ABSTRACT
Sr-Nd-Hf-Pb isotopes and major/trace element data are presented for amphibole megacrysts from two separate instances of lamprophyric intraplate magmatism in Zealandia (Cretaceous Westland Dike Swarm and Cenozoic Alpine Dike Swarm). Megacrysts from both regions have comparable geochemical and isotopic signatures (87Sr/86Sr(i) = 0.702754–0.703371, εNd(i) = +3.9 to +5.5, εHf(i) = +5.0 to +8.2, 206Pb/204Pb(i) = 18.909–20.526, 207Pb/204Pb(i) = 15.642–15.678, 208Pb/204Pb(i) = 38.824–40.271), regardless of them having traversed contrasting crustal terranes at different times and tectonic settings. Several megacryst cores may represent early-crystallised phenocrysts, whereas rims crystallised in equilibrium with amphibole microphenocrysts during final emplacement of host melts. Strontium isotopic offsets between megacrysts and host rocks indicate secondary hydrothermal alteration in some lamprophyres. Correlations between host rock – megacryst geochemistry as well as evidence for amphibole fractionation in Zealandia lamprophyres indicate that megacrysts likely crystallised from melts similar to their hosts. Isotopic (Pb, Nd) and Mg# offsets suggest a non-cognate relationship and reflect interaction between isotopically heterogeneous lamprophyric melts and/or cumulate amphibole in the lower crust. Modelling suggests that most alkaline intraplate magmatism throughout Zealandia may be derived from moderately heterogeneous SCLM sources that experienced localised varying degrees of subduction-related carbonatitic metasomatism between the Jurassic to Middle Cretaceous during Gondwana break-up.
Introduction
Lamprophyres are a group of igneous rocks that are volumetrically minor and inherently restricted to continental settings. These rocks were largely considered as enigmatic curiosities up until the works summarised in Rock (Citation1991), which defined lamprophyres as volatile-rich mantle-derived alkaline igneous rocks carrying hydrous minerals such as amphibole and biotite within a feldspar-rich groundmass. Since then, lamprophyres have been used extensively to constrain the nature and origin of the sub-continental lithospheric mantle (SCLM) through their geochemistry (e.g. Foley et al. Citation2002). Moreover, lamprophyre xenolith and xenocryst assemblages provide direct samples of the SCLM (e.g. Griffin et al. Citation2004; Pandey et al. Citation2018).
An ideal location for the study of lamprophyres and their crystal and xenolith assemblages is New Zealand. Since the Late Cretaceous and separation of Zealandia from Australia and Antarctica, repeated intraplate volcanism has erupted sporadically and indiscriminately within different crustal blocks of Zealandia (e.g. Timm et al. Citation2010; van der Meer et al. Citation2016). Such prolonged and widespread intraplate alkaline volcanism in Zealandia has provided an invaluable tool for probing the nature of the SCLM and its evolution during the past ~ 100 Ma. Several studies have argued for the tapping of a HIMU-like source component based on Zealandia intraplate alkaline volcanism displaying geochemical compositions and Sr, Nd, Hf ± Pb isotopic signatures similar to ocean island basalts (e.g. Hoernle et al. Citation2006; Sprung et al. Citation2007; van der Meer et al. Citation2017; Scott et al. Citation2020). However, the nature of this HIMU-like component and the mechanisms behind its repeated tapping remain debated. Nevertheless, the emplacement of comparable intraplate lamprophyres at different times in Zealandia as well as in different crustal blocks may have important implications for the apparent offset in crustal and mantle histories identified through studies of mantle xenoliths in Zealandia.
The presence of megacrystic amphibole is a characteristic feature of some of Zealandia intraplate lamprophyres. The petrogenetic nature of amphibole megacrysts in alkaline intraplate lamprophyres remains a debated subject. Intraplate mafic alkaline volcanism carrying amphibole megacrysts and amphibole-bearing mantle xenoliths has been reported worldwide (e.g. Kesson and Price Citation1972; Boettcher and O’Neil Citation1980; Irving and Frey Citation1984; Dautria et al. Citation1987; Shaw and Eyzaguirre Citation2000; Dobosi et al. Citation2003; Coltorti et al. Citation2007; Han et al. Citation2008; Fulmer et al. Citation2010; van der Meer et al. Citation2013). Amphibole megacrysts almost exclusively belong to the magnesio-hastingsite-pargasite-kaersutite series and have been interpreted as either: (1) high-pressure cognate phenocrysts (e.g. Binns Citation1969; Merrill and Wyllie Citation1975; Irving and Frey Citation1984; Mayer et al. Citation2014; Ulrych et al. Citation2018); (2) xenocrystic fragments of hydrous amphibole-rich pegmatitic veins in peridotites from the SCLM (e.g. Wilshire and Trask Citation1971; Best Citation1974; Boettcher and O’Neil Citation1980; Shaw and Eyzaguirre Citation2000; Dobosi et al. Citation2003); or (3) antecrysts that evolved in open system magma-plumbing systems (e.g. Ubide et al. Citation2014).
This study focuses on megacrystic amphiboles entrained in spatially and temporally decoupled pulses of intraplate alkaline magmatism in southern New Zealand. The aims of this paper are: (1) to constrain variations in major element, trace element and Sr-Nd-Hf-Pb isotope characteristics of megacrystic amphiboles occurring in different intrusives of South Island, New Zealand; (2) to determine the relationship between megacrystic amphiboles and their host rocks; and (3) thereby attempt to constrain the petrogenesis of these megacrystic amphiboles with concomitant implications for the origin of their host magmas.
Geological setting
The islands of New Zealand represent parts of the largely submerged (∼94%) Zealandia continent (>3.5 × 106 km2) in the southwestern Pacific (A) (Mortimer et al. Citation2017). This continent was situated adjacent to the Australian and Antarctica continents prior to the breakup of the Gondwana supercontinent in the Late Cretaceous. Zealandia formed by the accretion of batholith intrusions and fore-arc sedimentary and volcanic terranes onto the eastern convergent margin of Gondwana during the Phanerozoic and Mesozoic (ca. 520–100 Ma) (Mortimer Citation2004). This enduring accretionary subduction-dominated regime ended around 105–100 Ma and was substituted by crustal extension, which continued until the separation of Zealandia from West Antarctica and Australia and the onset of sea-floor spreading at ca. 85 Ma (Gaina et al. Citation1998; Waight et al. Citation1998a, Citation1998b; Laird and Bradshaw Citation2004; van der Meer et al. Citation2016). Sea-floor spreading resulted in the opening of the Tasman Sea and Zealandia consequently drifted northwest to its present-day location (Gaina et al. Citation1998). Zealandia is divided in two major provinces based on the age of basement rocks. Namely, the older early Palaeozoic (Cambrian to Early Devonian) Western Province (WP) and the younger Permian to mid-Cretaceous Eastern Province (EP) (Mortimer Citation2004) (). The Median Batholith, the plutonic root of a long-lived Devonian to Early Cretaceous cordilleran arc (Mortimer Citation2004; Scott Citation2013), separates and stitches both provinces (B).
Figure 1. A, Map of present-day Zealandia showing occurrences of intraplate magmatism. B, Map of southern New Zealand with sample locations modified from Cooper et al. (Citation1987). Ages for WDS samples are from (van der Meer et al. Citation2013), whereas ages for ADS samples are determined based on several studies (e.g. Cooper et al. Citation1987; Adams and Cooper Citation1996; Hoernle et al. Citation2006; Timm et al. Citation2010; Cooper Citation2020).
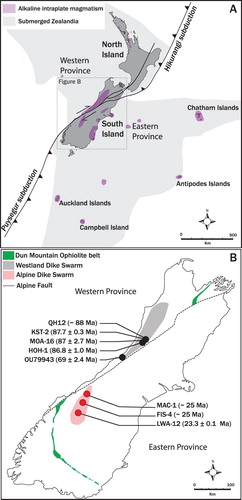
Since the cessation of the subduction-dominated regime in the Middle Cretaceous, intraplate volcanism has been widespread on the Zealandia continent (van Der Meer et al. Citation2018). Mafic intraplate volcanic rocks in Zealandia predominantly have alkaline compositions and Sr-Nd-Hf-Pb signatures approaching HIMU-like compositions, with 87Sr/86Sr(i) ∼ 0.703–704, εNd(i) = +2 to +8, εHf(i) = +2 to +10, 206Pb/204Pb(i) = 18.5–20.7 and 208Pb/204Pb(i) = 38.5–41 (Price Citation2003; Hoernle et al. Citation2006; Panter Citation2006; Sprung et al. Citation2007; Timm et al. Citation2009, Citation2010; McCoy-West et al. Citation2010; van der Meer et al. Citation2017; Hoernle et al. Citation2020; Scanlan et al. Citation2020; Scott et al. Citation2020). The sources behind such signatures in Zealandia intraplate magmas are still under debate. Some authors favour melting of a metasomatically enriched SCLM source (Panter Citation2006; McCoy-West et al. Citation2010; Scott et al. Citation2016a; van der Meer et al. Citation2017) while others favour melting of an asthenospheric source (Hoernle et al. Citation2006; Timm et al. Citation2010; Hoernle et al. Citation2020) or a combination of both (Finn et al. Citation2005; Sprung et al. Citation2007). The trigger of Cretaceous intraplate magmatism has generally been attributed to decompression of lithosphere thinned during extension associated with the opening of the Tasman Sea (Waight et al. Citation1998a, Citation1998b; van der Meer et al. Citation2016, Citation2017). Initiation of Cenozoic intraplate magmatism has been attributed to either the destabilisation of a hydrous lithospheric mantle (Panter Citation2006; Sprung et al. Citation2007; van der Meer et al. Citation2017) or decompression of the asthenosphere following delamination of the SCLM (e.g. Hoernle et al. Citation2006; Timm et al. Citation2010).
This paper focuses on intraplate lamprophyric dikes carrying megacrystic amphiboles in Zealandia. Such dikes are both spatially and temporally widespread, occurring in both the Western and Eastern Provinces of the South Island, and intruding in the Late Cretaceous and in the Cenozoic, respectively (e.g. Cooper et al. Citation1987; Waight et al. Citation1998a; van der Meer et al. Citation2013; Cooper Citation2020). Lamprophyric to phonolitic intraplate magmatism in the WP has been reported in the alkaline Westland Dike Swarm (WDS) centred on the Paparoa and Hohonu Ranges (B). These dikes vary in width from 1 cm to 15 m and display sharp intrusive contacts with their host Cretaceous granitoids (e.g. Waight et al. Citation1998a). Intrusion ages in the WDS have been constrained by 40Ar/39Ar ages from groundmass and megacrystic kaersutite in lamprophyric dikes to a first event at 90–84 Ma, with a second pulse around 72–68 Ma (van der Meer et al. Citation2013, Citation2016). The generation and emplacement of these dikes has been interpreted to be related to the extensional regime dominating during the opening of the Tasman Sea (Waight et al. Citation1998a; van der Meer et al. Citation2016). Similar lamprophyric dikes hosting megacrystic amphibole have been documented in the Alpine Dike Swarm (ADS) located in the EP (B). The ADS differs slightly from the WDS as it also includes carbonatite dikes (Cooper and Paterson Citation2008; Cooper Citation2020). Lamprophyric dikes of the ADS vary in thickness from centimetres to several tens of metres and show sharp intrusive contacts with the Haast Schist host rock (e.g. Cooper Citation2020). The ADS is significantly younger than the WDS and was emplaced around 25–23 Ma (Cooper et al. Citation1987; Adams and Cooper Citation1996; Hoernle et al. Citation2006; Timm et al. Citation2010; Cooper Citation2020) in a thickened lithosphere, and intruded along shear zones associated with the Alpine Fault plate boundary (Cooper Citation2020). ADS magmatism was contemporaneous with widespread Cenozoic intraplate activity in the South Island (Price Citation2003; Hoernle et al. Citation2006; Sprung et al. Citation2007; Timm et al. Citation2010; Scanlan et al. Citation2020; Scott et al. Citation2020).
The Zealandia SCLM has been investigated through studies of mantle xenoliths entrained by alkaline magmas (e.g. McCoy-West et al. Citation2013, Citation2015, Citation2016; Scott et al. Citation2014a, Citation2014b; Scott et al. Citation2016a, Citation2016b; Dalton et al. Citation2017; Scott et al. Citation2019; Scott Citation2020) as well as the exhumed Anita Peridotite (e.g. Czertowicz et al. Citation2016). While the Anita Peridotite has been interpreted to represent Cretaceous sub-arc lithospheric mantle, spinel-facies peridotite xenoliths revealed signs of widespread but variable mantle metasomatism in the Zealandia SCLM. Geochemical evidence from clinopyroxene suggests that peridotites reacted with carbonatite/CO2-rich silicate melts, which imprinted HIMU-like Sr, Nd and Pb isotopic signatures onto the SCLM (Scott et al. Citation2014a, Citation2014b; McCoy-West et al. Citation2016; Scott et al. Citation2016b; Dalton et al. Citation2017). Present-day Moho depth has been constrained to vary between 35 and 48 km (Salmon et al. Citation2013) in the ADS and has been assumed to be comparable during the Cenozoic (e.g. ∼ 37 km in Scott et al. Citation2014b). Late Cretaceous Moho depth in the WDS has not been constrained, but may be comparable to ∼ 30 km as in averaged extensional settings (Christensen and Mooney Citation1995).
Materials and methods
Methods
Major element compositions of amphibole were determined using a JEOL JXA-8200 Superprobe at the Department of Geosciences and Natural Resource Management, University of Copenhagen. Trace element compositions of amphibole were determined by LA-ICP-MS at GEUS (Geological Survey of Denmark and Greenland). Isotopic compositions of amphibole megacrysts were determined at the University of Copenhagen (Sr, Nd), University of Cape Town (Pb) and University of Cologne (Hf). A detailed description of methodologies and standards as well as data that support the findings of this study are openly available in Figshare at 10.6084/m9.figshare.12057843.
Studied samples
Westland Dike Swarm (WDS)
Four samples originating from a lamprophyre ± minor phonolite dike suite in the WDS were chosen due to the occurrence of megacrystic amphiboles. Samples QH12, KST-2, MOA-16 and HOH-1 were collected in the area around Lake Brunner in the Hohonu Dike Swarm (B). Hohonu Dike Swarm host rocks were emplaced in the Late Cretaceous (∼ 88 Ma) (van der Meer et al. Citation2013). The KST-2 sample was collected from a composite dike composed of both lamprophyre and phonolite (Waight et al. Citation1998a). Sample QH12 originates from a lamprophyric loose boulder collected near the KST-2 dike. Sample OU79943 was collected in the Bonar Range (Scott et al. Citation2011), southwest of the Hohonu Dike Swarm and is a lamprophyric dike dated to ∼ 69 Ma (van der Meer et al. Citation2013) (B). WDS samples are characterised as camptonitic and carry amphibole megacrysts, as well as spinel-facies peridotite xenoliths (Tulloch and Nathan Citation1990; van der Meer et al. Citation2013; Scott et al. Citation2016b) ().
Figure 2. A, Field photo of light-grey MOA-16 lamprophyre sample from the WDS, first presented in van der Meer et al. (Citation2013). Abundant visible mantle xenoliths (light brown) and amphibole megacrysts (black). B, Field photo from the Fish River locality in the ADS with a 15 cm long chisel for scale. The photo shows a grey-green ultramafic lamprophyre with abundant amphibole megacrysts and mantle xenoliths.
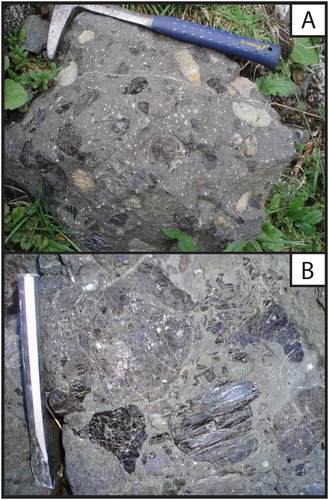
Alpine Dike Swarm (ADS)
Amphibole megacrysts were obtained from three samples belonging to a lamprophyre + phonolite + carbonatite dike suite in the ADS. Samples FIS-4, MAC-1 and LWA-12 originate from lamprophyres from Fish River, MacFarlane River and Lake Wanaka, respectively (B). They were chosen because they carry both megacrystic amphiboles and spinel-facies peridotite xenoliths (Scott et al. Citation2014b; Scott et al. Citation2016a) (B).
Results
General petrography
Investigated WDS camptonites comprise a groundmass consisting of sub-millimetre sized subhedral to euhedral brown amphibole microphenocrysts together with abundant feldspars, minor opaque phases, apatite and secondary epidote ± chlorite (). Larger phases consist of orange to brown pleochroic megacrystic amphiboles, as well as occasional <5 mm sized anhedral clinopyroxene and up to 2 mm sized epidote ± carbonate ocelli (). Investigated samples contain variable amounts of centimetre-sized amphibole megacrysts, which are represented by heavily fractured subhedral to anhedral grains with irregular edges (). When present, rims are <0.5 mm thin and show similar pleochroic colours to groundmass microphenocrysts. Most fractures in megacrystic amphiboles contain groundmass material (). Additionally, amphibole megacrysts show indications of chemical re-equilibration along fractures where pleochroic colours match those seen in rims and microphenocrysts (). Smaller 1–5 mm-sized anhedral amphibole grains have similar characteristics to the associated megacrysts and are therefore interpreted to represent fragmented megacrysts. Additionally, these amphibole fragments have rims grown uniformly around anhedral cores with no systematic differences in dark/light brown pleochroism between cores and rims.
Figure 3. Representative thin section of WDS samples (specifically from sample KST-2). The photo shows two amphibole megacrysts (Amph), as well as epidote ± carbonate ocelli (Oc) and rounded clinopyroxene grains (Cpx) in an amphibole + plagioclase + epidote + chlorite + opaque phase groundmass. Anhedral >1 mm sized zoned amphibole grains are interpreted to represent megacryst fragments.
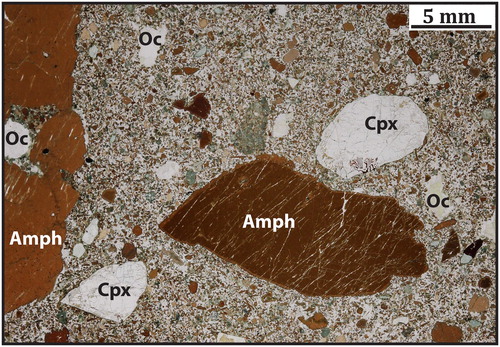
The petrography of the ADS camptonites has been provided in detail by Cooper (Citation2020), who describes them as consisting of a feldspar-rich + clinopyroxene + kaersutite + biotite groundmass hosting euhedral phenocrysts of olivine, kaersutite, clinopyroxene and biotite. Kaersutite phenocrysts show both normal and reverse zoning patterns with Mg# (Mg# = 100·Mg/(Mg + Fe)) ranging from 40 to 67 (Cooper Citation2020).
Major elements
Amphibole megacrysts and groundmass microphenocryst amphiboles in all samples are classified as predominantly ferri-kaersutite and minor magnesio-hastingsite according to the classification scheme of Hawthorne et al. (Citation2012) using calculations from Locock (Citation2014) (). A compilation of major element data of 177 amphibole grains is provided at 10.6084/m9.figshare.12057843. Investigated amphiboles show compositions rich in Al2O3 (11–15 wt.%), CaO (10–13 wt.%), TiO2 (3–7 wt.%) together with particularly variable FeOtot (9–18 wt.%) and MgO (8–14 wt.%) and minor K2O (1–2 wt.%) and Na2O3 (2–4 wt%) concentrations. High variability in FeOtot and MgO contents is reflected by amphibole megacryst core Mg# compositions (). While amphibole megacrysts in some samples show high Mg# with little to no internal variability [i.e. WDS samples HOH-1 (68–69) and MOA-16 (70); ADS samples FIS-4 (69–70), MAC-1 (71–72) and LWA-12 (68)], amphibole megacryst cores in WDS sample OU79943 show moderately variable Mg# (62–69), and WDS samples QH12 and KST-2 exhibit highly variable amphibole megacryst core Mg# compositions (48–66 and 47–66, respectively) (). Investigated amphibole megacryst rim compositions in WDS samples are identical to or approaching host rock microphenocryst compositions (with Mg# = 61–72). Amphibole megacryst rim compositions show higher Mg# compared to core compositions in WDS samples QH12 and KST-2, whereas the opposite is observed in sample MOA-16.
Figure 4. Mg# vs. Ti (apfu) diagram showing studied amphibole megacryst cores, rims and microphenocrysts following the classification after Hawthorne et al. (Citation2012). Ti (apfu) determined using calculations from Locock (Citation2014). Mega-C = megacryst core analysis; Mega-R = megacryst rim analysis; Micro = microphenocryst analysis. Black symbols represent WDS samples and red symbols represent ADS samples.
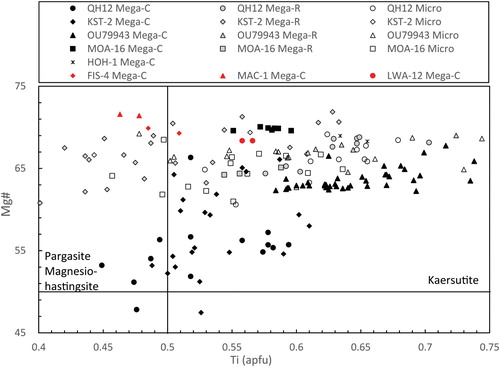
Trace elements
Trace element data for 83 amphibole grains are available at 10.6084/m9.figshare.12057843. Primitive mantle normalised incompatible trace element patterns of amphibole megacrysts show positive anomalies in Ba, Nb, Ta and Ti, as well as distinct negative anomalies in Th, U, Pb, Zr and Hf (A). Megacrystic amphiboles have similar convex-upward chondrite normalised REE patterns with a maximum at Nd [Nd(n) = 38–111 (avg. = 69)], enriched LREE [Nd(n)/Lu(n) = 5–22 (avg. = 9)], depleted HREE [Gd(n)/Lu(n)=3–10 (avg. = 5)] and no Eu anomaly (B). Host rock microphenocrysts are over twice as enriched in all REE [(totREE(n) = 600–1050 (avg. = 700))] relative to megacrysts [(totREE(n) = 180–480 (avg. = 310))] (B), while megacryst rims show intermediate compositions approaching microphenocryst compositions (B). It is evident that ADS and WDS megacrysts share comparable trace element signatures (C). However, WDS megacryst cores are more enriched in MREE and particularly HREE (avg. Ndn/Lun = 7) compared to ADS megacrysts, resulting in steeper LREE-HREE slopes in ADS samples (avg. Ndn/Lun = 16) (D).
Figure 5. A, Trace element analyses of amphibole cores, rims and microphenocrysts normalised to primitive mantle (McDonough and Sun Citation1995). B, REE analyses of amphibole cores, rims and microphenocrysts normalised to chondrite (McDonough and Sun Citation1995). The solid line in each field shows the averaged composition. C, Trace element analyses of WDS and ADS megacrystic amphibole cores compared to WDS and ADS lamprophyre compositions normalised to primitive mantle (McDonough and Sun Citation1995). D, REE analyses of WDS and ADS megacrystic amphibole cores compared to WDS and ADS lamprophyre compositions normalised to primitive mantle (McDonough and Sun Citation1995). Lamprophyre trace element and REE data are from Timm et al. (Citation2010), van der Meer et al. (Citation2016, Citation2017) and Cooper (Citation2020).
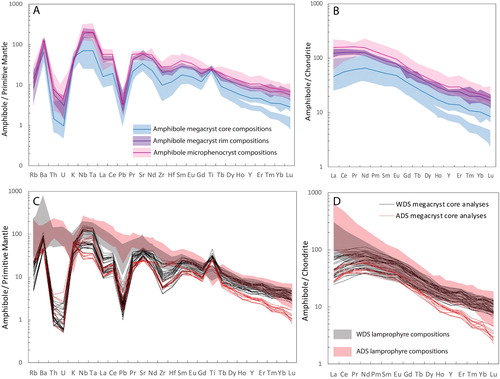
Radiogenic isotopes
To compare source characteristics of amphibole megacrysts occurring in two separate geological domains in southern New Zealand, amphibole megacrysts were separated and measured for Sr-Nd-Hf-Pb isotopes (). shows age-corrected Sr-Nd-Hf-Pb isotopic data of amphibole megacrysts related to published data from relevant WP and EP lithologies as well as BSE, HIMU and MORB compositions. The effect of age-corrections is relatively minor compared to the variations observed in the data set. Amphibole megacrysts from the WDS show limited variation in Sr-Nd-Hf isotopic signatures with 87Sr/86Sr(88-69Ma) = 0.702754–0.703371, 143Nd/144Nd(88-69Ma) = 0.512748–0.512807 (εNd(88-69Ma) = +3.9 to +5.5) and 176Hf/177Hf(88-69Ma) = 0.282869–0.282950 (εHf(88-69Ma) = +5.0 to +8.2). These values overlap with more constrained ADS amphibole megacryst signatures, which have 87Sr/86Sr(25-23Ma) = 0.702842–0.702991, 143Nd/144Nd(25-23Ma) = 0.512808–0.512828 (εNd(25-23Ma) = +3.9 to +4.3) and 176Hf/177Hf(25-23Ma) = 0.282899–0.282953 (εHf(25-23Ma) = +5.0 to +6.9) (A,B). With regards to Pb isotopes, WDS amphibole megacrysts show less radiogenic signatures with 206Pb/204Pb(88-69Ma) = 18.909–19.400, 207Pb/204Pb(88-69Ma) = 15.642–15.657 and 208Pb/204Pb(88-69Ma) = 38.824–39.290 compared to ADS amphibole megacrysts, which also show a larger spread in Pb signatures with 206Pb/204Pb(25-23Ma) = 19.494–20.526, 207Pb/204Pb(25-23Ma) = 15.661–15.678 and 208Pb/204Pb(25-23Ma) = 39.272–40.271 (C,D). Megacrystic amphiboles plot within the Sr-Nd-Hf-Pb general Zealandia intraplate magmatism arrays (); however, most WDS amphibole megacrysts show slightly less radiogenic Sr signatures compared to WDS intraplate volcanism (A). ADS amphibole megacrysts, as well as intraplate magmatism, generally show similar Sr-Nd-Pb signatures compared to peridotite xenoliths hosted by intraplate magmatism (). WDS amphibole megacrysts partly overlap with peridotite xenoliths in Sr-Nd signatures and only overlap with Pb signatures of the Anita Peridotite (Czertowicz et al. Citation2016) (). Hf signatures in amphibole megacrysts are generally less radiogenic than SCLM xenoliths, except for xenoliths collected in the Burke River in the ADS, which show comparable Hf signatures to both amphibole megacrysts and Zealandia intraplate magmatism (Scott et al. Citation2014b; Scott et al. Citation2016a) (B).
Figure 6. Age-corrected isotopic signatures of amphibole megacryst compared to published data from relevant Zealandia lithologies [i.e. EP Cenozoic intraplate volcanism (Price Citation2003; Hoernle et al. Citation2006; Sprung et al. Citation2007; Timm et al. Citation2009, Citation2010; Scanlan et al. Citation2020; Scott et al. Citation2020); EP Cretaceous intraplate volcanism (McCoy-West et al. Citation2010; Hoernle et al. Citation2020); EP peridotite xenoliths (Scott et al. Citation2014b; Scott et al. Citation2016a); WP Cretaceous intraplate volcanism (Timm et al. Citation2010; van der Meer et al. Citation2016, Citation2017; Hoernle et al. Citation2020); WP exhumed Anita Peridotite (Czertowicz et al. Citation2016)]. Amphibole megacryst signatures are age-corrected to the ages reported in . Literature data are age-corrected to ages reported in respective publications, except for Pb peridotite clinopyroxene data, for which only measured isotopic ratios are available. Approximate Tasman, MORB, HIMU and MORB fields are age-corrected to 60 Ma and are from Mortimer et al. (Citation2012), Stracke et al. (Citation2005) and Chauvel et al. (Citation2008), respectively. Present-day NHRL is from Hart (Citation1984) and the εHF vs. εNd mantle array is from Vervoort and Blichert-Toft (Citation1999). Estimated reproducibility is shown on each figure.
![Figure 6. Age-corrected isotopic signatures of amphibole megacryst compared to published data from relevant Zealandia lithologies [i.e. EP Cenozoic intraplate volcanism (Price Citation2003; Hoernle et al. Citation2006; Sprung et al. Citation2007; Timm et al. Citation2009, Citation2010; Scanlan et al. Citation2020; Scott et al. Citation2020); EP Cretaceous intraplate volcanism (McCoy-West et al. Citation2010; Hoernle et al. Citation2020); EP peridotite xenoliths (Scott et al. Citation2014b; Scott et al. Citation2016a); WP Cretaceous intraplate volcanism (Timm et al. Citation2010; van der Meer et al. Citation2016, Citation2017; Hoernle et al. Citation2020); WP exhumed Anita Peridotite (Czertowicz et al. Citation2016)]. Amphibole megacryst signatures are age-corrected to the ages reported in Table 1. Literature data are age-corrected to ages reported in respective publications, except for Pb peridotite clinopyroxene data, for which only measured isotopic ratios are available. Approximate Tasman, MORB, HIMU and MORB fields are age-corrected to 60 Ma and are from Mortimer et al. (Citation2012), Stracke et al. (Citation2005) and Chauvel et al. (Citation2008), respectively. Present-day NHRL is from Hart (Citation1984) and the εHF vs. εNd mantle array is from Vervoort and Blichert-Toft (Citation1999). Estimated reproducibility is shown on each figure.](/cms/asset/b3f078f6-4e0b-40d3-a9c4-15b95c23927f/tnzg_a_1801771_f0006_c.jpg)
Table 1. Radiogenic isotope results of Zealandia amphibole megacrysts.
Discussion
The petrogenesis of amphibole megacrysts
Kaersutitic amphibole megacrysts are a common occurrence in lamprophyric magmas worldwide. There is no single consensus on their origin, which has led to three main theories being proposed: (1) a cognate relationship between amphiboles and host rocks, with amphiboles representing high-pressure phenocrysts formed during magma ascent or in deep-seated magma chambers prior to eruption (e.g. Binns Citation1969; Merrill and Wyllie Citation1975; Irving and Frey Citation1984; Mayer et al. Citation2014; Ulrych et al. Citation2018); (2) a non-cognate relationship between amphiboles and host rocks, with amphiboles representing xenocrystic fragments from amphibole-rich veins in the upper mantle (e.g. Wilshire and Trask Citation1971; Best Citation1974; Boettcher and O’Neil Citation1980; Ben Othman et al. Citation1990; Shaw and Eyzaguirre Citation2000; Dobosi et al. Citation2003); and (3) amphibole megacrysts representing antecrysts that crystallised in deep and complex magma-plumbing systems (e.g. Ubide et al. Citation2014).
Mantle amphiboles often occur as major constituents in up to 30 cm thick hydrous hornblendite (± phlogopite) and/or amphibole-clinopyroxenite veins cross-cutting lherzolite xenoliths (e.g. Roden et al. Citation1984; Dautria et al. Citation1987; Nielson et al. Citation1993; Ionov and Hofmann Citation1995; Vaselli et al. Citation1995; Witt-Eickschen et al. Citation1998; Downes Citation2001; Dobosi et al. Citation2003; Witt-Eickschen Citation2003; Coltorti et al. Citation2004). Such xenoliths are interpreted to represent fragments of hornblendite and amphibole-pyroxenite veins from metasomatically enriched SCLM regions, analogous to hydrous veins reported in situ in the Lherz ultramafic massif in France (e.g. Zanetti et al. Citation1996; Bodinier Citation2004). Interestingly, occurrences of amphibole and phlogopite veins have also been reported as rare components to peridotite xenoliths entrained by Zealandia intraplate magmas (Dickey Citation1968; Tulloch and Nathan Citation1990; Scott et al. Citation2014b), as well as occurring in in situ hornblendite and orthopyroxene-amphibole veins in the Anita Peridotite ultramafic massif in Fiordland (Czertowicz et al. Citation2016). Moreover, the studied ADS and WDS amphibole megacrysts share strikingly similar trace element and REE patterns to upper mantle vein amphiboles from several studies (), indicating similar formation processes, which warrants a more detailed look into megacryst – host melt relationships. To that end, we investigate available isotopic data from three WDS host rock – megacrystic amphibole pairs (QH12, KST-2 and OU79943), as well as available major and trace element data in amphibole microphenocrysts and megacrysts from four WDS samples (QH12, KST-2, OU79943 and MOA-16).
Figure 7. A, Trace element analyses of WDS and ADS megacrystic amphibole cores compared to SCLM vein amphiboles from the literature. Data are normalised to primitive mantle (McDonough and Sun Citation1995). B, REE analyses of WDS and ADS megacrystic amphibole cores compared to SCLM vein amphiboles from the literature. Data are normalised to chondrite (McDonough and Sun Citation1995). Black lines with solid markers: amphibole in hydrous veins found within worldwide lherzolite xenoliths. Black lines with empty markers: amphibole from in situ amphibole-rich veins in the Lherz ultramafic massif, France. Purple line with empty marker represents amphibole composition from a hornblendite vein found in situ within the Anita Peridotite.
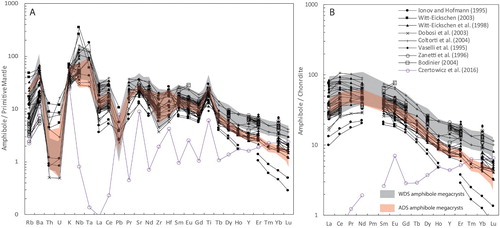
Isotope systematics between WDS amphibole megacrysts and host rocks
Overlapping Sr-Nd-Hf-Pb signatures in both ADS and WDS amphibole megacrysts and intraplate magmatism arrays from Zealandia demonstrate that amphibole megacrysts are closely linked to the Zealandia intraplate magmatic system (). In detail, phenocrysts should theoretically record the same isotopic signatures as the host melts in which they crystallised. However, melts rarely remain closed systems during ascent through the mantle and/or crust, where processes such as crustal contamination and element remobilisation are common. Thus, primary isotopic signatures of host melts are often modified during ascent. In this section, age-corrected isotopic signatures of three available amphibole megacryst – host rock pairs from the WDS are investigated in order to determine if amphibole megacrysts are in equilibrium with their respective host melts.
shows age-corrected Sr-Nd-Hf-Pb isotopic signatures for three WDS amphibole megacryst – host rock pairs relative to ADS megacryst amphiboles, as well as fields depicting both age-corrected Cretaceous and Cenozoic lamprophyric intraplate magmatism arrays. Amphibole megacrysts plot within the respective intraplate arrays, consistent with megacrysts being part of the Zealandia intraplate magmatic system. Host rocks show variable but consistently more radiogenic 86Sr/87Sr(i) compared to associated amphibole megacrysts (especially so in sample OU79943) (A). Enriched whole rock Sr signatures are interpreted as being a consequence of varying degrees of secondary hydrothermal alteration in WDS lamprophyres, as proposed by van der Meer et al. (Citation2016, Citation2017) and consistent with petrographic evidence for secondary calcite. The OU79943 amphibole megacryst shows small offsets in Hf and Pb, as well as comparable Nd signatures relative to its host rock. As the OU79943 host rock is rather evolved and may have been subject to hydrothermal alteration ± crustal contamination (van der Meer et al. Citation2016, Citation2017), such slight discrepancies between host rock and amphibole megacrysts isotopic signatures may be interpreted to be secondary. Thus, the OU79943 amphibole megacryst may either have crystallised as a phenocryst in a host melt, which subsequently experienced secondary alteration, or the OU79943 megacryst could be xenocrystic but derived from a source with similar isotopic signatures. The QH12 megacrystic amphibole has comparable Hf and Nd isotopic signatures while showing substantially more radiogenic Pb signatures relative to its host rock (C). Such Pb discrepancy between host rock and megacryst suggests that the analysed QH12 amphibole megacryst is not cognate to its host melt. In a similar manner, the KST-2 amphibole megacryst shows more evolved Nd signatures and slightly different Pb and Hf signatures compared to its host rock, also indicating that it did not crystallise from its host melt. Thus, based on host melt – amphibole megacryst isotopic signatures, at least two WDS amphibole megacrysts (KST-2 and QH12) cannot have crystallised from their host melts as reflected in the whole rock dike composition, and must have been collected by their host melts during ascent and/or storage in the lithosphere.
Figure 8. Age-corrected amphibole megacryst – host rock isotopic relationships using the ages indicated in . A, 143Nd/144Nd(i) vs. 86Sr/87Sr(i) diagram showing 143Nd/144Nd(i) discrepancy between KST-2 amphibole and host rock isotopic signatures as well as variable 86Sr/87Sr(i) discrepancies between WDS amphiboles and host rock isotopic signatures. B, εHf(i) vs. εNd(i) diagram showing εHf(i) discrepancies between host rock and amphibole signatures in samples KST-2 and OU79943, as well as a large εNd(i) discrepancy between host rock and amphibole signatures in sample KST-2. C, 208Pb/204Pb(i) vs. 206Pb/204Pb(i) diagram showing a major Pb isotopic discrepancy between QH12 amphibole megacryst and host rock signatures, as well as discrete 206Pb/204Pb(i) isotopic discrepancies between host rock and amphibole signatures in samples KST-2 and OU79943. WP Cretaceous intraplate volcanism data from van der Meer et al. (Citation2016, Citation2017) and Hoernle et al. (Citation2020). EP Cenozoic intraplate volcanism data from Price (Citation2003), Hoernle et al. (Citation2006), Sprung et al. (Citation2007), Timm et al. (Citation2009, Citation2010), Scanlan et al. (Citation2020) and Scott et al. (Citation2020).
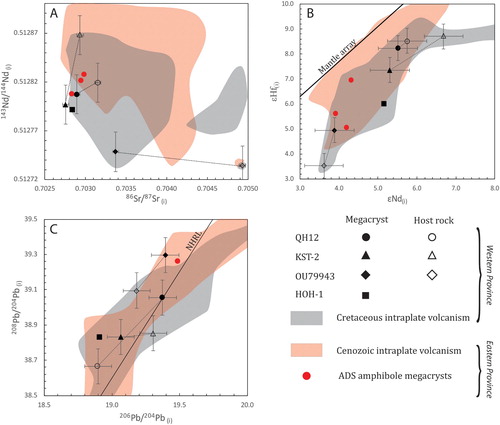
Geochemical systematics between WDS amphibole megacrysts and host rocks
Mg# variability in amphibole megacrysts. If amphibole megacrysts crystallised as phenocrysts in lamprophyric host melts, one would expect early-formed phenocrysts to have high Mg# and that subsequently formed rims and microphenocrysts to show lower Mg#, following a normal melt evolution. Amphibole megacrysts from sample MOA-16 show high Mg# (70) cores and low Mg# (61–68) rims and microphenocrysts (), indicating that they may likely have crystallised as earlier phenocrysts within the host melt. Contrastingly, sample OU79943 exhibits a large population of megacryst cores with slightly lower Mg# (62–64) compared to rim and microphenocryst Mg# (65–69) (). Moreover, amphibole megacryst cores show anomalously large variation towards low Mg# within WDS samples QH12 and KST-2 (i.e. Mg# 47–66) (), as well as showing substantially lower Mg# in amphibole megacryst cores relative to both rims and microphenocrysts (). Host lamprophyres typically evolve to lower MgO with increasing SiO2. While subtle but consistently higher Mg# in OU79943 rims and microphenocryst can be interpreted to be caused by a single injection of a more primitive melt after megacryst crystallisation, neither the chemical evolution of QH12 and KST-2 host melts nor the injection of a primitive melt alone can account for the large Mg# variability in these amphiboles. Thus, consistent with the previously described isotopic discrepancies in megacryst – host rock signatures, low-Mg# QH12 and KST-2 amphibole megacryst cores did not crystallise in their respective host melts.
Trace element systematics between megacryst core, rims and microphenocrysts. Similar major and trace element characteristics of megacryst amphibole rims and microphenocrysts ( and A,B) indicate that megacryst rims most likely grew concurrently with amphibole microphenocrysts in associated host rocks. This is supported by fractured megacryst cores showing signs of chemical reequilibration along fractures and showing matching pleochroism with megacryst rims and microphenocrysts (). This suggests that cores were fractured before concurrent crystallisation of both microphenocrysts and megacryst rims. The absence of Eu anomalies in all amphiboles suggests that groundmass feldspar was the last crystallising phase and that no feldspars crystallised in tandem with either amphibole megacrysts or microphenocrysts (A). Investigated megacryst cores show similar but lower trace element and REE patterns compared to rims and microphenocrysts (A,B). Given the incompatibility of these elements in both amphibole and host melts, early-formed phenocrysts should have lower trace elements and REE abundances compared to microphenocrysts and megacryst rims, which represent later crystallising phases. Thus, the general trace element systematics between amphibole megacryst cores, rims and microphenocrysts seem to depict a cognate relationship between amphibole megacrysts and host melts (). However, low-Mg# and host rock – megacrystic amphibole isotopic discrepancies in QH12 and KST-2 are inconsistent with a cognate model.
Based on the observations above, host rocks and amphibole trace element patterns are used to further constrain mineral-melt relationships. For this purpose, we calculate theoretical trace element compositions of amphiboles that are in equilibrium with host melt compositions at different degrees of crystallisation using the equation for fractional crystallisation [CAmph = CHost·DAmph·FDAmph−1 (where CAmph is the concentration of an element in an amphibole; CHost is the concentration of that element in the host melt for a remaining melt fraction F; and DAmph is the partition coefficient of a given element in amphibole)]. Any potential pyroxene or olivine is omitted from the models as these are assumed to have minimal influence due to low partition coefficients of the trace elements of interest. Additionally, petrographic observations revealed that olivine is absent and pyroxenes are scarce in these samples. Feldspars are assumed to form after amphibole crystallisation based on the absence of Eu anomalies in all investigated amphiboles. Relevant published amphibole partition coefficients were collated from Adam and Green (Citation2006) (for Rb, Ba, Th, U, Nb, Ta, La, Ce, Pb, Sr, Nd, Sm, Ho, Y, Yb and Lu) and Fulmer et al. (Citation2010) (for Pr, Eu Gd, Dy and Er).
Modelled amphibole trace element patterns approaching groundmass microphenocryst compositions constrain the timing of crystallisation to the last ∼20% of remaining melt of host magmas (A,B). Calculated amphibole compositions in equilibrium with host rocks at different amounts of remaining melt (25%–80%) generally cover the range of megacrystic amphibole core compositions in all three available host rock – amphibole pairs (). Thus, these models show that even though low-Mg# QH12, KST-2 and potentially OU79943 amphiboles did not form in their respective host rocks, they must have crystallised in melts with similar trace element compositions to investigated host lamprophyres.
Figure 9. Modelling of theoretical fractionated amphibole trace element compositions compared to amphibole compositional ranges within each sample. Data are normalised to primitive mantle (McDonough and Sun Citation1995). A, Modelling of fractionated amphibole compositions calculated using QH12 host rock chemistry. B, Modelling of fractionated amphibole compositions calculated using OU79943 host rock chemistry. C, Modelling of fractionated amphibole compositions calculated using KST-2 host rock chemistry. Bulk rock data from van der Meer et al. (Citation2016). Ti and K are omitted here due to large variations in published partition coefficients.
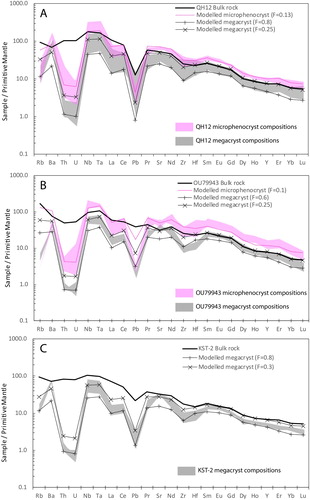
Evidence from host lamprophyre geochemical evolution. Bulk rock compositions of intraplate lamprophyres from both WDS and ADS show evidence of amphibole fractional crystallisation with decreasing Dy/Yb vs. increasing SiO2 (). This is further supported by Cooper (Citation2020) who argued for fractionation of MREE-rich kaersutite (+ olivine, clinopyroxene, and titano-magnetite) from ADS melts in order to produce the compositional evolutionary trends between lamprophyres and phonolites of the ADS. Moreover, fractionation of such phases in lamprophyres would also account for the occurrence of anhedral clinopyroxene grains found within WDS host lamprophyres (), which may represent remnants of fractionated phases. Considering that phonolites also occur in the WDS (Waight et al. Citation1998a) and that both ADS and WDS lamprophyre-phonolite arrays show decreasing Dy/Yb vs SiO2 (), it is tempting to envision the involvement of kaersutite fractionation in magma reservoirs to account for the lamprophyre-phonolite compositional range in both regions, in which investigated amphibole megacrysts represent remnants of such fractionated cumulate phases. WDS lamprophyres show a wide range of Mg# (37–68; van der Meer et al. Citation2017), which can account for the crystallisation of WDS megacrystic amphiboles with variable Mg# (47–70). Additionally, WDS Sr-Nd-Hf-Pb isotopic arrays overlap isotopic discrepancies between QH12 and KST-2 host rock and megacrystic amphiboles (). Thus, indicating that amphibole megacrysts likely crystallised from lamprophyric melts related to the WDS.
Figure 10. Lamprophyre to phonolite raw Dy/Yb vs. SiO2 arrays in the WDS and ADS. Grey arrow shows the negative correlation between Dy/Yb and SiO2, indicative of amphibole fractionation. WDS bulk rock data is from van der Meer et al. (Citation2016, Citation2017) and ADS bulk rock data from Timm et al. (Citation2010) and Cooper (Citation2020).
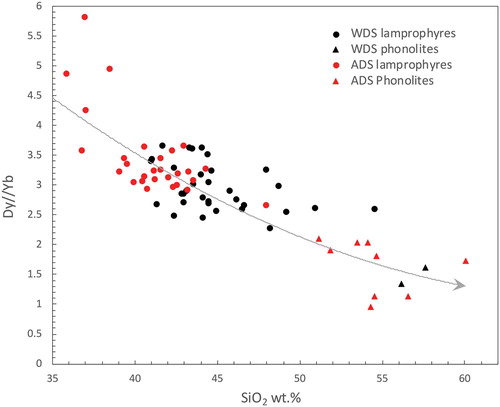
Amphibole thermobarometry
Tentative geothermobarometric calculations based on the partitioning of Al in amphibole were performed on megacryst cores using equations from Putirka (Citation2016) coupled with the amphibole hygrometer from Ridolfi and Renzulli (Citation2012), with the caveat that it is unclear how applicable these thermobarometers are to lamprophyric systems. Pressure estimates on individual amphiboles can yield uncertainties of ± 4 kbar (Putirka Citation2016), which are not trivial. Thus, we attempt to mitigate potential uncertainties by averaging pressure estimates of several amphiboles from each sample as recommended by Putirka (Citation2016). Results suggest that ADS amphibole megacrysts crystallised from magmas having 4–6.1 wt.% H2O at pressure conditions of 8.3–9.6 kbar and temperatures of 1031–1053°C, whereas WDS amphibole megacrysts crystallised in pressure conditions of 7.5–8.6 kbar and temperatures of 1024–1062°C in magmas containing 4.7–5.6 wt.% H2O. These results are consistent with the experimental study of Pilet et al. (Citation2010), which demonstrated that kaersutite in basanitic melts with 5–6 wt.% H2O is stable at conditions under 1130°C and 15 kbar. By following a conventional conversion factor of 1 kbar ∼ 3.7 km of crust, ADS and WDS amphibole megacryst are estimated to have crystallised around 31–36 km and 28–32 km depth, respectively. Based on present-day estimations of 35–48 km Moho depth (Salmon et al. Citation2013) and assumed Moho depths of ∼ 37 km during the Cenozoic in the ADS region (e.g. Scott et al. Citation2014b), it can be inferred that ADS amphibole megacrysts may have crystallised at depths equivalent to the lowermost crust. The crystallisation setting of WDS amphibole megacrysts is harder to constrain as Moho depths during the Late Cretaceous have not been explicitly studied. However, assuming an averaged extensional setting with a ∼ 30 km thick crust (Christensen and Mooney Citation1995), the crystallisation of WDS megacrystic amphiboles may have occurred close to the crust/mantle boundary.
Implications for amphibole megacryst petrogenesis in Zealandia
Investigating amphibole megacryst geochemical and isotopic characteristics alone is not adequate to fully determine their petrogenesis. Evidently, detailed investigations of isotopic and geochemical relationships in megacryst – host rock pairs are required to properly distinguish phenocrysts from xenocrysts. Even though we currently do not possess a full geochemical dataset of investigated amphibole megacrysts and their respective host rocks, the strong similarities between both amphibole megacrysts and host rocks from the WDS and ADS infer that similar processes may act in both regions. Even though the presented geochemical evidence suggests that amphibole megacrysts crystallised from lamprophyric melts, we cannot ignore that megacrystic amphiboles share remarkable trace element and REE compositional similarities to SCLM vein amphibole compositions (). As such, two models for the petrogenesis of amphibole megacrysts are considered.
The lamprophyre model. Considering tentative geothermobarometric estimations, megacrystic amphibole may have crystallised at lower crustal depths. Thus, low-Mg# amphibole megacrysts likely fractionated from low-Mg# lamprophyric melts in lower crustal magma-plumbing systems. Repeated melting of an isotopically moderately heterogeneous source region initiated the injection of more primitive lamprophyric host melts and promoted repeated magma mingling in lower crustal settings, resulting in both the variable Mg# and isotopic spread in megacryst cores as well as the high Mg# in megacryst rims and microphenocrysts. Alternatively, QH12 and KST-2 amphibole megacrysts may have fractionated in magma reservoirs from precursor low-Mg# lamprophyric melts that remained emplaced in deeper settings than the investigated WDS lamprophyric host rocks. Subsequent ascent of WDS host melts may have reactivated such reservoirs and collected xenocrystic cumulate amphibole megacrysts. Models involving different generations of lamprophyric melts in the WDS are further supported by the fact that the KST-2 lamprophyre host rock is part of a composite dike showing diffuse contacts with an associated amphibole megacryst-bearing phonolitic unit (Waight et al. Citation1998a; van der Meer et al. Citation2013), indicating the simultaneous presence of magmas of contrasting compositions and indicating that some degree of magma mixing and/or magma reservoir reactivation could have occurred in the WDS. This is also consistent with the presence of oscillatory zoning and sieve textures in plagioclase phenocrysts in doleritic members of the Hohonu Dike Swarm (Waight Citation1995)
The vein model. Low-Mg# megacrystic amphiboles could represent xenocrysts sampled from a veined SCLM. Such amphiboles would have crystallised in precursor hydrous melts, which were emplaced as metasomatic veins in the SCLM. Subsequent infiltration of host lamprophyric melts used the rheologically weaker veins as conduits and collected both fractured megacrystic amphiboles as well as peridotite xenoliths during their ascent through the SCLM. Recent studies have proposed that amphibole-rich veins in the SCLM may represent important components in the sources for lamprophyric magmatism in Zealandia (Panter Citation2006; Sprung et al. Citation2007; Scott et al. Citation2016a; van der Meer et al. Citation2017) and it is tempting to link the investigated amphibole megacrysts to such SCLM lithologies. In such a model, lamprophyre compositions would inherit similar trace element and REE patterns to amphibole compositions, as amphibole would be the main contributor of such elements. This is partly the case (), but an amphibole-rich SCLM source also requires an additional Th, and U-rich component to account for high concentrations in Zealandia lamprophyres. In a vein model, isotopic discrepancies between host rock – megacryst pairs would be expected if lamprophyres were derived from a heterogeneously metasomatised and veined SCLM source. Namely, WDS and ADS lamprophyres would record mixed isotopic signatures of heterogeneous melting sources, in which amphibole xenocrysts record primary isotopic signatures of individual source components. However, reconciling a vein model with the presented data raises some issues. Firstly, despite showing comparable trace element and REE patterns to megacrystic amphiboles, published vein amphiboles show exclusively high Mg# (70–90) (e.g. Ionov and Hofmann Citation1995; Zanetti et al. Citation1996; Coltorti et al. Citation2004). A single hornblendite vein in the Anita Peridotite hosts low-Mg# (52) amphibole (Czertowicz et al. Citation2016). However, the amphibole also displays heavily contrasting trace element and REE patterns compared to the studied megacrysts, which argues for disparate formation mechanisms (). One could argue for Fe-rich basanitic melts to have formed amphibole-rich metasomes with low-Mg# in order to accommodate the vein model (Frost Citation2006). However, such arguments remain speculative. Additionally, albeit being fairly uncertain, geobarometric estimations may place the formation depth of amphibole megacrysts in the lowermost crust instead of the SCLM.
In both models, amphibole megacryst cores that display higher than or comparable Mg# compared to microphenocryst compositions may represent cognate phenocrysts within host rocks. Additionally, amphibole megacrysts rims and host rock microphenocrysts likely crystallised during final emplacement of investigated WDS lamprophyres. The presented data cannot decisively constrain one single petrogenetic model for amphibole megacryst formation, but we currently favour the lamprophyre model as it more elegantly explains the data.
Comparison of WDS and ADS amphibole megacrysts
Both WDS and ADS megacrystic amphiboles fall into the classification of the magnesio-hastingsite – kaersutite series. Moreover, amphibole megacrysts from both regions show remarkably similar trace element and REE patterns (C,D) as well as overlapping restricted ranges of Sr-Nd-Hf isotopic signatures (i.e. 87Sr/86Sr(i) = 0.702754–0.703371, εNd(i) = +3.9 to +5.5 and εHf(i)= +5.0 to +8.2) (), which suggests that they formed under comparable conditions and from similar sources – despite an age difference of over 50 Ma. Such compositional similarities may simply be related to the megacrysts being hosted by compositionally and isotopically similar host lamprophyres in both regions (C,D and ). However, given the different settings in which host lamprophyre dike swarms were emplaced as well as being both spatially and temporally decoupled, such amphibole megacrysts are a peculiarity worth investigating.
Despite similarities, two key differences between ADS and WDS amphibole megacrysts are notable. The first difference is that amphibole megacrysts from the ADS show lower HREE concentrations relative to WDS megacrysts, which is a trait shared by ultramafic lamprophyres of the ADS (Cooper Citation2020). The resulting steeper LREE/HREE pattern in ADS megacrysts could be associated to HREE retention by garnet during partial melting in ADS source regions, which lowered the HREE budget of ADS host lamprophyres (Cooper Citation2020). It is noteworthy that peridotite xenoliths entrained by intraplate alkaline magmatism in Zealandia exclusively represent spinel-facies peridotites (Scott et al. Citation2020, and references therein). Thus, indicating either that ADS lamprophyres were derived in part from melting of garnet-facies peridotite and only incorporated xenoliths from shallower regions, or that steeper LREE/HREE patterns are caused by other processes. For example, such patterns can also be derived if host melts were (at least partially) sourced by partial melting of a depleted SCLM, which subsequently experienced heterogeneous enrichment of LREE through carbonatitic metasomatism (e.g. Scott et al. Citation2014a, Citation2014b; McCoy-West et al. Citation2015; Scott et al. Citation2016a, Citation2016b). The second notable difference is that compared to WDS amphibole megacrysts, ADS amphiboles show more radiogenic 206Pb/204Pb(i) and 208Pb/204Pb(i) signatures as well as a larger spread in these isotopic ratios (C,D). Radiogenic and variable HIMU-like 206Pb/204Pb(i) and 208Pb/204Pb(i) signatures have been reported in both Zealandia intraplate magmatic rocks (Hoernle et al. Citation2006; Panter Citation2006; Sprung et al. Citation2007; Timm et al. Citation2009, Citation2010; van der Meer et al. Citation2016, Citation2017; Scott et al. Citation2020) and in SCLM peridotite xenoliths entrained by intraplate magmatism (Scott et al. Citation2014a, Citation2014b; McCoy-West et al. Citation2016; Scott et al. Citation2016a) (C,D). Thus, pronounced HIMU-like Pb signatures in ADS megacrystic amphiboles may be inherited by a local enrichment of 206Pb/204Pb(i) and 208Pb/204Pb(i) in the source regions. As the main differences between WDS and ADS megacrystic amphiboles are derived by processes driven by regional differences, the next section will investigate isotopic signatures of amphibole megacrysts in relation to intraplate magmatism in Zealandia in order to place constrains on source characteristics and evolution.
Amphibole megacryst isotopic signatures in a Zealandia setting
Even though amphibole megacrysts share comparable compositions and isotopic signatures, they each belong to two separate instances of Zealandia intraplate magmatism emplaced in different regional settings and at different times. Both WDS and ADS magmatism belong to widespread alkaline intraplate magmatism that has been emplaced throughout Zealandia since the Late Cretaceous, with HIMU-like Sr-Nd-Hf-Pb signatures with 87Sr/86Sr(i) ∼ 0.703–0.704, εNd(i) = +2 to +8, εHf(i) = +2 to +10, 206Pb/204Pb(i) = 18.5–20.7 and 208Pb/204Pb(i) = 38.5–41 (Price Citation2003; Hoernle et al. Citation2006; Panter Citation2006; Sprung et al. Citation2007; Timm et al. Citation2009, Citation2010; McCoy-West et al. Citation2010; van der Meer et al. Citation2017; Hoernle et al. Citation2020; Scanlan et al. Citation2020; Scott et al. Citation2020).
WDS and ADS amphibole megacrysts plot within or close to both Cretaceous and Cenozoic intraplate alkaline magmatism arrays in Sr-Nd-Hf-Pb isotopic systems (A–D), forming a general array between MORB and HIMU compositions. Additionally, intraplate magmatism and megacrysts share similar Pb-Nd-Sr-Hf signatures to spinel-facies peridotite xenoliths originating from the Zealandia SCLM (e.g. Scott et al. Citation2014b; McCoy-West et al. Citation2016; Scott et al. Citation2016a; van der Meer et al. Citation2017) (). HIMU-like signatures in SCLM xenoliths in Zealandia have generally been attributed to subduction-related carbonatitic mantle metasomatism, broadly constrained to have occurred sometime in the Cretaceous (Scott et al. Citation2014a, Citation2014b; McCoy-West et al. Citation2016; Scott et al. Citation2016a; Dalton et al. Citation2017; van der Meer et al. Citation2017). Even though the origin of the HIMU-like Sr-Nd-Hf-Pb signatures in alkaline intraplate magmatism in Zealandia still remains under debate, we favour derivation from melting of a metasomatically enriched SCLM. As such, variable carbonatitic metasomatism in lamprophyre source regions is believed to have caused heterogeneous enrichments in Th and U sometime during the Cretaceous, which by ingrowth produced the observed spread in radiogenic 206Pb/204Pb(i) and 208Pb/204Pb(i) signatures in ADS and WDS host rocks and megacrystic amphiboles while retaining similar 207Pb/204Pb(i) and Sr-Nd-Hf signatures (A,C,D). Interestingly, investigated megacrystic amphiboles show HIMU-like 206Pb/204Pb(i) and 208Pb/204Pb(i) signatures that span the entire Zealandia lamprophyre array (). Additionally, we suggest that is not coincidental that Cretaceous WDS amphiboles record both the relatively most constrained and least radiogenic Pb signatures, while Cenozoic ADS megacrysts record the most radiogenic and diverse Pb signatures (). Due to the very low compatibility of Th and U in amphibole, cognate amphibole megacrysts [with low U-Th/Pb ratios (238U/204Pb ∼ 2–6 and 232Th/204Pb ∼ 8–32)] have negligible ingrowth and therefore preserve the Pb isotopic composition of the parental melts and sources at the time of crystallisation. Assuming that variable enrichment due to metasomatism occurred only a relatively short period prior to WDS magmatism, Late Cretaceous WDS megacrystic amphiboles should record relatively unradiogenic and constrained Pb signatures closer to those of pre-metasomatised sources as Th and U enrichment had limited time to substantially modify Pb signatures. By Cenozoic (∼ 25 Ma) times, megacrystic amphiboles will record more radiogenic and varied Pb signatures since longer time has spanned since heterogeneous U and Th enrichment, resulting in more variable degrees of ingrowth in the mantle sources. Such a relationship is exactly what is observed (C,D). Thus, WDS and ADS amphibole megacryst record time-constrained isotopic snapshots of in situ 206Pb and 208Pb ingrowth in mantle sources.
Assuming that megacrystic amphiboles represent early-crystallised phases in Zealandia intraplate lamprophyre magmatic systems, WDS and ADS megacrystic amphiboles should record primary isotopic compositions of sources from which associated host melts originate. Thus, it can be inferred that amphibole megacrysts record moderate heterogeneities in mantle sources that mix to form the variation in Sr-Nd-Hf signatures of intraplate alkaline magmatic arrays. Considering the comparable geochemical and isotopic characteristics of WDS and ADS host rocks and amphibole megacrysts, one can speculate that alkaline intraplate magmatism throughout Zealandia may be derived from similar processes acting in a moderately heterogeneous SCLM reservoir that experienced variable carbonatitic metasomatism.
To test the aforementioned hypothesis, we present Nd-Hf evolution models of a theoretical mantle source, using WDS amphibole megacryst initial isotopic signatures coupled with relevant published mantle reservoir parent/daughter ratios [i.e. Tasman-MORB 147Sm/144Nd from Mortimer et al. (Citation2012); average MORB 176Lu/177Hf and 147Sm/144Nd from Chauvel et al. (Citation2008) and DMM 147Sm/144Nd and 176Lu/177Hf from Salters and Stracke (Citation2004)] (A,B). Calculated models are compared to age-corrected compositions for Zealandia intraplate magmas since the Late Cretaceous (Price Citation2003; Hoernle et al. Citation2006; Sprung et al. Citation2007; Timm et al. Citation2009, Citation2010; McCoy-West et al. Citation2010; van der Meer et al. Citation2017; Hoernle et al. Citation2020; Scanlan et al. Citation2020; Scott et al. Citation2020), present day isotopic signatures of SCLM peridotite xenoliths (Scott et al. Citation2014b; Scott et al. Citation2016a) and relevant mantle reservoirs (). Strikingly, amphibole megacrysts from the ADS and most Zealandia intraplate magmas since the Late Cretaceous plot along calculated mantle source evolution fields in Nd-Hf space (A,B), further indicating that intraplate magmatism in Zealandia is derived from similar sources and processes since the break-up of Gondwana. Late Cretaceous EP Marlborough province magmatism display lower 143Nd/144Nd(i) and 176Hf/177Hf(i) than predicted source signatures (A,B), and may instead be derived from a distinct source originating from the subducting Hikurangi Plateau as proposed by van der Meer et al. (Citation2017). Interestingly, calculated sources evolve to present-day 143Nd/144Nd(0) and 176Hf/177Hf(0) that match signatures of SCLM peridotite xenoliths sampled in Burke River in the ADS by Scott et al. (Citation2016a) (A,B), who proposed that intraplate magmatism in Zealandia may be derived from such a metasomatically enriched and amphibole-bearing SCLM.
Figure 11. A and B, Theoretical Hf and Nd isotope evolution fields for a modelled reservoir using WDS megacryst amphibole initial isotopic ratios coupled with parent/daughter ratios from relevant reservoirs. C and D, Modelled effect of variable U and Th enrichment on Pb isotopic evolutions. Modelled evolution lines are plotted against age-corrected amphibole megacrysts (this study), dredged Tasman MORB evolution from Mortimer et al. (Citation2012); average MORB evolution from Chauvel et al. (Citation2008); approx. DMM evolution from Salters and Stracke (Citation2004) and Stracke et al. (Citation2005) with 238U/204Pb = 13 from White (Citation1993); age-corrected EP alkaline intraplate volcanism data from Price (Citation2003); Hoernle et al. (Citation2006); Sprung et al. (Citation2007); Timm et al. (Citation2009, Citation2010); McCoy-West et al. (Citation2010); Hoernle et al. (Citation2020); Scanlan et al. (Citation2020) and Scott et al. (Citation2020); age-corrected WP alkaline intraplate volcanism data from Timm et al. (Citation2010), van der Meer et al. (Citation2016, Citation2017) and Hoernle et al. (Citation2020); and present-day isotopic signatures of EP SCLM peridotite xenoliths from Scott et al. (Citation2014b) and Scott et al. (Citation2016a).
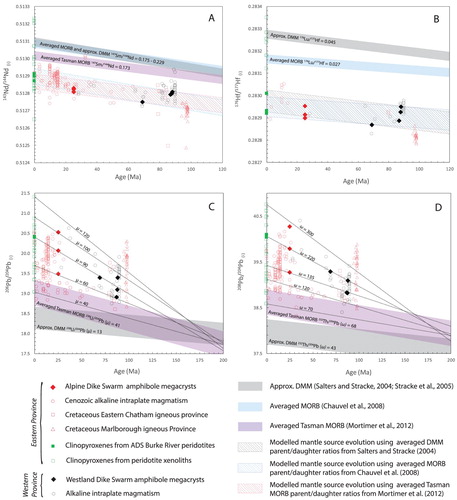
Intraplate magmatism in the WP and especially EP shows varying degrees of radiogenic Pb-enrichment towards HIMU-like signatures (C,D) compared to both Tasman MORB and DMM reservoir evolutions. As discussed, modified Pb signatures have previously been attributed to variable carbonatitic metasomatism in source regions (McCoy-West et al. Citation2016; Scott et al. Citation2016a; van der Meer et al. Citation2017), consistent with reported heterogeneous carbonatitic metasomatism in SCLM xenoliths (e.g. Scott et al. Citation2014a, Citation2014b). Additionally, present day 206Pb/204Pb(0) and 208Pb/204Pb(0) in SCLM xenoliths exhibit a wide range of signatures, similar to amphibole megacrysts and intraplate magmatism in Zealandia (C,D). It is also noteworthy that the least radiogenic Pb signatures in both intraplate magmatism and SCLM xenoliths intersect with the Tasman-MORB Pb evolution fields (C,D). As we have established that amphibole megacrysts likely represent phases which crystallised within lamprophyric melts of the ADS and WDS, investigating such spatially and temporally separate instances of low U-Th/Pb megacrystic amphiboles provide unique insight into the Pb isotopic history of sources from which Zealandia lamprophyres are derived. Studied megacrystic amphiboles record the full spectrum of lamprophyre Pb signatures () and are therefore inferred to also record the full extent of heterogeneous carbonatitic U and Th enrichment in lamprophyre source regions. Late Cretaceous WDS megacrystic amphiboles likely record an early snapshot into the isotopic evolution of Pb in lamprophyre sources before much ingrowth had occurred, whereas Cenozoic ADS megacrystic amphiboles record both the effects of prolonged Pb ingrowth as well as the heterogeneous nature of carbonatitic metasomatism in source regions. Additionally, the close isotopic relationship between amphibole megacrysts, intraplate magmatism and SCLM peridotites indicates that intraplate magmatism may be derived from a metasomatically enriched SCLM source.
Thus, using present-day isotopic signatures of SCLM peridotites coupled with parent-daughter ratios from published Zealandia intraplate intrusives (i.e. 238U/204Pb ∼ 10–120 and 232Th/204Pb ∼ 30–300), we can extrapolate appropriate Pb isotopic evolutions to intersect with both ADS and WDS megacrystic amphiboles as well as EP and WP lamprophyre signatures to t ∼ 90 Ma (C,D). Modelled Pb ingrowth regressions cover the range of HIMU-like intraplate magmatism Pb signatures throughout most of Zealandia since the Late Cretaceous (C,D), except 90–100 Ma EP Marlborough Province magmatism, which has previously been proposed to tap a distinct source with diluted HIMU signatures originating from the subducting Hikurangi Plateau (van der Meer et al. Citation2017). Pb isotopic regressions also indicate that intraplate magmatism in Zealandia is derived from sources which originally had similar isotopic compositions to Tasman MORB prior to Th and U enrichment (C,D). By using Pb regressions to fully encompass Pb ingrowth in intraplate magmatism, we can broadly constrain the timing of metasomatic Th and U enrichment to have occurred in the Jurassic to Middle Cretaceous in both 206Pb/204Pb and 208Pb/204Pb space (C,D), consistent with published estimates (Scott et al. Citation2014a, Citation2014b; McCoy-West et al. Citation2016; van der Meer et al. Citation2017).
In summary, amphibole megacryst and lamprophyre isotopic signatures depict a moderately heterogeneous SCLM source with Tasman MORB-like Nd-Hf parent/daughter isotope ratios. This SCLM source likely originally had similar Pb isotopic compositions to Tasman MORB, which was modified by localised varying degrees of subduction-related heterogeneous carbonatitic U and Th enrichment between the Jurassic and Middle Cretaceous. Our results support studies advocating melting of subduction-modified lithospheric mantle to account for alkaline intraplate magmatism in Zealandia (e.g. Scott et al. Citation2016a; van der Meer et al. Citation2017; van der Meer et al. Citation2019; Cooper Citation2020).
Conclusions
This study presents a comprehensive Sr-Nd-Hf-Pb isotopic and major/trace element dataset of amphibole megacrysts hosted by two spatially and temporally decoupled instances of alkaline intraplate magmatism in Zealandia. Results provide new insights into the genesis of both amphibole megacrysts and alkaline intraplate magmatism in Zealandia.
Geochemical and isotopic evidence suggest that amphibole megacrysts likely represent phenocrysts and/or remnants of fractionated phases belonging to lamprophyric melts.
Discrepancies in Mg# and isotopic signatures in host rock – amphibole megacryst pairs are interpreted to be a consequence of interaction between lamprophyric melts and/or cumulates in the lower crust derived from repeated tapping of a moderately heterogeneous mantle source.
Amphibole megacrysts from both the Cenozoic ADS and Cretaceous WDS show remarkably similar trace element and REE patterns as well as overlapping narrow ranges of Sr-Nd-Hf isotopic signatures (i.e. 87Sr/86Sr(i) = 0.702754–0.703371, εNd(i) = +3.9 to +5.5 and εHf(i) = +5.0 to +8.2), suggesting similar formation processes.
Diverse HIMU-like Pb isotopic signatures coupled with low Th-U/Pb ratios in amphibole megacrysts record the evolution of 206Pb and 208Pb ingrowth in intraplate sources since U and Th enrichment by carbonatitic metasomatism in the Jurassic to Middle Cretaceous.
Source modelling suggest that most alkaline intraplate magmatism throughout Zealandia is derived from moderately heterogeneous SCLM sources that suffered localised and varying degrees of subduction-related carbonatitic metasomatism between the Jurassic to Middle Cretaceous during Gondwana break-up, in agreement with results from recent studies.
Acknowledgements
We would like to thank Antonio M. Álvarez-Valero and an anonymous reviewer for very helpful comments that helped improve the final version of the paper. We thank Toni Larsen, Toby Leeper, Mojagan Alaei and Olga Nielsen for assistance in the laboratory work.
Disclosure statement
No potential conflict of interest was reported by the author(s).
Data availability statement
A detailed description of methodologies and standards as well as data that support the findings of this study are openly available in Figshare at 10.6084/m9.figshare.12057843.
Additional information
Funding
References
- Adam J, Green T. 2006. Trace element partitioning between mica- and amphibole-bearing garnet lherzolite and hydrous basanitic melt: 1. Experimental results and the investigation of controls on partitioning behaviour. Contributions to Mineralogy and Petrology. 152(1):1–17. doi: 10.1007/s00410-006-0085-4
- Adams CJ, Cooper AF. 1996. K-Ar age of a lamprophyre dike swarm near Lake Wanaka, west Otago, South Island, New Zealand. New Zealand Journal of Geology and Geophysics. 39(1):17–23. doi: 10.1080/00288306.1996.9514691
- Ben Othman D, Tilton GR, Menzies MA. 1990. Pb, Nd and Sr isotopic investigations of kaersutite and clinopyroxene from ultramafic nodules, and their host basalts: the nature of the subcontinental mantle. Geochimica et Cosmochimica Acta. 54(12):3449–3460. doi: 10.1016/0016-7037(90)90297-X
- Best MG. 1974. Mantle-derived amphibole within inclusions in alkalic-basaltic lavas. Journal of Geophysical Research. 79(14):2107–2113. doi: 10.1029/JB079i014p02107
- Binns RA. 1969. High-pressure megacrysts in basanitic lavas near Armidale, New South Wales. Americal Journal of Science. 267(A):33–49.
- Bodinier J-L. 2004. Silicate, hydrous and carbonate metasomatism at Lherz, France: contemporaneous derivatives of silicate melt-harzburgite reaction. Journal of Petrology. 45(2):299–320. doi: 10.1093/petrology/egg107
- Boettcher AL, O’Neil JR. 1980. Stable isotope, chemical, and petrographic studies of high-pressure amphiboles and micas : evidence for metasomatism in the mantle source regions of alkali basalts and kimberlites. American Journal of Science. 280(A):594–621.
- Chauvel C, Lewin E, Carpentier M, Arndt NT, Marini J-C. 2008. Role of recycled oceanic basalt and sediment in generating the Hf–Nd mantle array. Nature Geoscience. 1(1):64–67. doi: 10.1038/ngeo.2007.51
- Christensen NI, Mooney WD. 1995. Seismic velocity structure and composition of the continental crust: a global view. Journal of Geophysical Research. 100(B6):9761–9788. doi: 10.1029/95JB00259
- Coltorti M, Beccaluva L, Bonadiman C, Faccini B, Ntaflos T, Siena F. 2004. Amphibole genesis via metasomatic reaction with clinopyroxene in mantle xenoliths from Victoria Land, Antarctica. Lithos. 75(1–2):115–139. doi: 10.1016/j.lithos.2003.12.021
- Coltorti M, Bonadiman C, Faccini B, Grégoire M, O’Reilly SY, Powell W. 2007. Amphiboles from suprasubduction and intraplate lithospheric mantle. Lithos. 99(1–2):68–84. doi: 10.1016/j.lithos.2007.05.009
- Cooper AF. 2020. Petrology and petrogenesis of an intraplate alkaline lamprophyre-phonolite-carbonatite association in the Alpine Dyke Swarm, New Zealand. New Zealand Journal of Geology and Geophysics. in press.
- Cooper AF, Barreiro BA, Kimbrough DL, Mattinson JM. 1987. Lamprophyre dike intrusion and the age of the Alpine fault, New Zealand. Geology. 15(10):941–944. doi: 10.1130/0091-7613(1987)15<941:LDIATA>2.0.CO;2
- Cooper AF, Paterson LA. 2008. Carbonatites from a lamprophyric dyke-swarm, South Westland, New Zealand. The Canadian Mineralogist. 46(4):753–777. doi: 10.3749/canmin.46.4.753
- Czertowicz TA, Scott JM, Waight TE, Palin JM, Van der Meer QHA, Le Roux P, Münker C, Piazolo S. 2016. The Anita Peridotite, New Zealand: ultra-depletion and subtle enrichment in sub-arc mantle. Journal of Petrology. 57(4):717–750. doi: 10.1093/petrology/egw001
- Dalton HB, Scott JM, Liu J, Waight TE, Pearson DG, Brenna M, Le Roux P, Palin JM. 2017. Diffusion-zoned pyroxenes in an isotopically heterogeneous mantle lithosphere beneath the Dunedin Volcanic Group, New Zealand, and their implications for intraplate alkaline magma sources. Lithosphere. 9(3):463–475. doi: 10.1130/L631.1
- Dautria JM, Liotard JM, Cabanes N, Girod M, Briqueu L. 1987. Amphibole-rich xenoliths and host alkali basalts: petrogenetic constraints and implications on the recent evolution of the upper mantle beneath Ahaggar (Central Sahara, Southern Algeria). Contributions to Mineralogy and Petrology. 95(2):133–144. doi: 10.1007/BF00381263
- Dickey JS. 1968. Eclogitic and other inclusions in the mineral breccia member of the Deborah volcanic formation at Kakanui, New Zealand. The American Mineralogist. 53:1304–1319.
- Dobosi G, Downes H, Embey-Isztin A, Jenner GA. 2003. Origin of megacrysts and pyroxenite xenoliths from the Pliocene alkali basalts of the Pannonian Basin (Hungary). Neues Jahrbuch für Mineralogie – Abhandlungen. 178(3):217–237.
- Downes H. 2001. Formation and modification of the shallow sub-continental lithospheric mantle: a review of geochemical evidence from ultramafic xenolith suites and tectonically emplaced ultramafic massifs of western and central Europe. Journal of Petrology. 42(1):233–250. doi: 10.1093/petrology/42.1.233
- Finn CA, Müller RD, Panter KS. 2005. A Cenozoic diffuse alkaline magmatic province (DAMP) in the southwest Pacific without rift or plume origin. Geochemistry, Geophysics, Geosystems. 6(2):1–26. doi: 10.1029/2004GC000723
- Foley SF, Andronikov AV, Melzer S. 2002. Petrology of ultramafic lamprophyres from the Beaver Lake area of Eastern Antarctica and their relation to the breakup of Gondwanaland. Mineralogy and Petrology. 74(2–4):361–384. doi: 10.1007/s007100200011
- Frost DJ. 2006. The stability of hydrous mantle phases. Reviews in Mineralogy and Geochemistry. 62(1):243–271. doi: 10.2138/rmg.2006.62.11
- Fulmer EC, Nebel O, van Westrenen W. 2010. High-precision high field strength element partitioning between garnet, amphibole and alkaline melt from Kakanui, New Zealand. Geochimica et Cosmochimica Acta. 74(9):2741–2759. doi: 10.1016/j.gca.2010.02.020
- Gaina C, Müller DR, Royer J-Y, Stock J, Hardebeck J, Symonds P. 1998. The tectonic history of the Tasman Sea: a puzzle with 13 pieces. Journal of Geophysical Research. 103(B6):12413–12433. doi: 10.1029/98JB00386
- Griffin WL, O’Reilly SY, Doyle BJ, Pearson NJ, Coopersmith H, Kivi K, Malkovets V, Pokhilenko N. 2004. Lithosphere mapping beneath the North American plate. Lithos. 77(1–4):873–922. doi: 10.1016/j.lithos.2004.03.034
- Han B, Liu J, Zhang L. 2008. A noncognate relationship between megacrysts and host basalts from the Tuoyun Basin, Chinese Tian Shan. The Journal of Geology. 116(5):499–509. doi: 10.1086/590136
- Hart SR. 1984. A large-scale isotope anomaly in the Southern Hemisphere mantle. Nature. 309(5971):753–757. doi: 10.1038/309753a0
- Hawthorne FC, Oberti R, Harlow GE, Maresch WV, Martin RF, Schumacher JC, Welch MD. 2012. Nomenclature of the amphibole supergroup. American Mineralogist. 97(11–12):2031–2048. doi: 10.2138/am.2012.4276
- Hoernle K, Timm C, Hauff F, Tappenden V, Werner R, Jolis EM, Mortimer N, Weaver S, Riefstahl F, Gohl K. 2020. Late Cretaceous (99-69 Ma) basaltic intraplate volcanism on and around Zealandia: tracing upper mantle geodynamics from Hikurangi Plateau collision to Gondwana breakup and beyond. Earth and Planetary Science Letters. 529:1–12. doi: 10.1016/j.epsl.2019.115864
- Hoernle K, White JDL, van den Bogaard P, Hauff F, Coombs DS, Werner R, Timm C, Garbe-Schönberg D, Reay A, Cooper AF. 2006. Cenozoic intraplate volcanism on New Zealand: upwelling induced by lithospheric removal. Earth and Planetary Science Letters. 248(1–2):350–367. doi: 10.1016/j.epsl.2006.06.001
- Ionov DA, Hofmann AW. 1995. Nb & Ta-rich mantle amphiboles and micas: implications for subduction-related metasomatic trace element fractionations. Earth and Planetary Science Letters. 131(3–4):341–356. doi: 10.1016/0012-821X(95)00037-D
- Irving AJ, Frey FA. 1984. Trace element abundances in megacrysts and their host basalts: constraints on partition coefficients and megacryst genesis. Geochimica et Cosmochimica Acta. 48(6):1201–1221. doi: 10.1016/0016-7037(84)90056-5
- Kesson S, Price RC. 1972. The major and trace element chemistry of kaersutite and its bearing on the petrogenesis of alkaline rocks. Contributions to Mineralogy and Petrology. 35(2):119–124. doi: 10.1007/BF00370923
- Laird MG, Bradshaw JD. 2004. The break-up of a long-term relationship: the Cretaceous separation of New Zealand from Gondwana. Gondwana Research. 7(1):273–286. doi: 10.1016/S1342-937X(05)70325-7
- Locock AJ. 2014. An Excel spreadsheet to classify chemical analyses of amphiboles following the IMA 2012 recommendations. Computers & Geosciences. 62:1–11. doi: 10.1016/j.cageo.2013.09.011
- Mayer B, Jung S, Romer RL, Pfänder JA, Klügel A, Pack A, Gröner E. 2014. Amphibole in alkaline basalts from intraplate settings: implications for the petrogenesis of alkaline lavas from the metasomatised lithospheric mantle. Contrib Mineral Petrol. 167(3):1–22. doi: 10.1007/s00410-014-0989-3
- McCoy-West AJ, Baker JA, Faure K, Wysoczanski R. 2010. Petrogenesis and origins of Mid-Cretaceous continental intraplate volcanism in Marlborough, New Zealand: implications for the long-lived HIMU magmatic mega-province of the SW Pacific. Journal of Petrology. 51(10):2003–2045. doi: 10.1093/petrology/egq046
- McCoy-West AJ, Bennett VC, Amelin Y. 2016. Rapid Cenozoic ingrowth of isotopic signatures simulating “HIMU” in ancient lithospheric mantle: distinguishing source from process. Geochimica et Cosmochimica Acta. 187:79–101. doi: 10.1016/j.gca.2016.05.013
- McCoy-West AJ, Bennett VC, O’Neill HSC, Hermann J, Puchtel IS. 2015. The interplay between melting, refertilization and carbonatite metasomatism in off-cratonic lithospheric mantle under Zealandia: an integrated major, trace and platinum group element study. Journal of Petrology. 56:563–604. doi: 10.1093/petrology/egv011
- McCoy-West AJ, Bennett VC, Puchtel IS, Walker RJ. 2013. Extreme persistence of cratonic lithosphere in the southwest Pacific: Paleoproterozoic Os isotopic signatures in Zealandia. Geology. 41:231–234. doi: 10.1130/G33626.1
- McDonough WF, Sun S-s. 1995. The composition of the Earth. Chemical Geology. 120(3–4):223–253. doi: 10.1016/0009-2541(94)00140-4
- Merrill RB, Wyllie PJ. 1975. Kaersutite and kaersutite eclogite from Kakanui, New Zealand — water-excess and water-deficient melting to 30 kilobars. Geological Society of America Bulletin. 86(4):555–570. doi: 10.1130/0016-7606(1975)86<555:KAKEFK>2.0.CO;2
- Mortimer N. 2004. New Zealand’s Geological Foundations. Gondwana Research. 7(1):261–272. doi: 10.1016/S1342-937X(05)70324-5
- Mortimer N, Campbell HJ, Tulloch AJ, King PR, Stagpoole VM, Wood RA, Rattenbury MS, Sutherland R, Adams CJ, Collot J, Seton M. 2017. Zealandia: Earth’s hidden continent. GSA Today. 27(3):27–35. doi: 10.1130/GSATG321A.1
- Mortimer N, Gans PB, Hauff F, Barker DHN. 2012. Paleocene MORB and OIB from the Resolution Ridge, Tasman Sea. Australian Journal of Earth Sciences. 59(6):953–964. doi: 10.1080/08120099.2012.676569
- Nielson JE, Budahn JR, Unruh DM, Wilshire HG. 1993. Actualistic models of mantle metasomatism documented in a composite xenolith from Dish Hill, California. Geochimica et Cosmochimica Acta. 57(1):105–121. doi: 10.1016/0016-7037(93)90472-9
- Pandey R, Rao NVC, Pandit D, Sahoo S, Dhote P. 2018. Imprints of modal metasomatism in the post-Deccan subcontinental lithospheric mantle: petrological evidence from an ultramafic xenolith in an Eocene lamprophyre, NW India. Geological Society, London, Special Publications. 463(1):117–136. doi: 10.1144/SP463.6
- Panter KS. 2006. The origin of HIMU in the SW Pacific: evidence from intraplate volcanism in Southern New Zealand and subantarctic islands. Journal of Petrology. 47(9):1673–1704. doi: 10.1093/petrology/egl024
- Pilet S, Ulmer P, Villiger S. 2010. Liquid line of descent of a basanitic liquid at 1.5 Gpa: constraints on the formation of metasomatic veins. Contributions to Mineralogy and Petrology. 159(5):621–643. doi: 10.1007/s00410-009-0445-y
- Price RC. 2003. Phonolitic diatremes within the Dunedin Volcano, South Island, New Zealand. Journal of Petrology. 44(11):2053–2080. doi: 10.1093/petrology/egg070
- Putirka K. 2016. Amphibole thermometers and barometers for igneous systems and some implications for eruption mechanisms of felsic magmas at arc volcanoes. American Mineralogist. 101(4):841–858. doi: 10.2138/am-2016-5506
- Ridolfi F, Renzulli A. 2012. Calcic amphiboles in calc-alkaline and alkaline magmas: thermobarometric and chemometric empirical equations valid up to 1,130°C and 2.2 GPa. Contributions to Mineralogy and Petrology. 163(5):877–895. doi: 10.1007/s00410-011-0704-6
- Rock NMS. 1991. Lamprophyres. Glasgow: Blackie and Sons Ltd.
- Roden MK, Hart SR, Frey FA, Melson WG. 1984. Sr, Nd and Pb isotopic and REE geochemistry of St. Paul’s Rocks: the metamorphic and metasomatic development of an alkali basalt mantle source. Contributions to Mineralogy and Petrology. 85(4):376–390. doi: 10.1007/BF01150294
- Salmon M, Kennett BLN, Stern T, Aitken ARA. 2013. The Moho in Australia and New Zealand. Tectonophysics. 609:288–298. doi: 10.1016/j.tecto.2012.07.009
- Salters VJ, Stracke A. 2004. Composition of the depleted mantle. Geochemistry, Geophysics, Geosystems. 5(5):1–27. doi: 10.1029/2003GC000597
- Scanlan E, Scott J, le Roux P. 2020. Pyro metamorphosed Otago Schist xenoliths reveal domainal equilibration and limited chemical contamination of host Dunedin Volcanic Group basanite. New Zealand Journal of Geology and Geophysics. in press.
- Scott JM. 2013. A review of the location and significance of the boundary between the Western Province and Eastern Province, New Zealand. New Zealand Journal of Geology and Geophysics. 56(4):276–293. doi: 10.1080/00288306.2013.812971
- Scott JM 2020. An updated catalogue of New Zealand’s mantle peridotite and serpentinite. New Zealand Journal of Geology and Geophysics, in press.
- Scott JM, Brenna M, Crase JA, Waight TE, van der Meer QHA, Cooper AF, Michael Palin J, Le Roux P, Münker C. 2016a. Peridotitic lithosphere metasomatized by volatile-bearing melts, and its association with intraplate alkaline HIMU-like magmatism. Journal of Petrology. 57(10):2053–2078. doi: 10.1093/petrology/egw069
- Scott JM, Hodgkinson A, Palin JM, Waight TE, Van der Meer QHA, Cooper AF. 2014a. Ancient melt depletion overprinted by young carbonatitic metasomatism in the New Zealand lithospheric mantle. Contributions to Mineralogy and Petrolology. 167(1):1–17.
- Scott JM, Liu J, Pearson DG, Harris GA, Czertowicz TA, Woodland SJ, Luth RW. 2019. Continent stabilisation by lateral accretion of subduction zone-processed depleted mantle residues: insights from Zealandia. Earth & Planetary Science Letters. 507:175–186. doi: 10.1016/j.epsl.2018.11.039
- Scott JM, Liu J, Pearson DG, Waight TE. 2016b. Mantle depletion and metasomatism recorded in orthopyroxene in highly depleted peridotites. Chemical Geology. 441:280–291. doi: 10.1016/j.chemgeo.2016.08.024
- Scott JM, Muhling J, Fletcher I, Billia M, Palin JM, Elliot T, Günter C. 2011. The relationship of Palaeozoic metamorphism and S-type magmatism on the paleo-Pacific Gondwana margin. Lithos. 127(3–4):522–534. doi: 10.1016/j.lithos.2011.09.008
- Scott JM, Pontesilli A, Brenna M, White JDL, Giacalone E, Palin JM, le Roux PJ. 2020. The Dunedin Volcanic Group and a revised model for Zealandia’s alkaline intraplate volcanism. New Zealand Journal of Geology and Geophysics. in press.
- Scott JM, Waight TE, van der Meer QHA, Palin JM, Cooper AF, Münker C. 2014b. Metasomatized ancient lithospheric mantle beneath the young Zealandia microcontinent and its role in HIMU-like intraplate magmatism. Geochemistry, Geophysics, Geosystems. 15(9):3477–3501. doi: 10.1002/2014GC005300
- Shaw CSJ, Eyzaguirre J. 2000. Origin of megacrysts in the mafic alkaline lavas of the West Eifel volcanic field, Germany. Lithos. 50(1–3):75–95. doi: 10.1016/S0024-4937(99)00048-1
- Sprung P, Schuth S, Münker C, Hoke L. 2007. Intraplate volcanism in New Zealand: the role of fossil plume material and variable lithospheric properties. Contributions to Mineralogy and Petrology. 153(6):669–687. doi: 10.1007/s00410-006-0169-1
- Stracke A, Hofmann AW, Hart SR. 2005. FOZO, HIMU, and the rest of the mantle zoo. Geochemistry, Geophysics, Geosystems. 6(5):1–20. doi: 10.1029/2004GC000824
- Timm C, Hoernle K, Van Den Bogaard P, Bindeman I, Weaver S. 2009. Geochemical evolution of intraplate volcanism at Banks Peninsula, New Zealand: interaction between asthenospheric and lithospheric melts. Journal of Petrology. 50(6):989–1023. doi: 10.1093/petrology/egp029
- Timm C, Hoernle K, Werner R, Hauff F, van den Bogaard P, White J, Mortimer N, Garbe-Schönberg D. 2010. Temporal and geochemical evolution of the Cenozoic intraplate volcanism of Zealandia. Earth-Science Reviews. 98(1–2):38–64. doi: 10.1016/j.earscirev.2009.10.002
- Tulloch AJ, Nathan S. 1990. Spinel harzburgite xenoliths in alkali basalt and camptonite from North Westland and southeast Nelson, New Zealand. New Zealand Journal of Geology and Geophysics. 33(4):529–534. doi: 10.1080/00288306.1990.10421370
- Ubide T, Galé C, Arranz E, Lago M, Larrea P. 2014. Clinopyroxene and amphibole crystal populations in a lamprophyre sill from the Catalonian Coastal Ranges (NE Spain): a record of magma history and a window to mineral-melt partitioning. Lithos. 184–187:225–242. doi: 10.1016/j.lithos.2013.10.029
- Ulrych J, Krmíček L, Teschner C, Skála R, Adamovič J, Ďurišová J, Křížová Š, Kuboušková S, Radoň M. 2018. Chemistry and Sr-Nd isotope signature of amphiboles of the magnesio-hastingsite–pargasite–kaersutite series in Cenozoic volcanic rocks: insight into lithospheric mantle beneath the Bohemian Massif. Lithos. 312–313:308–321. doi: 10.1016/j.lithos.2018.05.017
- van der Meer QHA, Scott JM, Serre SH, Whitehouse MJ, Kristoffersen M, le Roux PJ, Pope EC. 2019. Low-δ18O zircon xenocrysts in alkaline basalts; a window into the complex carbonatite-metasomatic history of the Zealandia lithospheric mantle. Geochimica et Cosmochimica Acta. 254:21–39. doi: 10.1016/j.gca.2019.03.029
- van der Meer QHA, Scott J, Waight T, Sudo M, Scherstén A, Cooper A, Spell T. 2013. Magmatism during Gondwana break-up: new geochronological data from Westland, New Zealand. New Zealand Journal of Geology and Geophysics. 56(4):229–242. doi: 10.1080/00288306.2013.826699
- van der Meer QHA, Storey M, Scott JM, Waight TE. 2016. Abrupt spatial and geochemical changes in lamprophyre magmatism related to Gondwana fragmentation prior, during and after opening of the Tasman Sea. Gondwana Research. 36:142–156. doi: 10.1016/j.gr.2016.04.004
- van der Meer QHA, Waight TE, Scott JM, Münker C. 2017. Variable sources for Cretaceous to recent HIMU and HIMU-like intraplate magmatism in New Zealand. Earth and Planetary Science Letters. 469:27–41. doi: 10.1016/j.epsl.2017.03.037
- van Der Meer QHA, Waight TE, Tulloch AJ, Whitehouse MJ, Andersen T. 2018. Magmatic evolution during the Cretaceous transition from subduction to continental break-up of the Eastern Gondwana margin (New Zealand) documented by in-situ zircon O–Hf isotopes and bulk-rock Sr–Nd isotopes. Journal of Petrology. 59(5):849–880. doi: 10.1093/petrology/egy047
- Vaselli O, Downes H, Thirlwall M, Dobosi G, Coradossi N, Seghedi I, Szakacs A, Vannucci R. 1995. Ultramafic xenoliths in Plio-Pleistocene alkali basalts from the eastern Transylvanian Basin: depleted mantle enriched by vein metasomatism. Journal of Petrology. 36(1):23–53. doi: 10.1093/petrology/36.1.23
- Vervoort JD, Blichert-Toft J. 1999. Evolution of the depleted mantle: Hf isotope evidence from juvenile rocks through time. Geochimica et Cosmochimica Acta. 63(3–4):533–556. doi: 10.1016/S0016-7037(98)00274-9
- Waight TE. 1995. The geology and geochemistry of the Hohonu Batholith and adjacent rocks, North Westland, New Zealand [PhD dissertation]. Christchurch (NZ): University of Canterbury.
- Waight TE, Weaver SD, Maas R, Eby GN. 1998a. French Creek Granite and Hohonu Dyke Swarm, South Island, New Zealand: late Cretaceous alkaline magmatism and the opening of the Tasman Sea. Australian Journal of Earth Sciences. 45(6):823–835. doi: 10.1080/08120099808728438
- Waight TE, Weaver SD, Muir RJ. 1998b. Mid-Cretaceous granitic magmatism during the transition from subduction to extension in southern New Zealand: a chemical and tectonic synthesis. Lithos. 45(1–4):469–482. doi: 10.1016/S0024-4937(98)00045-0
- White WM. 1993. 238U/204Pb in MORB and open system evolution of the depleted mantle. Earth and Planetary Science Letters. 115(1–4):211–226. doi: 10.1016/0012-821X(93)90223-V
- Wilshire HG, Trask NJ. 1971. Structural and textural relationships of amphibole and phlogopite in peridotite inclusions, Dish hill, California. The American Mineralogist. 56:240–255.
- Witt-Eickschen G. 2003. Lithospheric mantle evolution beneath the Eifel (Germany): constraints from Sr-Nd-Pb isotopes and trace element abundances in spinel peridotite and pyroxenite xenoliths. Journal of Petrology. 44(6):1077–1095. doi: 10.1093/petrology/44.6.1077
- Witt-Eickschen G, Kaminsky W, Kramm U, Harte B. 1998. The nature of Young vein metasomatism in the lithosphere of the West Eifel (Germany): geochemical and isotopic Constraints from composite mantle xenoliths from the Meerfelder Maar. Journal of Petrology. 39(1):155–185. doi: 10.1093/petroj/39.1.155
- Zanetti A, Vannucci R, Oberti R, Ottolini L. 1996. Infiltration metasomatism at Lherz as monitored by systematic ion-microprobe investigations close to a homblendite vein. Chemical Geology. 134:113–133. doi: 10.1016/S0009-2541(96)00080-0