analysis of lithic types in reservoir sandstone is needed. Future work also should include collecting and petrographically analyzing an expanded suite of basement-derived modern sand samples, such as the mineralogy of stream sediment derived from the Rotoroa Igneous Complex (Median Batholith) to determine its similarity or differences to the Separation Point Suite reported on here. In addition, further sampling within the Takaka Terrane in particular would provide greater intra-Takaka lithological and intra-Takaka schist terrane variation. We recognise that our sample size points to clear major groupings that differentiate basement rock types but is based on too few samples. These features, together with provenance studies utilising other techniques such as heavy minerals and detrital zircon geochronology, will create more robust data to help create an actualistic sand provenance model for subsurface sandstones across Zealandia.
Supplementary Table 3 - Point-count Data
Download PDF (72.8 KB)Supplementary Table 4 - Recalculated Parameters
Download PDF (67.8 KB)Acknowledgements
For samples collected within Kahurangi and Abel Tasman National Parks, a permit to collect these samples was obtained from the Department of Conservation. Other samples were collected from public lands that did not require any special permitting. Thanks to Sarah Møller and Morten Nielsen for providing field assistance, good cheer, and expert left-side driving skills in New Zealand, and to Richard Heermance and Joshua Schwartz for their input during the project. Mark Rattenbury helped with . At California State University Northridge, additional support for this project was provided by the Office of Graduate Studies (KMM and LMD) and a Hanna fellowship (LMD). We thank journal reviewers Jon Rotzien and Nick Mortimer for their comments.
Disclosure statement
No potential conflict of interest was reported by the authors.
ORCID
Greg H. Browne http://orcid.org/0000-0003-0865-6146
Additional information
Funding
References
- Adams CJ, Kelley S. 1998. Provenance of Permian-Triassic and Ordovician metagraywacke terranes in New Zealand: evidence from 40Ar/39Ar dating of detrital micas. GSA Bulletin. 110:422–432. doi: 10.1130/0016-7606(1998)110<0422:POPTAO>2.3.CO;2
- Adams CJ, Mortimer N, Campbell HJ, Griffin WL. 2015. Detrital zircon ages in Buller and Takaka terranes, New Zealand: constraints on early Zealandia history. New Zealand Journal of Geology and Geophysics. 58:176–201. doi:10.1080/00288306.2015.1025798.
- Barnes PM. 2009. Postglacial (after 20 ka) dextral slip rate of the offshore Alpine fault, New Zealand. Geology. 37:3–6. doi: 10.1130/G24764A.1
- Begg JG, Johnston M.R, compilers. 2000. Geology of the Wellington Area: Lower Hutt, New Zealand, Institute of Geological and Nuclear Sciences Ltd., 64 p.
- Bender-Whitaker C, Marsaglia KM, Browne GH, Jaeger JM. 2018. Sedimentary processes and sequence stratigraphy of a Quaternary siliciclastic shelf-slope system: insights from sand provenance studies, Canterbury Basin, New Zealand. Contributions of W. Dickinson in Geoscience. Geological Society of America Special Paper 540. Edited by Ingersoll, R. V., Graham, S., Lawton, T. F., 159–196.
- Bishop DG, Bradshaw JD, Landis CA. 1985. Provisional Terrane Map of South Island, New Zealand. In Howell DG, editor. Tectonostratigraphic terranes of the circum pacific region: American Association of Petroleum Geologists, circum-pacific council for energy and mineral resources, Earth Science Series, Number 1. Houston (TX): Circum Pacific Council Publications; p. 515–521.
- Bjørlykke K. 1984. Formation of secondary porosity: how important is it. In: McDonald DA, Surdam RC, editors. Clastic diagenesis: American Association of Petroleum Geologists Memoir. 37, Tulsa (OK); p. 277–286.
- Blake MC, Landis CA. 1973. The Dun Mountain ultramafic belt–Permian oceanic crust and upper mantle in New Zealand. Journal of Research of the U.S. Geological Survey. 1:529–534.
- Blatt H, Christie JM. 1963. Undulatory extinction in quartz of igneous and metamorphic rocks and its significance in provenance studies of sedimentary rocks. Journal of Sedimentary Petrology. 33:559–579.
- Boggs JM, Adams EE. 1992. Field study of dispersion in a heterogeneous aquifer: 4. Investigation of adsorption and sampling bias. Water Resources Research. 28:3325–3336. doi: 10.1029/92WR01759
- Boggs JM, Young SC, Beard LM, Gelhar LW, Rehfeldt KR, Adams EE. 1992. Field study of dispersion in a heterogeneous aquifer: 1. Overview and site description. Water Resources Research. 28:3281–3291. doi: 10.1029/92WR01756
- Briggs RM, Middleton MP, Nelson CS. 2004. Provenance history of a Late Triassic-Jurassic Gondwana margin forearc basin, Murihiku Terrane, North Island, New Zealand: petrographic and geochemical constraints. New Zealand Journal of Geology & Geophysics. 47:589–602. doi: 10.1080/00288306.2004.9515078
- Brown EH. 1971. Phase relations of biotite and stilpnomelane in the greenschist facies. Contributions in Mineralogy and Petrology. 31:275–299. doi: 10.1007/BF00371150
- Browne GH, Kennedy EM, Constable RM, Raine IJ, Crouch EM, Sykes R. 2008. An outcrop-based study of the economically significant Late Cretaceous Rakopi Formation, northwest Nelson, Taranaki Basin, New Zealand. New Zealand Journal of Geology and Geophysics. 51:295–315. doi: 10.1080/00288300809509867
- Coombs DS. 1960. Lower grade mineral facies in New Zealand. In Sorgenfrei T, editor. Report of the 21st Session of the International Geological Congress, Copenhagen, 1960, Volume 13, Petrographic provinces, igneous and metamorphic rocks: Det Berlingske Bogtrykkeri, pp. 339–351.
- Cooper RA, Tulloch AJ. 1992. Early Palaeozoic terranes in New Zealand and their relationship to the Lachlan Fold Belt. Tectonophysics. 214:129–144. doi: 10.1016/0040-1951(92)90193-A
- Cox SC, Sutherland R. 2007. Regional geological framework of South Island, New Zealand, and its significance for understanding the active plate boundary. In: Okaya D, Stern T, Davey F, editors. A continental plate boundary: tectonics at South Island. Washington (DC): American Geophysical Union Geophysical Monograph Series 175; p. 19–46.
- Craw D. 1984. Lithologic variations in Otago Schist, Mt Aspiring area, northwest Otago, New Zealand. New Zealand Journal of Geology and Geophysics. 27:151–166. doi: 10.1080/00288306.1984.10422524
- Dickinson WR. 1985. Interpreting provenance relations from detrital modes of sandstones. In Zuffa GG, editor. Provenance of arenites: Cosenza, Italy, NATO Advanced Study Institute on Reading Provenance from Arenites (and D. Reidel Publishing Co.), p. 333–361.
- Doran L. 2014. Characterizing modern stream sand to better understand reservoir sandstone provenance in the Taranaki Basin, New Zealand [Unpublished MS thesis]. California State University Northridge, 130 p.
- Edbrooke SW, Heron DW, Forsyth PJ, Jongens R, compilers. 2015. Geological Map of New Zealand 1:1,000,000. GNS Science Geological Map 2. Lower Hutt.
- Folk RL, Andrews PB, Lewis DW. 1970. Detrital sedimentary rock classification and nomenclature for use in New Zealand. New Zealand Journal of Geology and Geophysics. 13:937–968. doi: 10.1080/00288306.1970.10418211
- Garzanti E, Andò S, Vezzoli G. 2008. Settling equivalence of detrital minerals and grain-size dependence of sediment composition. Earth and Planetary Science Letters. 273:138–151. doi: 10.1016/j.epsl.2008.06.020
- Garzanti E, Andò S, Vezzoli G. 2009. Grain-size dependence of sediment composition and environmental bias in provenance studies. Earth and Planetary Science Letters. 277:422–432. doi: 10.1016/j.epsl.2008.11.007
- Garzanti E, Vezzoli G. 2003. A classification of metamorphic grains in sands based on their composition and grade. Journal of Sedimentary Research. 73:830–837. doi: 10.1306/012203730830
- Hayward BW, Smale D. 1992. Heavy minerals and the provenance history of Waitemata Basin sediments (Early Miocene, Northland, New Zealand). New Zealand Journal of Geology and Geophysics. 35:223–242. doi: 10.1080/00288306.1992.9514516
- Higgs KE, Arnot MJ, Browne GH, Kennedy EM. 2010. Reservoir potential of Late Cretaceous terrestrial to shallow marine sandstones, Taranaki Basin, New Zealand. Marine and Petroleum Geology. 27:1849–1871. doi: 10.1016/j.marpetgeo.2010.08.002
- Higgs KE, Crouch EM, Raine JI. 2017. An interdisciplinary approach to reservoir characterisation; an example from the early to middle Eocene Kaimiro Formation, Taranaki Basin, New Zealand. Marine and Petroleum Geology. 86:111–139. doi: 10.1016/j.marpetgeo.2017.05.018
- Higgs KE, King PR. 2018. Sandstone provenance and sediment dispersal in a complex tectonic setting: Taranaki Basin, New Zealand: sediment provenance and dispersal pathways. Sedimentary Geology. 372:112–139. doi: 10.1016/j.sedgeo.2018.05.004
- Higgs KE, King PR, Raine JI, Sykes R, Browne GH, Crouch EM, Baur JR. 2012. Sequence stratigraphy and controls on reservoir sandstone distribution in an Eocene marginal marine-coastal plain fairway, Taranaki Basin, New Zealand. Marine and Petroleum Geology. 32:110–137. doi: 10.1016/j.marpetgeo.2011.12.001
- Higgs KE, Zwingmann H, Reyes AG, Funnell RH. 2007. Diagenesis, porosity evolution, and petroleum emplacement in tight gas reservoirs, Taranaki Basin, New Zealand. Journal of Sedimentary Research. 77:1003–1025. doi: 10.2110/jsr.2007.095
- Ingersoll RV. 1990. Actualistic sandstone petrofacies: discriminating modern and ancient source rocks. Geology. 18:733–736. doi: 10.1130/0091-7613(1990)018<0733:ASPDMA>2.3.CO;2
- Ingersoll RV, Bullard TF, Ford RL, Grimm JP, Pickle JD, Sares SW. 1984. The effect of grain size on detrital modes: a test of the Gazzi-Dickinson point-counting method. Journal of Sedimentary Petrology. 54:103–116.
- Ingersoll RV, Eastmond DJ. 2007. Composition of modern sand from the Sierra Nevada, California, U.S.A.: implications for actualistic petrofacies of continental-margin magmatic arcs. Journal of Sedimentary Research. 77:784–796. doi: 10.2110/jsr.2007.071
- Ingersoll RV, Steinpress MG, Spangler D. 2016. Application of actualistic sand petrofacies in hydrogeology: An example from the northern Sacramento Valley, California, USA. GSA Bulletin. 128:661–668.
- James DE, DeVaughn AM, Marsaglia KM. 2007. Sand and gravel provenance in the Waipaoa River System: Sedimentary recycling in an actively deforming forearc basin, North Island, New Zealand. Special Paper Geological Society of America v. 420, Sedimentary provenance and petrogenesis: Perspectives from petrography and geochemistry: J. Arribas et al., eds., 253–276.
- Johnston MR. 1974. Geology of the Mount Arthur district, North-west Nelson. New Zealand Journal of Geology and Geophysics. 17:75–92. doi: 10.1080/00288306.1974.10428477
- Jongens R. 2006. Structure of the Buller and Takaka Terrane rocks adjacent to the Anatoki Fault, northwest Nelson, New Zealand. New Zealand Journal of Geology and Geophysics. 49:443–461. doi: 10.1080/00288306.2006.9515180
- Kautz CQ, Martin CE. 2007. Chemical and physical weathering in New Zealand’s Southern Alps monitored by bedload sediment major element composition. Applied Geochemistry. 22:1715–1735. doi: 10.1016/j.apgeochem.2007.03.031
- Kear D. 1971. Basement rock facies—Northern North Island. New Zealand Journal of Geology and Geophysics. 14:275–283. doi: 10.1080/00288306.1971.10421924
- Kimbrough DL, Mattinson JM, Coombs DS, Landis CA, Johnston MR. 1992. Uranium-lead ages from the Dun Mountain ophiolite belt and Brook Street terrane, South Island, New Zealand. Geological Society of America Bulletin. 104:429–443. doi: 10.1130/0016-7606(1992)104<0429:ULAFTD>2.3.CO;2
- Laird MG, Shelley D. 1974. Sedimentation and early tectonic history of the Greenland group, Reefton, New Zealand. New Zealand Journal of Geology and Geophysics. 17:839–854. doi: 10.1080/00288306.1974.10418229
- Landis CA, Campbell HJ, Aslund T, Cawood PA, Douglas A, Kimbrough DL, Pillai DDL, Raine JI, Willsman A, 1999. Permian-Jurassic strata at Productus Creek, Southland, New Zealand: Implications for terrane dynamics of the eastern Gondwanaland margin. New Zealand Journal of Geology and Geophysics. 42:255–278. doi: 10.1080/00288306.1999.9514844
- MacKinnon TC. 1983. Origin of the Torlesse terrane and coeval rocks, South Island, New Zealand. Geological Society of America Bulletin. 94:967–985. doi: 10.1130/0016-7606(1983)94<967:OOTTTA>2.0.CO;2
- Marsaglia KM, DeVaughn AM, James DE, Marden M. 2010. Provenance of fluvial terrace sediments within the Waipaoa sedimentary system and their importance to New Zealand source-to-sink studies. Marine Geology. 270:84–93. doi: 10.1016/j.margeo.2009.10.017
- Marsaglia KM, Martin CE, Kautz CQ, Shapiro SA, Carter L. 2011. Linking a late Miocene–Pliocene hiatus in the deep-sea Bounty Fan off South Island, New Zealand, to onshore tectonism and lacustrine sediment storage. Geosphere. 7:305–312. doi: 10.1130/GES00621.1
- Marsaglia KM, Tazaki K. 1992. Diagenetic trends in Leg 126 sandstones. In: Taylor B, Fujioka K et al., editors. Proceedings of the Ocean Drilling Program, Scientific Results. College Station (TX): Ocean Drilling Program, 126, p. 125–138.
- Martin KR, Baker JC, Hamilton PJ, Thrasher GP. 1994. Diagenesis and reservoir quality of Paleocene sandstones in the Kupe South field, Taranaki Basin, New Zealand. American Association of Petroleum Geologists Bulletin. 78:624–643.
- Mortimer N. 1993. Metamorphic zones, terranes, and Cenozoic faults in the Marlborough schist, New Zealand. New Zealand Journal of Geology and Geophysics. 36:357–368. doi: 10.1080/00288306.1993.9514581
- Mortimer N. 1994. Origin of the Torlesse Terrane and Coeval rocks, North Island, New Zealand. International Geology Review. 36:891–910. doi: 10.1080/00206819409465494
- Mortimer N. 2004. New Zealand’s geological foundations. Gondwana Research. 7:261–272. doi: 10.1016/S1342-937X(05)70324-5
- Mortimer N. 2009. Zealandia’s Early Paleozoic sandstones: detrital mineralogy of the Greenland and Reefton Groups. p. 305–313 IN: Australasian Institute of Mining and Metallurgy, 42nd New Zealand Branch annual conference. AUSIMM NZ Branch 42. https://data.gns.cri.nz/bib/abstract.jsp?id=173388.
- Mortimer N, Little T. 1998. Altered biotites in the Marlborough schist, New Zealand? New Zealand Journal of Geology and Geophysics. 41:105–109. doi:10.1080/00288306.1998.9514794.
- Mortimer N, McLaren S, Dunlap WJ. 2012. Ar-Ar dating of K-feldspar in low grade metamorphic rocks: example of an exhumed Mesozoic accretionary wedge and forearc, South Island, New Zealand. Tectonics. 31:1–15. doi: 10.1029/2011TC003057
- Mortimer N, Nathan S, Jongens R, Kawachi Y, Ryland C, Cooper AF, Stewart M, Randall S. 2013. Regional metamorphism of the Early Palaeozoic Greenland group, South Westland, New Zealand. New Zealand Journal of Geology and Geophysics. 56:1–15. doi:10.1080/00288306.2012.734830.
- Mortimer N, Tulloch AJ, Ireland TR. 1997. Basement geology of Taranaki and Wanganui basins, New Zealand. New Zealand Journal of Geology and Geophysics. 40:223–236. doi: 10.1080/00288306.1997.9514754
- Mortimer N, Tulloch AJ, Spark AN, Walker NW, Ladley E, Allibone A, Kimbrough DL. 1999. Overview of the median batholith, New Zealand: a new interpretation of the geology of the median tectonic zone and adjacent rocks. Journal of African Earth Sciences. 29:257–268. doi: 10.1016/S0899-5362(99)00095-0
- Mortimer N, Wopereis P. 1997. Change in direction of the Pelorus River, Marlborough, New Zealand: evidence from composition of Quaternary gravels. New Zealand Journal of Geology and Geophysics. 40:307–313. doi:10.1080/00288306.1997.9514763.
- Mueller M. 1991. Karst hydrogeology of the Takaka valley, golden bay, Northwest Nelson. New Zealand Journal of Geology and Geophysics. 34:11–16. doi: 10.1080/00288306.1991.9514434
- Muir RJ, Weaver SD, Bradshaw JD, Eby GN, Evans JA. 1995. The cretaceous separation point batholith, New Zealand: granitoid magmas formed by melting of mafic lithosphere. Journal of the Geological Society. 152:689–701. doi: 10.1144/gsjgs.152.4.0689
- Muir RJ, Weaver SD, Bradshaw JD, Eby GN, Evans JA, Ireland TR. 1996. Geochemistry of the Karamea Batholith, New Zealand, and comparisons with the Lachlan Fold Belt granites of SE Australia. Lithos. 39:1–20. doi: 10.1016/S0024-4937(96)00017-5
- Münker C, Cooper R. 1999. The Cambrian arc complex of the Takaka Terrane, New Zealand: an integrated stratigraphical, paleontological and geochemical approach. New Zealand Journal of Geology and Geophysics. 42:415–445. doi: 10.1080/00288306.1999.9514854
- Nesbitt HW, Fedo CM, Young GM. 1997. Quartz and feldspar stability, steady and non-steady-state weathering, and petrogenesis of siliciclastic sands and muds. The Journal of Geology. 105:173–192. doi: 10.1086/515908
- Noda A, Takeuchi M, Adachi M. 2004. Provenance of the Murihiku Terrane, New Zealand: evidence from the Jurassic conglomerates and sandstones in Southland. Sedimentary Geology. 164:203–222. doi: 10.1016/j.sedgeo.2003.10.003
- Parra JG, Marsaglia KM, Rivera KS, Dawson ST, Walsh JP. 2012. Provenance of sand on the poverty bay shelf, the link between source and sink sectors of the Waipaoa River sedimentary system. Sedimentary Geology. 280:208–233. doi: 10.1016/j.sedgeo.2012.04.012
- Pound K. 1993. Geology of the lower Paleozoic Haupiri group rocks, Cobb valley area, Northwest Nelson, New Zealand (Unpublished PhD dissertation). University of Otago, New Zealand, 172 p.
- Rahl JM, Brandon MT, Deckert H, Ring U, Mortimer N. 2011. Tectonic significance of ductile deformation in low-grade sandstones in the Mesozoic Otago subduction wedge, New Zealand. American Journal of Science. 311:27–62. doi: 10.2475/01.2011.02
- Randall K, Lamb S, Conall MN. 2011. Large tectonic rotations in a wide zone of Neogene distributed dextral shear, northeastern South Island, New Zealand. Tectonophysics. 509:165–180. doi: 10.1016/j.tecto.2011.05.006
- Rattenbury MS, Cooper RA, Johnston MR, compilers. 1998. Geology of the Nelson Area. New Zealand, Institute of Geological and Nuclear Sciences, Lower Hutt. QMAP Geological Map 9, scale 1:250,000, 1 sheet and 67 p.
- Roser BP, Cooper RA, Nathan S, Tulloch AJ. 1996. Reconnaissance sandstone geochemistry, provenance, and tectonic setting of the lower Paleozoic terranes of the West Coast and Nelson, New Zealand. New Zealand Journal of Geology and Geophysics. 39:1–16. doi: 10.1080/00288306.1996.9514690
- Rotzien JR, Browne GH, King PR. 2018. Geochemical, petrographic, and uranium–lead geochronological evidence for multisourced polycyclic provenance of deep-water strata in a hybrid tectonic setting: The upper Miocene upper Mount Messenger Formation, Taranaki Basin, New Zealand. American Association of Petroleum Geologists Bulletin. 102:1763–1802. doi:10.1306/0206181616817222.
- Shapiro SA, Marsaglia KM, Carter L. 2007. The petrology and provenance of sand in the Bounty Submarine Fan, New Zealand: Geological Society of America Special Paper 420, Sedimentary provenance and petrogenesis. Perspectives from petrography and geochemistry. J. Arribas et al., eds. 277–296.
- Skinner DNB, Brathwaite RL. 2002. Wakamarina Quartzite and associated mafic rocks of Pelorus group, Marlborough: Geochemistry and origins. New Zealand Journal of Geology and Geophysics. 45:175–192. doi: 10.1080/00288306.2002.9514967
- Smale D. 1988. Detrital pumpellyite and epidote group minerals in Cretaceous and Tertiary sandstones in the South Island, New Zealand. Journal of Sedimentary Petrology. 58:985–991.
- Smale D. 1990. Distribution and provenance of heavy minerals in the South Island: a review. New Zealand Journal of Geology and Geophysics. 33:557–571. doi: 10.1080/00288306.1990.10421374
- Smale D. 1991a. Distribution and provenance of sand in the Wanganui River. In: Research Notes 1990. NZ Zealand Geological Survey Record 43. Lower Hutt: DSIR; p. 7–13.
- Smale D. 1991b. Provenance of sediments in Taranaki Basin – an assessment from heavy minerals. In: Petroleum Conference Proceedings: NZ Petroleum & Minerals. p. 245–254.
- Smale D. 1992. Provenance of sediments in Taranaki Basin: an assessment from heavy minerals. In: NZ Oil Exploration Conference Proceedings, Ministry of Commerce; Wellington. p. 245–254.
- Smale D. 1993. Sediment source and movement in Tauranga Harbour and nearshore Bay of Plenty. GNS Science Report 93/25, GNS Science, Lower Hutt. 20 p.
- Smale D. 1997. Heavy minerals in the Torlesse terrane of the Wellington area, New Zealand. New Zealand Journal of Geology and Geophysics. 40:499–506. doi: 10.1080/00288306.1997.9514779
- Smale D. 2007. Sediment trails in tectonically active islands: heavy minerals in use in New Zealand. In: Mange MA, Wright DT, editor. Heavy minerals in use. Developments in sedimentology 58. Amsterdam: Elsevier; p. 569–585.
- Smale D, Mauk JL, Palmer J, Soong R, Blattner P. 1999. Variations in sandstone diagenesis with depth, time, and space, onshore Taranaki wells, New Zealand. New Zealand Journal of Geology and Geophysics. 42:137–154. doi: 10.1080/00288306.1999.9514836
- Stipp MS, Heilbronner RH, Schmid S. 2002. The eastern Tonale fault zone: a ‘natural laboratory’ for crystal plastic deformation of quartz over a temperature range from 250 to 700°C. Journal of Structural Geology. 24:1861–1884. doi: 10.1016/S0191-8141(02)00035-4
- Strogen DP, compiler. 2011. Updated paleogeographic maps for the Taranaki Basin and surrounds. GNS Science Report 53, 83 p.
- Sutherland R. 1999. Cenozoic bending of New Zealand basement terranes and Alpine Fault displacement: A brief review. New Zealand Journal of Geology and Geophysics. 42:295–301. doi: 10.1080/00288306.1999.9514846
- Tulloch AJ. 1983. Granitoid rocks of New Zealand – a brief review. Geological Society of America Memoirs. 159:5–20. doi: 10.1130/MEM159-p5
- Tulloch AJ. 1988. Batholiths, plutons, and suites: nomenclature for granitoid rocks of Westland—Nelson, New Zealand. New Zealand Journal of Geology and Geophysics. 31:505–509. doi: 10.1080/00288306.1988.10422147
- Tulloch AJ, Kimbrough DL, Landis CA, Mortimer N. 1999. Relationships between the Brook Street Terrane and Median Tectonic Zone (Median Batholith): evidence from Jurassic conglomerates. New Zealand Journal of Geology and Geophysics. 42:279–293. doi: 10.1080/00288306.1999.9514845
- Tulloch AJ, Mortimer N, Ireland TR, Waight TE, Maas R, Palin JM, Sahoo T, Seebeck H, Sagar MW, Barrier A, Turnbull RE. 2019. Reconnaissance basement geology and tectonics of South Zealandia. Tectonics. 38:516–551. doi:10.1029/2018TC005116.
- Tulloch AJ, Palmer K. 1990. Tectonic implications of granite cobbles from the mid-Cretaceous Pororari group, southwest Nelson, New Zealand. New Zealand Journal of Geology and Geophysics. 33:205–217. doi: 10.1080/00288306.1990.10425679
- Tulloch AJ, Ramezani J, Kimbrough DL, Faure K, Allibone AH. 2009. U-Pb geochronology of mid-Paleozoic plutonism in western New Zealand: implications for S-type granite generation and growth of the east Gondwana margin. Geological Society of America Bulletin. 121:1236–1261. doi: 10.1130/B26272.1
- Turnbull IM. 1979. Petrography of the Caples terrane of the Thomson Mountains, northern Southland, New Zealand. New Zealand Journal of Geology and Geophysics. 22:709–727. doi: 10.1080/00288306.1979.10424178
- Turnbull IM. 1980. Structure and interpretation of the Caples Terrane of the Thomson Mountains, northern Southland, New Zealand. New Zealand Journal of Geology and Geophysics. 23:43–62. doi: 10.1080/00288306.1980.10424191
- Turnbull IM, Allibone AH. 2003. Geology of the Murihiku Area. New Zealand, Institute of Geological and Nuclear Sciences, Lower Hutt. QMAP Geological Map 20, scale 1:250,000, 1 sheet and 74 p.
- Turnbull IM, Mortimer N, Craw D. 2001. Textural zones in the Haast schist—a reappraisal. New Zealand Journal of Geology and Geophysics. 44:171–183. doi:10.1080/00288306.2001.9514933.
- Vezzoli G, Lombardo B, Rolfo F. 2017. Petrology of the tista and rangit river sands (Sikkim, India). Italian Journal of Geosciences. 136:103–109. doi: 10.3301/IJG.2016.04
- Walcott RI. 1998. Modes of oblique compression: Late Cenozoic tectonics of the South Island of New Zealand. Reviews of Geophysics. 36:1–26. doi: 10.1029/97RG03084
- White PJ. 1994. Thermobarometry of the Charleston metamorphic group and implications for the evolution of the Paparoa metamorphic core complex, New Zealand. New Zealand Journal of Geology and Geophysics. 37:201–209. doi: 10.1080/00288306.1994.9514615
- Yoshida K, Taki S, Iba Y, Sugawara M, Hikida Y. 2003. Discovery of serpentinite bearing conglomerate in the lower Yezo group, Hokkaido, northern Japan and its tectonic significance. Journal of the Geological Society of Japan. 109:336–344. doi: 10.5575/geosoc.109.336