ABSTRACT
Nitrogen is an essential element for living organisms because it is a crucial constituent of biomolecules. Inadequate supply of usable nitrogen reduces plant growth and crop yield. The primary nitrogen sources for plants are nitrate and ammonium in soils, and plants have multiple layers of sensing and adaptive mechanisms that respond to the availability of these nutrients. The adaptive responses are called ‘nitrogen responses,’ which include morphological and physiological responses enabling plants to efficiently take up nitrogen and adapt to spatiotemporal fluctuations in nitrogen abundance in the field. In this review, we summarize the strategies that plants use to respond to changes in the nitrogen nutrient status in the soil and discuss different effects produced by nitrate and ammonium, emphasizing the important role of nitrate for plant growth. Recent studies revealed the molecular mechanism mediating the primary response to nitrate provision and the molecular mechanisms that coordinate the nitrogen response with responses to another macronutrient, phosphorus. We thus discuss these molecular mechanisms as well.
1. Introduction
Nitrogen is a crucial element for all living organisms because it is a constituent of numerous biomolecules, including proteins, nucleic acids, and secondary metabolites (Miller and Cramer Citation2005). Plants require copious amounts of nitrogen to sustain growth, and thus, nitrogen acquisition is frequently a limiting factor of plant growth and crop production in both natural and agricultural ecosystems. The Haber–Bosch process, an artificial nitrogen-fixation reaction developed in the early twentieth century, enabled the production of nitrogen fertilizers on an industrial scale. Since then, huge amounts of nitrogen fertilizer have been applied to agricultural fields to boost crop yields (Erisman et al. Citation2008). Nitrogen fertilizer has arguably been the most element in increasing the global food supply during the twentieth century. An estimation of nitrogen fertilization and crop production (Erisman et al. Citation2008) suggests that nitrogen fertilizer is feeding approximately one-half of the world’s population by increasing crop productivity. Therefore, an understanding of how plants sense nitrogen sources and adapt their physiological and developmental processes to a spatiotemporally inhomogeneous nitrogen supply is crucial for the maintenance and improvement of current crop production.
Plant adaptation responses to diverse nitrogen nutrition conditions or fluctuations in the nitrogen supply are collectively designated as the nitrogen response, which is a critical aspect of the regulatory network controlling plant growth. Extensive physiological, molecular, and genetic studies have been performed to characterize the nitrogen response and elucidate the underlying mechanisms. Plant responses to nitrate (the nitrate response) have been especially intensively investigated, because nitrate, the dominant nitrogen resource in oxidative terrestrial ecosystems, functions as signaling molecule that induces several cellular responses (Konishi and Yanagisawa Citation2014; Medici and Krouk Citation2014). Many excellent reviews have summarized our knowledge of the nitrogen response (Hodge Citation2004; Tsay et al. Citation2011; Wang et al. Citation2012; Xu et al. Citation2012; Nacry et al. Citation2013; Forde Citation2014; Bloom Citation2015; Krapp Citation2015; Brien et al. Citation2016; Hachiya and Sakakibara Citation2016; Kiba and Krapp Citation2016). In this review, we provide an overview of nitrogen responses in plants by comparing the use of nitrate or ammonium as the nitrogen source. We also summarize recent progress in our knowledge of the mechanism regulating the nitrate response, and the mechanisms that coordinate the nitrogen response with responses to phosphorus.
2. Nitrogen response
Plants take up nitrogen as nitrate, ammonium, or organic nitrogen (amino acids and urea) from the rhizosphere (Miller and Cramer Citation2005). In the natural ecosystem, plants generally acquire little amino acids as a nitrogen source because of competition with soil microorganisms (Owen and Jones Citation2001). The contribution to plant growth from amino acids is therefore negligible. The primary accessible forms of nitrogen for plants are nitrate and ammonium (Owen and Jones Citation2001). These molecules are absorbed by roots, allocated to different tissues via specific transporters, and then differentially induce distinct nitrogen responses in specific tissues (reviewed in Krapp Citation2015; Brien et al. Citation2016; Kiba and Krapp Citation2016). Accordingly, the nitrogen response involves nitrate- and ammonium-induced changes in developmental and physiological processes. In this section, we provide an overview of morphological and physiological responses to nitrate and ammonium, which are summarized in .
Figure 1. Overview of nitrogen response in plants. Nitrogen source limitation (right half) leads to axial root elongation and root cortical aerenchyma formation. Nitrogen source limitation also increases ammonium and nitrate uptake activity. Supplementation of nitrogen nutrients (left half), either nitrate or ammonium, induces lateral root proliferation and increase of nitrate or ammonium uptake capacity. Note that the promotion of root cortical aerenchyma formation under nitrogen limitation has been reported only in rice and maize. The specific responses might differ among different plant species.
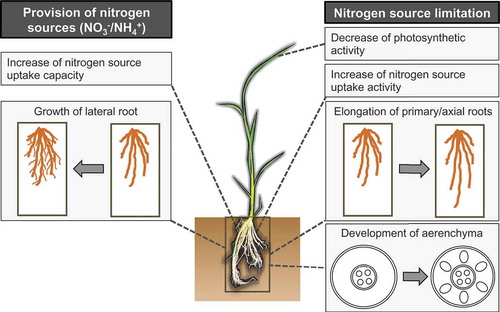
2.1. Morphological response to nitrogen
In the field, nitrogen sources are not evenly distributed due to localized fertilizer application and the spatially inhomogeneous distribution of soil microorganisms with nitrification or denitrification activity (Hodge Citation2004; Hinsinger et al. Citation2005; Gallardo et al. Citation2006; Li et al. Citation2016). To contend with the uneven soil distribution of nitrogen, plants sense nitrogen sources and subsequently change their root architecture to effectively exploit available nitrogen sources. The most evident change is the enhancement of lateral root growth in response to a localized source of nitrate or ammonium, which has been confirmed in many plant species of both monocots and dicots (Drew Citation1975; Remans et al. Citation2006). The effects of these inorganic nitrogen compounds on lateral root growth are complex and depend on the nitrate and ammonium concentrations. The maximum effect on lateral root proliferation was observed in the presence of moderate nitrate and ammonium concentrations (approximately 0.5 and 0.8 mM, respectively), whereas higher concentrations (>10 and >5 mM, respectively) inhibited lateral root growth in Arabidopsis (Zhang et al. Citation2007; Lima et al. Citation2010).
Nitrate and ammonium differentially influence root growth. In general, local nitrate supply primarily affects lateral root elongation, whereas local ammonium supply primarily affects lateral root initiation and higher order lateral root branching, thereby increasing lateral root density (Lima et al. Citation2010; Giehl et al. Citation2014). This appears to be reasonable, because the diffusion coefficient of ammonium in soil is low (Clarke and Barley Citation1968; Hinsinger et al. Citation2005). By contrast, nitrate has a higher diffusion rate in soil due to its negative charge, and its soil concentration can be relatively high (Hodge Citation2004; Miller and Cramer Citation2005). When nitrate is abundant in soil, plants take up large amounts of nitrate through mass flow of soil water rather than by nitrate diffusion (Zhang et al. Citation2007). Therefore, the promotion of lateral root elongation appears to provide only a small benefit for plants (Hodge Citation2004). However, several studies have proposed that lateral root elongation can be advantageous when different plant species are cropped together under finite local nitrogen conditions (Robinson et al. Citation1999; Hodge Citation2004).
The nitrogen nutrient condition also affects root architecture by controlling primary root elongation and axial root growth, as observed in studies with alfalfa, tomato, rice, and maize. Nitrogen starvation consistently promoted axial root growth in those plant species (Chun et al. Citation2005; Yoneyama et al. Citation2012; Zhang et al. Citation2012; Sun et al. Citation2014). However, localized nitrogen source provision yielded inconsistent effects on primary root growth in different studies. The application of 5 mM nitrate to the bottom region of roots in plants grown with glutamine as the sole nitrogen source did not significantly affect primary root growth in the Arabidopsis C24 ecotype but did enhance primary root elongation in other Arabidopsis ecotypes (Walch-Liu and Forde Citation2008). Lima et al. (Citation2010) also reported an enhancement of primary root length in response to local application of nitrogen sources (0.05–50 mM nitrate or 0.3–1 mM ammonium) to the middle region of primary roots of Arabidopsis seedlings that had been cultured for 10 days in the absence of nitrogen before the application. By contrast, Vidal et al. (Citation2010) reported that 5 mM nitrate application inhibited primary root growth of Arabidopsis seedlings grown with ammonium as the sole nitrogen source. High nitrate concentrations (>5 mM) also negatively affected axial root growth in maize (Tian et al. Citation2008). From these results, the effect of nitrogen source supply on axial root growth appears to depend on preculture conditions, although the effect has not been conclusively elucidated.
The mechanisms underlying root architecture modification in response to nitrogen nutrient conditions have been studied extensively in Arabidopsis. These studies revealed that the nitrate transporter 1.1 (NRT1.1) not only takes up nitrate but also facilitates transport of the phytohormone auxin and that changes in auxin level mediated by NRT1.1 in the lateral root primordium has a central role in nitrate-induced lateral root growth (Krouk et al. Citation2010; Tsay et al. Citation2011; Mounier et al. Citation2014; Bouguyon et al. Citation2016; Krouk Citation2016). Much less is known about the mechanism underlying ammonium-induced changes in root architecture morphology, although ammonium transporters (AMTs) and GDP-mannose pyrophosphorylase are likely involved (Qin et al. Citation2008; Lima et al. Citation2010; Tsay et al. Citation2011). Several novel factors involved in nitrogen-dependent lateral root growth have been identified through recent phenome analysis of Arabidopsis ecotypes, subsequent genetic mapping of causative genes, and validation using mutants. The factors identified include JASMONATE RESPONSIVE 1 (JR1) and D-AMINO ACID RACEMASE2 (DAAR2), which are involved in lateral root growth under low nitrate conditions (0.03 mM KNO3) (Gifford et al. Citation2013), and ROOT SYSTEM ARCHITECTURE1 (RSA1), which is involved in determining the ratio of primary root to lateral roots in seedlings grown in medium containing 1 mM nitrate after transfer from nitrogen-free medium (Rosas et al. Citation2013). JR1 is a jasmonate-responsive gene encoding a mannose-binding lectin superfamily protein involved in flowering time and heat stress tolerance (Xiao et al. Citation2015; Echevarría-Zomeño et al. Citation2016), while DAAR2 and RSA1 encode uncharacterized proteins (phenazine biosynthesis-like domain-containing protein and tyrosine transaminase family protein, respectively). These findings indicate that root architecture modification in response to nitrogen nutrients is a very complex process, and thus additional factors will be identified in the future. Several reviews have summarized the current knowledge of these factors (Zhang et al. Citation2007; Tsay et al. Citation2011; Forde Citation2014; Brien et al. Citation2016).
Nitrogen-deficient conditions promote aerenchyma formation in maize and rice root cortex (He et al. Citation1992; Siyiannis et al. Citation2012; Abiko and Obara Citation2014). Thus, plants can adapt to diverse nitrogen nutrient conditions by changing both root architecture morphology and internal root structure. Root cortical aerenchyma formation, which is caused by cell death (Drew et al. Citation2000), is likely under the control of internal nitrogen metabolite levels rather than external nitrate or ammonium levels because root aerenchyma formation is promoted by removing nitrate or ammonium from culture medium (He et al. Citation1992; Siyiannis et al. Citation2012; Abiko and Obara Citation2014). Based on the finding that growth under nitrogen-deficient conditions is positively correlated with root cortical aerenchyma formation, Postma and Lynch (Citation2011) proposed that aerenchyma formation may increase adaptation to low-nitrogen conditions by reducing respiration and enhancing nutrient mobilization.
2.2. Physiological response to nitrogen
A number of physiological processes are differentially influenced in distinct tissues by environmental nitrogen nutrient conditions or the intracellular nitrogen status (Nacry et al. Citation2013; Gastal et al. Citation2014; Vidal et al. Citation2014b). In this section, we discuss three physiological processes as typical and critical examples.
The first example is modulation of nitrogen uptake activity. Nitrogen-deficient conditions enhance the activity of both nitrate and ammonium uptake in barley (Lee and Rudge Citation1986). On the other hand, the application of nitrogen sources to plants growing in the absence of nitrate or under nitrogen-starved conditions transiently expands the nitrogen uptake potential by elevating the expression of genes involved in nitrate and ammonium uptake (Zhuo et al. Citation1999; Sonoda et al. Citation2003; Gu et al. Citation2013). These complex modulations may be a strategy to conserve energy and acquire nitrogen at the appropriate level in response to current nitrogen conditions and fluctuations. These physiological changes in the nitrogen uptake activity proceed within minutes or hours, whereas morphological changes of roots require several days. Accordingly, the physiological changes function in short-term adaptation, and root morphology changes may be more crucial during long-term adaptation to patchy nitrogen source distribution (Hodge Citation2004).
Another example of a physiological response is the mutual interplay between photosynthesis and nitrogen assimilation, which was indicated by the strong correlation between leaf nitrogen content and the CO2 assimilation rate (Makino Citation2011). The ribulose 1,5-bisphosphate carboxylase/oxygenase (Rubisco) content, which accounts for 20–50% of the total soluble protein in leaves (Xu et al. Citation2012), is strongly affected by the nitrogen supply (Makino and Osmond Citation1991). Conversely, photosynthesis provides energy and carbon skeletons required for nitrogen assimilation (Yanagisawa Citation2014; Griffiths et al. Citation2016). Nitrogen nutrients promote the expression of photosynthesis-related genes (Martin et al. Citation2002), whereas soluble sugars promote the expression of nitrate assimilation-related genes (Faure et al. Citation1994; Melo-Oliveira et al. Citation1996). This mutual interplay is the reason why nitrogen nutrition is crucial for biomass production and crop yield.
Nitrogen nutrition is presumed to affect the life cycle and the vegetative-to-reproductive stage transition in plants, although its effect on stage transition is controversial because of inconsistent results. Low-nitrogen conditions resulted in an earlier transition from vegetative to reproductive stage along with modifications in the expression levels of genes involved in flowering in Arabidopsis (Vidal et al. Citation2014b; Yuan et al. Citation2016) and rice (Williams and Angus Citation1994). However, opposite results were presented in independent studies with other crop species. The transition to the reproductive stage was advanced by nitrogen fertilizer application and delayed by low nitrogen availability in wheat and maize (Nerson et al. Citation1990; Fischer et al. Citation1993; Fischer Citation1993; Gungula et al. Citation2003). Conversely, a meta-analysis conducted on wheat and barley did not identify any clear correlation between nitrogen fertilizer application and days to flowering/heading (Hall et al. Citation2014). Therefore, nitrogen nutrient regulation of the reproductive stage transition has not been clarified. The reproductive stage transition might be determined in cooperation with other factors, such as the time of nitrogen application and the presence of other nutrients. The nitrate effect on flowering time is photoperiod-dependent in Arabidopsis. Under short-day conditions, low nitrate conditions promoted early flowering, whereas there was essentially no nitrate effect on flowering under long-day conditions (Marín et al. Citation2011). Currently, no model successfully integrates the experimental results on nitrogen nutrients and the transition to flowering.
3. Nitrate and ammonium as nitrogen sources
3.1. Distinct functions of nitrate and ammonium in the nitrogen response
Nitrate taken up by plants is first reduced in the cytosol by nitrate reductase to produce nitrite and then further reduced in chloroplasts by nitrite reductase to produce ammonium, before assimilation through the glutamine synthetase/glutamine oxoglutarate aminotransferase cycle (Miller and Cramer Citation2005; Yanagisawa Citation2014; Krapp Citation2015). These reduction reactions are energy-consuming, and plants are estimated to utilize up to 25% of their energy for nitrate assimilation (Bloom et al. Citation1989; Bloom Citation2015). Therefore, nitrate is a costlier nitrogen nutrient than ammonium. When both nitrate and ammonium are supplied in hydroponic cultures, plants favor ammonium uptake over nitrate (Sasakawa and Yamamoto Citation1978; Taylor and Bloom Citation1998; Gazzarrini et al. Citation1999). In upland conditions (i.e., non-flooded conditions), ammonium availability is low due to competition with soil microorganisms and nitrification by aerobic microorganisms (Li et al. Citation2016). Nitrate is the main nitrogen source for most plant species growing in upland conditions, where the nitrate:ammonium ratio reaches several dozen-fold (Miller et al. Citation2007). By contrast, the nitrate content is low in paddy fields due to its low electric potential and relative inactivity of nitrifying bacteria under hypoxic conditions. Ammonium is the predominant nitrogen source for plants growing in flooded conditions, where the ammonium:nitrate ratio in paddy fields can reach up to more than 10-fold (Li et al. Citation2016). Although both nitrogen sources can be utilized by plants, the ratio of ammonium to nitrate that maximizes the biomass production differs among plant species (Falkengren-Grerup Citation1995). A previous study showed that plant species adapted to acidic soils tend to prefer ammonium to nitrate, whereas those adapted to less acidic habitat prefer nitrate (Falkengren-Grerup Citation1995). Furthermore, it is also known that this preference is affected by a number of environmental factors such as temperature, radiation, soil moisture, and soil pH (detailed in Britto and Kronzucker Citation2013).
Nitrate and ammonium induce different responses in plants. Nitrate dramatically induces genome-wide changes in gene expression patterns in both aerial and subterranean plant organs (discussed in the next section). By contrast, ammonium application stimulates much lower levels of transcriptional reprogramming (Patterson et al. Citation2010) and primarily induces the expression of genes encoding AMTs and ammonium assimilation enzymes (GS1;2 and NADH-GOGAT) (Sonoda et al. Citation2003; Konishi et al. Citation2014; Chandran et al. Citation2016). Although the ammonium-induced response mechanisms have not been fully elucidated, some of ammonium-induced gene expression, including that of rice OsAMT1;1 and OsAMT1;2, is likely caused by an increase in glutamine (a downstream metabolite), because they were inhibited by the treatment with methionine sulfoximine, a glutamine synthetase inhibitor (Sonoda et al. Citation2003). Another report showed that the removal of ammonium from the culture solution had essentially no immediate effects on transcription, whereas removal of nitrate drastically changed gene expression levels in Arabidopsis (Menz et al. Citation2016). These combined results suggest that the internal nitrogen status may be more important than the presence of external ammonium in ammonium-induced response.
3.2. Synergistic effects of nitrate and ammonium in rice
Despite the dominance of ammonium in flooded soils, a few studies already revealed that the presence of nitrate positively affects the growth of rice. The substitution of ammonium with ammonium nitrate stimulated biomass production in both indica and japonica cultivars that were hydroponically grown, indicating that nitrate and ammonium synergistically promote biomass production (Chanh et al. Citation1981; Chanh and Ohira Citation1981). This enhanced biomass production is not simply explained by nitrate-mediated induction of nitrogen assimilation-related gene expression. Li et al. (Citation2006) observed that expression of the nitrate reductase gene OsNR1 in rice was promoted more strongly by ammonium nitrate than by either nitrate or ammonium alone. Similarly, the expression of OsAMT1;3 in roots and the ammonium concentration in leaves were higher in rice grown with ammonium nitrate than with nitrate or ammonium alone. Duan et al. (Citation2007) proposed that higher biomass production, grain yield, and ammonium uptake in the presence of both ammonium and nitrate could result from the enhanced expression of AMT1 family genes. Therefore, the presence of both nitrate and ammonium may optimally stimulate the nitrogen assimilation pathway.
Nitrate and ammonium uptake cause cytosolic acidification and alkalinization, respectively (Britto and Kronzucker Citation2005; Fan et al. Citation2016), which may be related to changes in cytosolic pH. Stability of cytosolic pH might be important for the influx and assimilation of both ions (Fan et al. Citation2016). Xu et al. (Citation2012) proposed that the pH adjustment by proton-coupled nitrate transport underlies their synergistic effect. Future work designed to elucidate this synergistic effect more precisely could help use it to improve the biomass and yield in rice.
3.3. Roles of nitrate in rice growing under flooded conditions
Despite low availability of oxygen in flooded soils, studies revealed that the oxygen supply via aerenchyma and the barrier to radial oxygen loss form a partially aerobic environment in the root surface vicinity, especially close to the root tip in rice (Rubinigg et al. Citation2002; Colmer Citation2003; Watanabe et al. Citation2013). Nitrifying bacteria (e.g., Nitrosomonas spp. and Nitrobacter spp.; Miller and Cramer Citation2005) become active under these conditions, and ammonium can be oxidized to nitrate. As this nitrate is produced within 1–2 mm from the root surface (Arth and Frenzel Citation2000; Kirk Citation2001), the nitrate should be immediately absorbed by plants. One model suggested that more than 30% of the total nitrogen uptake by lowland rice was in the form of nitrate (Kirk and Kronzucker Citation2005).
The importance of nitrate in rice cultivation was also indicated by a search for traits associated with high nitrogen-use efficiency (NUE). A study subjecting plants to flooded soil revealed that the high NUE of a rice cultivar Yangdao 6 was associated with enhanced aerenchyma development, enhanced oxygen emission from the root surface (radial oxygen loss), higher nitrification activity in the rhizosphere soil, and higher total nitrogen uptake as compared with Nongken 57, which undergoes less aerenchyma formation (Li et al. Citation2008). Cultivation of Yangdao 6 increased the rhizospheric nitrate content, and the nitrate-inducible nitrate reductase activity in leaves of Yangdao 6 was higher than that in Nongken 57. Hence, it was proposed that the enhanced nitrogen uptake activity of Yangdao 6 was caused by enhanced nitrate production on the root surface (Li et al. Citation2008). Enhanced aerenchyma formation under nitrogen starvation might have evolved to increase the available nitrate resources and thereby improve growth. Because of the positive aspect of nitrate nutrition on rice in the field (), nitrate nutrition could be a key to more effective fertilizer application and improvement of NUE.
Figure 2. Importance of nitrate as a nitrogen source in lowland rice. (a) Hypothetical scheme of nitrate utilization in rice grown under flooded conditions. Oxygen is supplied to the root from the shoot through aerenchyma. Conveyed oxygen is diffused into the rhizosphere and produces locally aerobic conditions. Then, it activates bacteria involved in nitrification, and the microbes produce nitrate, which is immediately taken up by plants via nitrate transporters. (b) Selection for nitrate transporters (NRTs) during indica rice domestication and modern breeding. Plants displayed with NRT alleles with better function (hexagons) were selected over the unfavorable ones (triangles), possibly due to their effects on nitrogen utilization, biomass production, and grain yield.
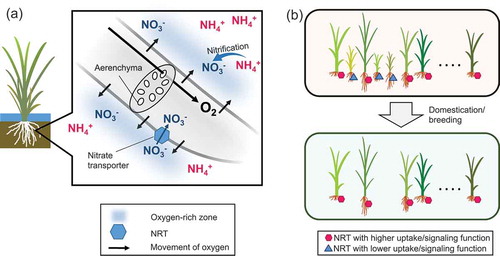
The physiological relevance of nitrate for growth in rice was also revealed in studies of rice homologs of Arabidopsis genes encoding the high-affinity nitrate transporter NRT2 and its associated protein, NITRATE ASSIMILATION-RELATED 2 (NAR2), which is necessary for NRT2 activity (Cai et al. Citation2008; Liu et al. Citation2014). Reduced expression of nitrate-inducible OsNAR2.1 (one of NAR2 genes) in RNA interference lines led to reduced biomass production in a pot experiment under flooded conditions (Yan et al. Citation2011). By contrast, transgenic rice plants expressing the nitrate transporter gene OsNRT2.1 under the control of the OsNAR2.1 promoter displayed higher grain yields, increased nitrogen contents, and enhanced nitrogen translocation to the panicles (Chen et al. Citation2016). Hu et al. (Citation2015) also showed that an allele of OsNRT1.1B encoding a nitrate transporter harboring higher nitrate uptake activity due to an amino acid substitution was actively selected during the domestication of indica rice. It is also known that modern breeders have selected for genes associated with nitrate uptake and assimilation, such as OsNRT2.3, OsNAR2.2, and OsNiR1 (nitrite reductase gene), as well as ammonium transporter (OsAMT1;1) loci during the breeding of indica rice in flooded areas (Ding et al. Citation2011; GRiSP Citation2013; Xie et al. Citation2015) (). These studies suggest that these loci might be important for better growth of rice in paddy fields.
Nitrate may have dual functions as a nitrogen source and as a signaling molecule even in rice plants growing under flooded conditions. Recent works in Arabidopsis reported a nitrate-responsive mechanism in which master transcription factors [NODULE INCEPTION (NIN)-like proteins (NLPs)] bind to their cognate nitrate-responsive cis-element (NRE) DNA sequences and initiate nitrate-inducible gene expression (described in the next section). There are NLP genes in the rice genome (Schauser et al. Citation2005), and mutation of the NRE-like sequence in the OsNAR2.1 promoter attenuated nitrate-inducible promoter activity in rice (Liu et al. Citation2015), indicating that rice also possesses the NLP-mediated mechanism for nitrate-responsive gene expression. Taken together, rice can effectively use nitrate as a nitrogen source under the flooded conditions to promote growth and also as a signaling molecule to induce gene expression reprogramming.
4. Mechanism underlying nitrate signaling and response
Nitrate supply to nitrate-starved plants was found to induce nitrate reductase activity and gene expression in several plant species more than half a century ago (Tang and Wu Citation1957; Hewitt and Afridi Citation1959; Hageman and Flesher Citation1960; Cheng et al. Citation1986; Lahners et al. Citation1988; Gowri and Campbell Citation1989). Recent transcriptome analyses subsequently revealed that nitrate rapidly modifies the expression of numerous (>1000) genes, including genes involved in nitrate transport and assimilation, genes involved in carbon, iron, and sulfur metabolism, and genes with regulatory functions (Wang et al. Citation2000, Citation2003; Scheible et al. Citation2004), implying that the primary nitrate response induces the genome-wide modification of gene expression patterns. In this nitrate-induced modification, nitrate, but not downstream nitrogen metabolites, presumably acts as signaling molecule, because nitrate reductase mutants that could not metabolize nitrate still responded to nitrate supply in much the same way as wild-type plants (Pouteau et al. Citation1989; Wang et al. Citation2004). This section summarizes the current knowledge of the molecular mechanisms underlying nitrate signaling and response.
4.1. NLP transcription factors are key regulators of the nitrate response
Recent analyses have shed light on the molecular mechanism mediating nitrate-induced gene expression in Arabidopsis and identified several regulatory genes encoding transcription factors and protein kinases as factors involved in nitrate-responsive gene expression. NLPs, which are structurally very similar to NIN in Lotus japonicus, are currently modeled as the primary transcription factors for nitrate signaling and response. Although NIN is an essential protein for nodulation in legumes (Schauser et al. Citation1999) and functions as a transcription factor (Soyano et al. Citation2013; Suzuki et al. Citation2013), NLPs are present in both leguminous and non-leguminous plants. Although the function of NLPs was a mystery until recently, NLPs have been revealed as the primary transcription factors for nitrate-inducible gene expression (Konishi and Yanagisawa Citation2013a). They bind to NRE sequences in loci containing nitrate-inducible genes and promote their expression. Plants contain several copies of NLP genes, and Arabidopsis, L. japonicus, and rice contain 9, 4, and 6 NLP genes, respectively (Schauser et al. Citation2005; Suzuki et al. Citation2013; Chardin et al. Citation2014). All NLPs share the following three conserved domains: the RWP-RK domain in the middle, a novel conserved domain in the amino-terminal region, and the PB1 (Phox Bem 1) domain in the carboxy-terminal region (). The RWP-RK domain, named after its invariant amino acid sequence Arg-Trp-Pro-X-Arg-Lys (X for any amino acid), is the DNA-binding domain that binds to NREs (Schauser et al. Citation2005; Konishi and Yanagisawa Citation2013a, Citation2013b, Citation2014). The novel conserved domain in the N-terminal region is a nitrate-responsive domain, because fusing this domain of Arabidopsis NLPs converted an unrelated DNA-binding protein into a nitrate-responsive transcription factor (Konishi and Yanagisawa Citation2013a; Konishi and Yanagisawa unpublished data). NIN, which possesses mutations in this domain, is a nitrate-insensitive transcription factor (Suzuki et al. Citation2013). The PB1 domain, which was originally found in the mammalian phagocyte oxidase activator p67phox protein and the yeast’s polarity establishment protein Bem1, generally mediates protein–protein interactions (Sumimoto et al. Citation2007). Recently, it was shown that the PB1 domains of NLP6 and NLP7 are also protein–protein interaction domains mediating NLP homo- and hetero-dimerization and complex formation with other transcription factors (Guan et al. Citation2017).
Figure 3. Molecular mechanism of nitrate-induced gene expression. (a) Structure of Arabidopsis NLP7. NLP proteins contain an amino-terminal conserved domain (indicated by dark gray), a DNA-binding RWP-RK domain, and a PB1 domain. The amino-terminal region to the RWP-RK domain is nitrate responsive. The conserved serine residue (Ser 205) of NLP7 is phosphorylated in response to nitrate supply. (b) Without nitrate, NLP7 is inactive and mostly resides in the cytosol due to active export out of the nucleus. Nitrate enters into cells through nitrate transporters (NRT1.1 and NRT2). Calcium-dependent protein kinases (CPK10, CPK30, and CPK32) are located at the plasma membrane, but nitrate supply stimulates their translocation to the nucleus and their activation through elevated cytosolic Ca2+. Ca2+-bound CPKs interact with NLP7 inside the nucleus and phosphorylate the conserved serine residue, leading to NLP7 accumulation in the nucleus. Activated NLP7 binds to the cognate DNA sequences (nitrate-responsive elements [NREs]) and promotes transcription of target genes, including NIA1 (nitrate reductase gene), NITR2 (plastidic nitrite transporter gene, Maeda et al. Citation2014), and NIR1 (nitrite reductase gene). The presence of a bifurcating pathway for nitrate signaling is also implicated.
![Figure 3. Molecular mechanism of nitrate-induced gene expression. (a) Structure of Arabidopsis NLP7. NLP proteins contain an amino-terminal conserved domain (indicated by dark gray), a DNA-binding RWP-RK domain, and a PB1 domain. The amino-terminal region to the RWP-RK domain is nitrate responsive. The conserved serine residue (Ser 205) of NLP7 is phosphorylated in response to nitrate supply. (b) Without nitrate, NLP7 is inactive and mostly resides in the cytosol due to active export out of the nucleus. Nitrate enters into cells through nitrate transporters (NRT1.1 and NRT2). Calcium-dependent protein kinases (CPK10, CPK30, and CPK32) are located at the plasma membrane, but nitrate supply stimulates their translocation to the nucleus and their activation through elevated cytosolic Ca2+. Ca2+-bound CPKs interact with NLP7 inside the nucleus and phosphorylate the conserved serine residue, leading to NLP7 accumulation in the nucleus. Activated NLP7 binds to the cognate DNA sequences (nitrate-responsive elements [NREs]) and promotes transcription of target genes, including NIA1 (nitrate reductase gene), NITR2 (plastidic nitrite transporter gene, Maeda et al. Citation2014), and NIR1 (nitrite reductase gene). The presence of a bifurcating pathway for nitrate signaling is also implicated.](/cms/asset/1fc7a11d-e1db-40a7-9eb2-3e184a1f47ba/tssp_a_1360128_f0003_oc.jpg)
Several lines of evidence indicate that NLP transcription factors are key regulators of the nitrate response: (1) the Arabidopsis nlp7 mutant is globally impaired in nitrate-stimulated gene expression (Marchive et al. Citation2013); (2) NLP6 activity is post-translationally induced by nitrate, indicating that NLP6 encodes a transcription factor involved in the primary response to nitrate (Konishi and Yanagisawa Citation2013a); (3) Arabidopsis NLP6 fused to a transcriptional repressor domain globally inhibited nitrate-induced gene expression (Konishi and Yanagisawa Citation2014); and (4) genome-wide analysis of in vivo NLP7-binding sites in Arabidopsis showed that nitrate supply promoted NLP7 binding to loci associated with nitrate metabolism and signaling (Marchive et al. Citation2013). These combined results suggest that NLP transcription factors orchestrate nitrate-responsive gene expression.
4.2. Mechanism of post-translational induction of NLP activity
The key process for nitrate signaling and nitrate-induced gene expression is post-translational induction of NLP activity, because NLPs are present as the primary transcription factors for the nitrate response before nitrate signaling. Recent studies are beginning to elucidate the mechanism of post-translational regulation of NLP transcription factor activity (). The first study observed nitrate-dependent nuclear accumulation of Arabidopsis NLP7 in the nucleus (Marchive et al. Citation2013). NLP7 fused to green fluorescent protein rapidly accumulates in the nucleus after application of nitrate, and this nuclear accumulation was reversed when nitrate was withdrawn. The presence of a nuclear export inhibitor promoted the nuclear accumulation of NLP7, suggesting that the nuclear retention of NLPs in the presence of nitrate is a crucial part of the nitrate signaling mechanism (). The second study observed Ca2+-dependent NLP phosphorylation and Ca2+-dependent induction of NLP activity (Liu et al. Citation2017a). Arabidopsis NLP6 and NLP7 were immediately phosphorylated by CPK10, 30, and 32 (some of Arabidopsis calcium-dependent protein kinases [CPKs]) in response to nitrate application, and this phosphorylation was inhibited by Ca2+ channel blockers and Ca2+ sensor inhibitors. The phosphorylated amino acid residue was a serine within the nitrate-responsive domain (), which is conserved in all NLPs but not in NIN. A mutation of this serine residue in NLP7 (205th residue) to alanine abolished the nitrate-inducible nuclear accumulation of NLP7 and largely reduced NLP7-dependent transactivation. Consistent with the loss of activity, expression of the mutant NLP7 no longer complemented the nlp7 mutant phenotype. The cpk10 cpk30 cpk32 triple mutant displayed a nitrate-insensitive phenotype, indicating the central role of NLP phosphorylation in the nitrate response. Although CPK10, CPK30, and CPK32 are probably N-myristoylated and associate at the plasma membrane in the absence of nitrate signaling, similar to other CPKs (Zou et al. Citation2010; Boudsocq and Sheen Citation2013; Liu et al. Citation2017a), some percentage of the CPKs was translocated into the nucleus and interacted with NLP7 in response to nitrate application (Liu et al. Citation2017a). Based on these findings, a current model for nitrate signaling includes Ca2+ signaling, NLP phosphorylation, NLP nuclear accumulation, and subsequent NRE-dependent activation of a number of nitrate-responsive genes ().
An outline of the molecular mechanism for nitrate signaling is emerging. However, there are still several unclarified questions. First, neither the elevated Ca2+ concentration nor the constitutive activation of CPKs was sufficient for NLP-mediated activation of nitrate-inducible gene expression (Sakakibara et al. Citation1997; Liu et al. Citation2017a), suggesting that an additional pathway needs to work cooperatively with the Ca2+–CPK pathway. Second, Arabidopsis NLP8 is constitutively localized to the nucleus, although it is capable of nitrate-dependent transactivation via its N-terminal region (Yan et al. Citation2016). The phosphorylated serine residue is also conserved (Liu et al. Citation2017a). Thus, another mechanism is needed to regulate NLP8 activity. Finally, the connection between NLP-mediated gene expression and the sensor function of the nitrate transporter NRT1.1 also remains elusive. NRT1.1 was proposed as a nitrate sensor because its effect on nitrate-responsive expression of several genes (NRT2.1, NIA1, NIA2, and NRT1.1) could be decoupled from its nitrate uptake activity by exploiting a unique nrt1.1 mutant allele (Ho et al. Citation2009). However, the expression of some nitrate-inducible genes and the nitrate-induced Ca2+ increase were not regulated by this sensor activity (Vidal et al. Citation2014a; Alvarez et al. Citation2014; Riveras et al. Citation2015). Taken together with the fact that NRT1.1 also transports auxin and modulates lateral root growth independently of nitrate-responsive gene expression (Krouk et al. Citation2010), the connection between NRT1.1 and NLP-mediated gene expression needs to be carefully verified.
4.3. Similarities and differences in the physiological roles of Arabidopsis NLPs
All Arabidopsis NLPs analyzed are transcriptional activators that are post-transcriptionally regulated by nitrate (Konishi et al. unpublished data). However, it is arguable whether all NLPs have similar physiological functions. The Arabidopsis nlp7 mutants displayed reduced vegetative growth and gene expression profiles similar to those of nitrogen-starved plants (Castaings et al. Citation2009; Marchive et al. Citation2013). Disruption of NLP6 alone (the gene encoding an Arabidopsis NLP protein that is most similar to NLP7) did not affect vegetative growth, but the nlp6 mutation greatly enhanced the effects of the nlp7 mutation (Guan et al. Citation2017; Konishi et al. unpublished data). Thus, NLP6 and NLP7 appear to redundantly regulate growth during the vegetative stage. In contrast, Arabidopsis NLP8 was recently reported to have different functions. NLP8 was highly expressed during seed imbibition, and the NLP8 protein that was activated by nitrate bound to the promoter of CYP707A2 and stimulated expression of this gene (Yan et al. Citation2016). Because CYP707A2 is an abscisic acid (ABA) 8′-hydoroxylase that degrades ABA during seed germination (Kushiro et al. Citation2004; Okamoto et al. Citation2006; Matakiadis et al. Citation2009), NLP8 triggered nitrate-dependent promotion of seed germination by reducing the ABA level. This result is consistent with the previous observation that nitrate treatment promoted the germination of dormant seeds in several plant species by the action of nitrate, but not by downstream metabolites (Steinbauer and Grigsby Citation1957; Fawcett and Slife Citation1978; Batak et al. Citation2002; Alboresi et al. Citation2005). Interestingly, NLP7 expression under control of the 35S promoter could not complement the nlp8 mutant, unlike NLP8 (Yan et al. Citation2016), suggesting a unique role of NLP8.
Nitrate supply evokes a variety of metabolic and physiological changes, such as increases in cytokinin levels, organic acid accumulation, lateral root elongation, increases in shoot:root ratios, and increases in leaf growth (Scheible et al. Citation1997a; Citation1997b; Zhang and Forde Citation1998; Vidal et al. Citation2010; Walch-Liu et al. Citation2000; Takei et al. Citation2002, Citation2004). The unique function of NLP8 suggests that some NLPs may have unique roles in different physiological processes, whereas others may have redundant roles in the same physiological processes.
5. Coordination of nitrogen and phosphorus responses
Nitrogen source levels affect uptake of other nutrients. For instance, the increasing nitrate concentration in the culture medium promoted the uptake of potassium, magnesium, and calcium in wheat, whereas high concentrations of ammonium had adverse effects, probably due to ionic competition (Cox and Reisenauer Citation1973). Furthermore, phosphate uptake was upregulated by supply of nitrogen sources in wheat (Cox and Reisenauer Citation1973), while phosphate uptake was lower under nitrogen-limited conditions in maize (Smith and Jackson Citation1987a, Citation1987b). Similarly, nitrogen response is not only modulated by nitrogen availability but also influenced by responses to other mineral nutrients, such as phosphorus. This was shown by earlier physiological studies in maize, where phosphorus availability positively affected the uptake rate of nitrogen sources (de Magalhães et al. Citation1998, Citation2000). Recent studies elucidated the mechanisms coordinating the nitrogen response with the responses regulated by the availability of phosphorus.
NITROGEN LIMITATION ADAPTATION (NLA) is an Arabidopsis ubiquitin E3 ligase that mediates degradation of the plasma membrane-localized phosphate transporter PHT1;4 and thus negatively regulates phosphate uptake (Peng et al. Citation2007; Kant et al. Citation2011; Park et al. Citation2014). NLA was also proposed to degrade the phloem-localized nitrate transporter NRT1.7 (Liu et al. Citation2017b), which participates in nitrate remobilization from source organs to sink organs (Fan et al. Citation2009). The Arabidopsis nla mutant translocated more nitrate from old leaves to young leaves than wild-type plants (Liu et al. Citation2017b). Taken together with the suppression of NLA expression by low phosphate availability and reduction in the NLA protein level under nitrogen-deficient conditions (Kant et al. Citation2011; Liu et al. Citation2017b), NLA activity under the control of both nitrogen and phosphorus availability may contribute to the distribution and homeostasis of both nitrogen and phosphorus.
A small ubiquitin-related modifier (SUMO) E3 ligase in Arabidopsis (AtSIZ1) and its homolog in rice (OsSIZ1) are factors that affect both nitrogen and phosphorus homeostasis (Miura et al. Citation2005; Park et al. Citation2011; Wang et al. Citation2015). AtSIZ1 covalently attaches the SUMO protein to nitrate reductase to enhance its stability and activity. Consistently, the Arabidopsis siz1 mutant displayed reduced biomass production in the presence of nitrate as sole nitrogen source (Park et al. Citation2011). Thus, although AtSIZ1 was originally isolated from a mutant line exhibiting enhanced sensitivity to phosphorus deficiency (Miura et al. Citation2005), AtSIZ1 has broader functions in nutrient uptake and utilization, in addition to previously suggested roles in hormonal regulation and abiotic stress tolerance (Miura et al. Citation2011, Citation2013). However, the following results obtained with Arabidopsis and rice mutants were inconsistent and remain to be elucidated. The nitrogen content was lower in the Arabidopsis siz1 mutant, consistent with its reduced nitrate reductase activity (Park et al. Citation2011), whereas the nitrogen and phosphorus contents were higher in rice knockout mutants of OsSIZ1, which also displayed shorter primary root length and reduced biomass (Wang et al. Citation2015).
HYPERSENSITIVITY TO LOW PI-ELICITED PRIMARY ROOT SHORTENING1 (HRS1) and its closest homolog, HRS1 HOMOLOG 1, are Arabidopsis nitrate-inducible genes involved in the suppression of primary root growth in the absence of phosphate (Liu et al. Citation2009; Medici et al. Citation2015). This inhibitory effect on root growth is more dramatic when nitrate-containing medium is used (Medici et al. Citation2015). On the other hand, the rice homolog [NITRATE-INDUCIBLE GARP-TYPE TRANSCRIPTIONAL REPRESSOR1 (OsNIGT1)] was identified as an early nitrate-responsive gene encoding a transcriptional repressor, and overexpression of NIGT1 reduced the chlorophyll content in rice seedlings only when they were grown with nitrate as sole nitrogen source (Sawaki et al. Citation2013). Therefore, Arabidopsis HRS1 and rice NIGT1 are nitrate-inducible transcription factors that potentially control both nitrate and phosphate responses.
Another factor involved in the coordination of nitrogen and phosphorus responses is a rice microRNA, miR444a. miR444a expression was induced by nitrogen and phosphorus limitation, and miR444a-overexpressing rice had higher nitrate and phosphate contents, along with increased expression levels of both nitrate transporter genes and phosphate transporter genes (Yan et al. Citation2014). Overexpression of miR444a rendered plants insensitive to applied nitrate and phosphate starvation in terms of lateral root formation, further implicating its role in both nitrogen and phosphorus homeostasis.
6. Conclusions and perspectives
Plants deploy multiple layers of sensing and adaptive mechanisms to perceive and respond to fluctuating nitrogen nutrient conditions in the soil. Numerous studies have shed light on these complex responses and molecular mechanisms during the past few decades. The nitrogen response involves many factors and is mutually influenced by the responses to other mineral nutrients. These mechanisms should underlie the fine tuning of nitrogen homeostasis under the fluctuating environments.
Recent increases in crop productivity owe a lot to increased application of nitrogen fertilizers and genetic enhancement of nitrogen uptake capacity. From an agricultural point of view, there is increasing demand for efficient nitrogen use in crop species to reduce fertilizer application and its associated environmental pollution. A fundamental understanding of the nitrogen response and its regulatory mechanisms would furnish new insights into the design of crops with higher NUE. These mechanisms have been uncovered primarily in the model plant Arabidopsis, and it has not been determined if they are widely conserved in the plant kingdom. Future investigations of the mechanisms regulating nitrogen responses in crops will be necessary to determine how the current knowledge can be translated into applications in crop production. Although several intracellular nitrogen metabolites likely affect the nitrogen response, such as root architecture and the expression levels of NRT and AMT genes (Nacry et al. Citation2013), little is known about the potential regulation of the nitrogen response by nitrogen-containing organic metabolites. Although a large quantity of nitrogen is applied in the form of urea to agricultural fields, urea transport and plant responses to urea have barely been characterized. Further investigations of the nitrogen response in both model plants and crops are warranted to answer these questions and to reveal more details regarding the nitrogen response mechanisms in plants for future agricultural applications.
Acknowledgments
This work was supported in part by JST CREST: [Grant Number JPMJCR15O5] and JSPS KAKENHI Scientific Research: [Grant Numbers 25252014, 26221103, and 15H05616]. We apologize to the authors of many publications that we were unable to cite due to space constraints.
Additional information
Funding
References
- Abiko T, Obara M 2014: Enhancement of porosity and aerenchyma formation in nitrogen-deficient rice roots. Plant Sci., 215–216, 76–83. doi:10.1016/j.plantsci.2013.10.016
- Alboresi A, Gestin C, Leydecker M-T, Bedu M, Meyer C, Truong H-N 2005: Nitrate, a signal relieving seed dormancy in Arabidopsis. Plant, Cell Environ., 28, 500–512. doi:10.1111/pce.2005.28.issue-4
- Alvarez JM, Riveras E, Vidal EA et al. 2014: Systems approach identifies TGA1 and TGA4 transcription factors as important regulatory components of the nitrate response of Arabidopsis thaliana roots. Plant J., 80, 1–13. doi:10.1111/tpj.12618
- Arth I, Frenzel P 2000: Nitrification and denitrification in the rhizosphere of rice: the detection of processes by a new multi-channel electrode. Biol. Fertil. Soils, 31, 427–435. doi:10.1007/s003749900190
- Batak I, Dević M, Giba Z, Grubisić D, Poff KL, Konjević R 2002: The effects of potassium nitrate and NO-donors on phytochrome A- and phytochrome B-specific induced germination of Arabidopsis thaliana seeds. Seed Sci. Res., 12, 253–259. doi:10.1079/SSR2002118
- Bloom AJ 2015: The increasing importance of distinguishing among plant nitrogen sources. Curr. Opin. Plant Biol., 25, 10–16. doi:10.1016/j.pbi.2015.03.002
- Bloom AJ, Caldwell RM, Finazzo J, Warner RL, Weissbart J 1989: Oxygen and carbon dioxide fluxes from barley shoots depend on nitrate assimilation. Plant Physiol., 91, 352–356. doi:10.1104/pp.91.1.352
- Boudsocq M, Sheen J 2013: CDPKs in immune and stress signaling. Trends Plant Sci., 18, 30–40. doi:10.1016/j.tplants.2012.08.008
- Bouguyon E, Perrine-Walker F, Pervent M et al. 2016: Nitrate controls root development through posttranscriptional regulation of the NRT1.1/NPF6.3 transporter/sensor. Plant Physiol., 172, 1237–1248.
- Brien JAO, Vega A, Bouguyon E, Krouk G, Gojon A, Coruzzi G, Gutiérrez RA 2016: Nitrate transport, sensing and responses in plants. Mol. Plant, 9, 837–856. doi:10.1016/j.molp.2016.05.004
- Britto DT, Kronzucker HJ 2005: Nitrogen acquisition, PEP carboxylase, and cellular pH homeostasis: new views on old paradigms. Plant, Cell Environ., 28, 1396–1409. doi:10.1111/pce.2005.28.issue-11
- Britto DT, Kronzucker HJ 2013: Ecological significance and complexity of N-source preference in plants. Ann. Bot., 112, 957–963. doi:10.1093/aob/mct157
- Cai C, Wang J, Zhu Y, Shen Q, Li B, Tong Y, Li Z-S 2008: Gene structure and expression of the high-affinity nitrate transport system in rice roots. J. Integr. Plant Biol., 50, 443–451. doi:10.1111/j.1744-7909.2008.00642.x
- Castaings L, Camargo A, Pocholle D et al. 2009: The nodule inception-like protein 7 modulates nitrate sensing and metabolism in Arabidopsis. Plant J., 57, 426–435. doi:10.1111/j.1365-313X.2008.03695.x
- Chandran AKN, Priatama RA, Kumar V, Xuan Y, Il JB, Kim CM, Jung KH, Han CD 2016: Genome-wide transcriptome analysis of expression in rice seedling roots in response to supplemental nitrogen. J. Plant Physiol., 200, 62–75. doi:10.1016/j.jplph.2016.06.005
- Chanh TT, Ohira K 1981: Effects of various environmental and medium conditions on the response of Indica and Japonica rice plants to ammonium and nitrate nitrogen. Soil Sci. Plant Nutr., 27, 347–355. doi:10.1080/00380768.1981.10431257
- Chanh TT, Tsutsumi M, Kurihara K 1981: Comparative study on the response of Indica and Japonica rice plants to ammonium and nitrate nitrogen. Soil Sci. Plant Nutr., 27, 83–92. doi:10.1080/00380768.1981.10431257
- Chardin C, Girin T, Roudier F, Meyer C, Krapp A 2014: The plant RWP-RK transcription factors: key regulators of nitrogen responses and of gametophyte development. J. Exp. Bot., 65, 5577–5587. doi:10.1093/jxb/eru261
- Chen J, Zhang Y, Tan Y, Zhang M, Zhu L, Xu G, Fan X 2016: Agronomic nitrogen-use efficiency of rice can be increased by driving OsNRT2.1 expression with the OsNAR2.1 promoter. Plant Biotechnol. J., 14, 1705–1715. doi:10.1111/pbi.2016.14.issue-8
- Cheng C-L, Dewdney J, Kleinhofs A, Goodman HM 1986: Cloning and nitrate induction of nitrate reductase mRNA. Proc. Natl. Acad. Sci. USA, 83, 6825–6828. doi:10.1073/pnas.83.18.6825
- Chun L, Mi G, Li J, Chen F, Zhang F 2005: Genetic analysis of maize root characteristics in response to low nitrogen stress. Plant Soil, 276, 369–382. doi:10.1007/s11104-005-5876-2
- Clarke AL, Barley KP 1968: The uptake of nitrogen from soils in relation to solute diffusion. Aust. J. Soil Res., 6, 75–92. doi:10.1071/SR9680075
- Colmer TD 2003: Aerenchyma and an inducible barrier to radial oxygen loss facilitate root aeration in upland, paddy and deep-water rice (Oryza sativa L.). Ann. Bot., 91, 301–309. doi:10.1093/aob/mcf114
- Cox WJ, Reisenauer HM 1973: Growth and ion uptake by wheat supplied nitrogen as nitrate, or ammonium, or both. Plant Soil, 38, 363–380. doi:10.1007/BF00779019
- de Magalhães JV, Alves VMC, de Novais RF, Mosquim PR, Magalhães JR, Bahia Filho AFC, Huber DM 1998: Nitrate uptake by corn under increasing periods of phosphorus starvation. J. Plant Nutr., 21, 1753–1763. doi:10.1080/01904169809365520
- de Magalhães JV, Alves VMC, de Novais RF, Mosquim PR, Magalhães JR, Bahia Filho AFC, Huber DM 2000: Influence of phosphorus stress on ammonium uptake by maize. J. Plant Nutr., 23, 263–273. doi:10.1080/01904160009382013
- Ding Z, Wang C, Chen S, Yu S 2011: Diversity and selective sweep in the OsAMT1;1 genomic region of rice. BMC Evol. Biol., 11, 61. doi:10.1186/1471-2148-11-61
- Drew MC 1975: Comparison of the effects of a localized supply of phosphate, nitrate, ammonium and potassium on the growth of the seminal root system, and the shoot, in barley. New Phytol., 75, 479–490. doi:10.1111/j.1469-8137.1975.tb01409.x
- Drew MC, He C-J, Morgan PW 2000: Programmed cell death and aerenchyma formation in roots. Trends Plant Sci., 5, 123–127. doi:10.1016/S1360-1385(00)01570-3
- Duan YH, Zhang YL, Ye LT, Fan XR, Xu GH, Shen QR 2007: Responses of rice cultivars with different nitrogen use efficiency to partial nitrate nutrition. Ann. Bot., 99, 1153–1160. doi:10.1093/aob/mcm051
- Echevarría-Zomeño S, Fernández-Calvino L, Castro-Sanz AB, López JA, Vázquez J, Castellano MM 2016: Dissecting the proteome dynamics of the early heat stress response leading to plant survival or death in Arabidopsis. Plant, Cell Environ., 39, 1264–1278. doi:10.1111/pce.12664
- Erisman JW, Sutton MA, Galloway J, Klimont Z, Winiwarter W 2008: How a century of ammonia synthesis changed the world. Nat. Geosci., 1, 636–639. doi:10.1038/ngeo325
- Falkengren-Grerup U 1995: Interspecies differences in the preference of ammonium and nitrate in vascular plants. Oecologia, 102, 305–311. doi:10.1007/BF00329797
- Fan S-C, Lin C-S, Hsu P-K, Lin S-H, Tsay Y-F 2009: The Arabidopsis nitrate transporter NRT1.7, expressed in phloem, is responsible for source-to-sink remobilization of nitrate. Plant Cell, 21, 2750–2761. doi:10.1105/tpc.109.067603
- Fan X, Tang Z, Tan Y, Zhang Y, Luo B, Yang M, Lian X, Shen Q, Miller AJ, Xu G 2016: Overexpression of a pH-sensitive nitrate transporter in rice increases crop yields. Proc. Natl. Acad. Sci. USA, 113, 7118–7123. doi:10.1073/pnas.1525184113
- Faure J-D, Jullien M, Caboche M 1994: Zea3: a pleiotropic mutation affecting cotyledon development, cytokinin resistance and carbon-nitrogen metabolism. Plant J., 5, 481–491. doi:10.1046/j.1365-313X.1994.5040481.x
- Fawcett RS, Slife FW 1978: Effects of field applications of nitrate on weed seed germination and dormancy. Weed Sci., 26, 594–596.
- Fischer RA 1993: Irrigated spring wheat and timing and amount of nitrogen fertilizer. II. Physiology of grain yield response. Field Crop Res., 33, 57–80. doi:10.1016/0378-4290(93)90094-4
- Fischer RA, Howe GN, Ibrahim Z 1993: Irrigated spring wheat and timing and amount of nitrogen-fertilizer. I. Grain yield and protein content. Field Crop Res., 33, 37–56. doi:10.1016/0378-4290(93)90093-3
- Forde BG 2014: Nitrogen signalling pathways shaping root system architecture: an update. Curr. Opin. Plant Biol., 21, 30–36. doi:10.1016/j.pbi.2014.06.004
- Gallardo A, Paramá R, Covelo F 2006: Differences between soil ammonium and nitrate spatial pattern in six plant communities. Simulated effect on plant populations. Plant Soil, 279, 333–346. doi:10.1007/s11104-005-8552-7
- Gastal F, Lemaire G, Durand JL, Louarn G 2014: Quantifying crop responses to nitrogen and avenues to improve nitrogen-use efficiency. In Crop Physiology – Applications for Genetic Improvement and Agronomy, Ed. Sadras VO, Calderini D, pp. 161–206. Elsevier Academic Press, San Diego, CA.
- Gazzarrini S, Lejay L, Gojon A, Ninnemann O, Frommer WB, von Wirén N 1999: Three functional transporters for constitutive, diurnally regulated, and starvation-induced uptake of ammonium into Arabidopsis roots. Plant Cell, 11, 937–947. doi:10.1105/tpc.11.5.937
- Giehl RFH, Gruber BD, von Wirén N 2014: It’s time to make changes: modulation of root system architecture by nutrient signals. J. Exp. Bot., 65, 769–778. doi:10.1093/jxb/ert421
- Gifford ML, Banta JA, Katari MS, Hulsmans J, Chen L, Ristova D, Tranchina D, Purugganan MD, Coruzzi GM, Birnbaum KD 2013: Plasticity regulators modulate specific root traits in discrete nitrogen environments. PLoS Genet., 9, e1003760. doi:10.1371/journal.pgen.1003760
- Gowri G, Campbell WH 1989: cDNA clones for corn leaf NADH-nitrate reductase and chloroplast NAD(P)+: glyceraldehyde-3-phosphatedehydrogenase – characterization of the clones and analysis of the expression of the genes in leaves as influenced by nitrate in the light and dark. Plant Physiol., 90, 792–798. doi:10.1104/pp.90.3.792
- Griffiths CA, Paul MJ, Foyer CH 2016: Metabolite transport and associated sugar signalling systems underpinning source/sink interactions. Biochim. Biophys. Acta Bioenerg., 1857, 1715–1725. doi:10.1016/j.bbabio.2016.07.007
- GRiSP 2013: Rice Almanac. International Rice Research Institute, Los Baños.
- Gu R, Duan F, An X, Zhang F, von Wirén N, Yuan L 2013: Characterization of AMT-mediated high-affinity ammonium uptake in roots of maize (Zea mays L.). Plant Cell Physiol., 54, 1515–1524. doi:10.1093/pcp/pct099
- Guan P, Ripoll J-J, Wang R, Vuong L, Bailey-Steinitz LJ, Ye D, Crawford NM 2017: Interacting TCP and NLP transcription factors control plant responses to nitrate availability. Proc. Natl. Acad. Sci. USA, 114, 2419–2424. doi:10.1073/pnas.1615676114
- Gungula DT, Kling JG, Togun AO 2003: CERES-Maize predictions of maize phenology under nitrogen-stressed conditions in Nigeria. Agron. J., 95, 892–899. doi:10.2134/agronj2003.0892
- Hachiya T, Sakakibara H 2016: Interactions between nitrate and ammonium in their uptake, allocation, assimilation, and signaling in plants. J. Exp. Bot. doi:10.1093/jxb/erw449
- Hageman RH, Flesher D 1960: Nitrate reductase activity in corn seedlings as affected by light and nitrate content of nutrient media. Plant Physiol., 35, 700–708. doi:10.1104/pp.35.5.700
- Hall AJ, Savin R, Slafer GA 2014: Is time to flowering in wheat and barley influenced by nitrogen?: a critical appraisal of recent published reports. Eur. J. Agron., 54, 40–46. doi:10.1016/j.eja.2013.11.006
- He C-J, Morgan PW, Drew MC 1992: Enhanced sensitivity to ethylene in nitrogen- or phosphate-starved roots of Zea mays L. during aerenchyma formation. Plant Physiol., 98, 137–142. doi:10.1104/pp.98.1.137
- Hewitt EJ, Afridi MMRK 1959: Adaptive synthesis of nitrate reductase in higher plants. Nature, 183, 57–58. doi:10.1038/183057a0
- Hinsinger P, Gobran GR, Gregory PJ, Wenzel WW 2005: Rhizosphere geometry and heterogeneity arising from root mediated physical and chemical processes. New Phytol., 168, 293–303. doi:10.1111/j.1469-8137.2005.01512.x
- Ho C-H, Lin S-H, Hu H-C, Tsay Y-F 2009: CHL1 functions as a nitrate sensor in plants. Cell, 138, 1184–1194. doi:10.1016/j.cell.2009.07.004
- Hodge A 2004: The plastic plant: root responses to heterogeneous supplies of nutrients. New Phytol., 162, 9–24. doi:10.1111/nph.2004.162.issue-1
- Hu B, Wang W, Ou S et al. 2015: Variation in NRT1.1B contributes to nitrate-use divergence between rice subspecies. Nat. Genet., 47, 834–838. doi:10.1038/ng.3337
- Kant S, Peng M, Rothstein SJ 2011: Genetic regulation by NLA and microRNA827 for maintaining nitrate-dependent phosphate homeostasis in Arabidopsis. PLoS Genet., 7, e1002021. doi:10.1371/journal.pgen.1002021
- Kiba T, Krapp A 2016: Plant nitrogen acquisition under low availability: regulation of uptake and root architecture. Plant Cell Physiol., 57, 707–714. doi:10.1093/pcp/pcw052
- Kirk GJD 2001: Plant-mediated processes to acquire nutrients: nitrogen uptake by rice plants. Plant Soil, 232, 129–134. doi:10.1023/A:1010341116376
- Kirk GJD, Kronzucker HJ 2005: The potential for nitrification and nitrate uptake in the rhizosphere of wetland plants: a modelling study. Ann. Bot., 96, 639–646. doi:10.1093/aob/mci216
- Konishi M, Yanagisawa S 2013a: Arabidopsis NIN-like transcription factors have a central role in nitrate signalling. Nat. Commun., 4, 1617. doi:10.1038/ncomms2621
- Konishi M, Yanagisawa S 2013b: An NLP-binding site in the 3ʹ flanking region of the nitrate reductase gene confers nitrate-inducible expression in Arabidopsis thaliana (L.) Heynh. Soil Sci. Plant Nutr., 59, 612–620. doi:10.1080/00380768.2013.809602
- Konishi M, Yanagisawa S 2014: Emergence of a new step towards understanding the molecular mechanisms underlying nitrate-regulated gene expression. J. Exp. Bot., 65, 5589–5600. doi:10.1093/jxb/eru267
- Konishi N, Ishiyama K, Matsuoka K, Maru I, Hayakawa T, Yamaya T, Kojima S 2014: NADH-dependent glutamate synthase plays a crucial role in assimilating ammonium in the Arabidopsis root. Physiol. Plantarum, 152, 138–151. doi:10.1111/ppl.12177
- Krapp A 2015: Plant nitrogen assimilation and its regulation: a complex puzzle with missing pieces. Curr. Opin. Plant Biol., 25, 115–122. doi:10.1016/j.pbi.2015.05.010
- Krouk G 2016: Hormones and nitrate: a two-way connection. Plant Mol. Biol., 91, 599–606. doi:10.1007/s11103-016-0463-x
- Krouk G, Lacombe B, Bielach A et al. 2010: Nitrate-regulated auxin transport by NRT1.1 defines a mechanism for nutrient sensing in plants. Dev. Cell, 18, 927–937. doi:10.1016/j.devcel.2010.05.008
- Kushiro T, Okamoto M, Nakabayashi K, Yamagishi K, Kitamura S, Asami T, Hirai N, Koshiba T, Kamiya Y, Nambara E 2004: The Arabidopsis cytochrome P450 CYP707A encodes ABA 8ʹ-hydroxylases: key enzymes in ABA catabolism. EMBO J., 23, 1647–1656. doi:10.1038/sj.emboj.7600121
- Lahners K, Kramer V, Back E, Privalle L, Rothstein S 1988: Molecular cloning of complementary DNA encoding maize nitrite reductase: molecular analysis and nitrate induction. Plant Physiol., 88, 741–746. doi:10.1104/pp.88.3.741
- Lee RB, Rudge KA 1986: Effects of nitrogen deficiency on the absorption of nitrate and ammonium by barley plants. Ann. Bot., 57, 471–486. doi:10.1093/oxfordjournals.aob.a087129
- Li B, Xin W, Sun S, Shen Q, Xu G 2006: Physiological and molecular responses of nitrogen-starved rice plants to re-supply of different nitrogen sources. Plant Soil, 287, 145–159. doi:10.1007/s11104-006-9051-1
- Li Y, Kronzucker HJ, Shi W 2016: Microprofiling of nitrogen patches in paddy soil: analysis of spatiotemporal nutrient heterogeneity at the microscale. Sci. Rep., 6, 27064. doi:10.1038/srep27064
- Li YL, Fan XR, Shen QR 2008: The relationship between rhizosphere nitrification and nitrogen-use efficiency in rice plants. Plant, Cell Environ., 31, 73–85.
- Lima JE, Kojima S, Takahashi H, von Wirén N 2010: Ammonium triggers lateral root branching in Arabidopsis in an AMMONIUM TRANSPORTER1;3-dependent manner. Plant Cell, 22, 3621–3633. doi:10.1105/tpc.110.076216
- Liu H, Yang H, Wu C, Feng J, Liu X, Qin H, Wang D 2009: Overexpressing HRS1 confers hypersensitivity to low phosphate-elicited inhibition of primary root growth in Arabidopsis thaliana. J. Integr. Plant Biol., 51, 382–392. doi:10.1111/j.1744-7909.2009.00819.x
- Liu K-H, Niu Y, Konishi M et al. 2017a: Discovery of nitrate-CPK-NLP signalling in central nutrient-growth networks. Nature, 545, 311–316. doi:10.1038/nature22077
- Liu W, Sun Q, Wang K, Du Q, Li W-X 2017b: Nitrogen Limitation Adaptation (NLA) is involved in source-to-sink remobilization of nitrate by mediating the degradation of NRT1.7 in Arabidopsis. New Phytol., 214, 734–744. doi:10.1111/nph.14396
- Liu X, Feng H, Huang D, Song M, Fan X 2015: Two short sequences in OsNAR2.1 promoter are necessary for fully activating the nitrate induced gene expression in rice roots. Sci. Rep., 5, 11950. doi:10.1038/srep11950
- Liu X, Huang D, Tao J, Miller AJ, Fan X, Xu G 2014: Identification and functional assay of the interaction motifs in the partner protein OsNAR2.1 of the two-component system for high-affinity nitrate transport. New Phytol., 204, 74–80. doi:10.1111/nph.12986
- Maeda S, Konishi M, Yanagisawa S, Omata T 2014: Nitrite transport activity of a novel HPP family protein conserved in cyanobacteria and chloroplasts. Plant Cell Physiol., 55, 1311–1324. doi:10.1093/pcp/pcu075
- Makino A 2011: Photosynthesis, grain yield, and nitrogen utilization in rice and wheat. Plant Physiol., 155, 125–129. doi:10.1104/pp.110.165076
- Makino A, Osmond B 1991: Effects of nitrogen nutrition on nitrogen partitioning between chloroplasts and mitochondria in pea and wheat. Plant Physiol., 96, 355–362. doi:10.1104/pp.96.2.355
- Marchive C, Roudier F, Castaings L, Bréhaut V, Blondet E, Colot V, Meyer C, Krapp A 2013: Nuclear retention of the transcription factor NLP7 orchestrates the early response to nitrate in plants. Nat. Commun., 4, 1713. doi:10.1038/ncomms2650
- Marín IC, Loef I, Bartetzko L, Searle I, Coupland G, Stitt M, Osuna D 2011: Nitrate regulates floral induction in Arabidopsis, acting independently of light, gibberellin and autonomous pathways. Planta, 233, 539–552. doi:10.1007/s00425-010-1316-5
- Martin T, Oswald O, Graham IA 2002: Arabidopsis seedling growth, storage lipid mobilization, and photosynthetic gene expression are regulated by carbon: nitrogen availability. Plant Physiol., 128, 472–481. doi:10.1104/pp.010475
- Matakiadis T, Alboresi A, Jikumaru Y, Tatematsu K, Pichon O, Renou J-P, Kamiya Y, Nambara E, Truong H-N 2009: The Arabidopsis abscisic acid catabolic gene CYP707A2 plays a key role in nitrate control of seed dormancy. Plant Physiol., 149, 949–960. doi:10.1104/pp.108.126938
- Medici A, Krouk G 2014: The primary nitrate response: a multifaceted signalling pathway. J. Exp. Bot., 65, 5567–5576. doi:10.1093/jxb/eru245
- Medici A, Marshall-Colon A, Ronzier E, Szponarski W, Wang R, Gojon A, Crawford NM, Ruffel S, Coruzzi GM, Krouk G 2015: AtNIGT1/HRS1 integrates nitrate and phosphate signals at the Arabidopsis root tip. Nat. Commun., 6, 6274. doi:10.1038/ncomms7274
- Melo-Oliveira R, Oliveira IC, Coruzzi GM 1996: Arabidopsis mutant analysis and gene regulation define a nonredundant role for glutamate dehydrogenase in nitrogen assimilation. Proc. Natl. Acad. Sci. USA, 93, 4718–4723. doi:10.1073/pnas.93.10.4718
- Menz J, Li Z, Schulze WX, Ludewig U 2016: Early nitrogen-deprivation responses in Arabidopsis roots reveal distinct differences on transcriptome and (phospho-) proteome levels between nitrate and ammonium nutrition. Plant J., 88, 717–734. doi:10.1111/tpj.2016.88.issue-5
- Miller AJ, Cramer MD 2005: Root nitrogen acquisition and assimilation. Plant Soil, 274, 1–36. doi:10.1007/s11104-004-0965-1
- Miller AJ, Fan X, Orsel M, Smith SJ, Wells DM 2007: Nitrate transport and signalling. J. Exp. Bot., 58, 2297–2306. doi:10.1093/jxb/erm066
- Miura K, Lee J, Gong Q, Ma S, Jin JB, Yoo CY, Miura T, Sato A, Bohnert HJ, Hasegawa PM 2011: SIZ1 regulation of phosphate starvation-induced root architecture remodeling involves the control of auxin accumulation. Plant Physiol., 155, 1000–1012. doi:10.1104/pp.110.165191
- Miura K, Okamoto H, Okuma E, Shiba H, Kamada H, Hasegawa PM, Murata Y 2013: SIZ1 deficiency causes reduced stomatal aperture and enhanced drought tolerance via controlling salicylic acid-induced accumulation of reactive oxygen species in Arabidopsis. Plant J., 73, 91–104. doi:10.1111/tpj.12014
- Miura K, Rus A, Sharkhuu A et al. 2005: The Arabidopsis SUMO E3 ligase SIZ1 controls phosphate deficiency responses. Proc. Natl. Acad. Sci. USA, 102, 7760–7765. doi:10.1073/pnas.0500778102
- Mounier E, Pervent M, Ljung K, Gojon A, Nacry P 2014: Auxin-mediated nitrate signalling by NRT1.1 participates in the adaptive response of Arabidopsis root architecture to the spatial heterogeneity of nitrate availability. Plant, Cell Environ., 37, 162–174. doi:10.1111/pce.2014.37.issue-1
- Nacry P, Bouguyon E, Gojon A 2013: Nitrogen acquisition by roots: physiological and developmental mechanisms ensuring plant adaptation to a fluctuating resource. Plant Soil, 370, 1–29. doi:10.1007/s11104-013-1645-9
- Nerson H, Edelstein M, Pinthus MJ 1990: Effects of N and P nutrition on spike development in spring wheat. Plant Soil, 124, 33–37. doi:10.1007/BF00010928
- Okamoto M, Kuwahara A, Seo M, Kushiro T, Asami T, Hirai N, Kamiya Y, Koshiba T, Nambara E 2006: CYP707A1 and CYP707A2, which encode abscisic acid 8ʹ-hydroxylases, are indispensable for proper control of seed dormancy and germination in Arabidopsis. Plant Physiol., 141, 97–107. doi:10.1104/pp.106.079475
- Owen AG, Jones DL 2001: Competition for amino acids between wheat roots and rhizosphere microorganisms and the role of amino acids in plant N acquisition. Soil Biol. Biochem., 33, 651–657. doi:10.1016/S0038-0717(00)00209-1
- Park BS, Seo JS, Chua N-H 2014: NITROGEN LIMITATION ADAPTATION recruits PHOSPHATE2 to target the phosphate transporter PT2 for degradation during the regulation of Arabidopsis phosphate homeostasis. Plant Cell, 26, 454–464. doi:10.1105/tpc.113.120311
- Park BS, Song JT, Seo HS 2011: Arabidopsis nitrate reductase activity is stimulated by the E3 SUMO ligase AtSIZ1. Nat. Commun., 2, 400. doi:10.1038/ncomms1408
- Patterson K, Cakmak T, Cooper A, Lager I, Rasmusson AG, Escobar MA 2010: Distinct signalling pathways and transcriptome response signatures differentiate ammonium- and nitrate-supplied plants. Plant, Cell Environ., 33, 1486–1501.
- Peng M, Hannam C, Gu H, Bi Y-M, Rothstein SJ 2007: A mutation in NLA, which encodes a RING-type ubiquitin ligase, disrupts the adaptability of Arabidopsis to nitrogen limitation. Plant J., 50, 320–337. doi:10.1111/j.1365-313X.2007.03050.x
- Postma JA, Lynch JP 2011: Root cortical aerenchyma enhances the growth of maize on soils with suboptimal availability of nitrogen, phosphorus, and potassium. Plant Physiol., 156, 1190–1201. doi:10.1104/pp.111.175489
- Pouteau S, Cherel I, Vaucheret H, Caboche M 1989: Nitrate reductase mRNA regulation in Nicotiana plumbaginifolia nitrate reductase-deficient mutants. Plant Cell, 1, 1111–1120. doi:10.1105/tpc.1.11.1111
- Qin C, Qian W, Wang W, Wu Y, Yu C, Jiang X, Wang D, Wu P 2008: GDP-mannose pyrophorphorylase is a genetic determinant of ammonium sensitivity in Arabidopsis thaliana. Proc. Natl. Acad. Sci. USA, 105, 18308–18313. doi:10.1073/pnas.0806168105
- Remans T, Nacry P, Pervent M, Filleur S, Diatloff E, Mounier E, Tillard P, Forde BG, Gojon A 2006: The Arabidopsis NRT1.1 transporter participates in the signaling pathway triggering root colonization of nitrate-rich patches. Proc. Natl. Acad. Sci. USA, 103, 19206–19211. doi:10.1073/pnas.0605275103
- Riveras E, Alvarez JM, Vidal EA, Oses C, Vega A, Gutiérrez RA 2015: The calcium ion is a second messenger in the nitrate signaling pathway of Arabidopsis. Plant Physiol., 169, 1397–1404. doi:10.1104/pp.15.00961
- Robinson D, Hodge A, Griffiths BS, Fitter AH 1999: Plant root proliferation in nitrogen-rich patches confers competitive advantage. Proc. Roy. Soc. Lond. B: Biol. Sci., 266, 431–435. doi:10.1098/rspb.1999.0656
- Rosas U, Cibrian-Jaramillo A, Banta JA et al. 2013: Integration of responses within and across Arabidopsis natural accessions uncovers loci controlling root systems architecture. Proc. Natl. Acad. Sci. USA, 110, 15133–15138. doi:10.1073/pnas.1305883110
- Rubinigg M, Stulen I, Theo J, Elzenga M, Colmer TD 2002: Spatial patterns of radial oxygen loss and nitrate net flux along adventitious roots of rice raised in aerated or stagnant solution. Funct. Plant Biol., 29, 1475–1481. doi:10.1071/FP02081
- Sakakibara H, Kobayashi K, Deji A, Sugiyama T 1997: Partial characterization of the signaling pathway for the nitrate-dependent expression of genes for nitrogen-assimilatory enzymes using detached maize leaves. Plant Cell Physiol., 38, 837–843. doi:10.1093/oxfordjournals.pcp.a029242
- Sasakawa H, Yamamoto Y 1978: Comparison of the uptake of nitrate and ammonium by rice seedlings. Plant Physiol., 62, 665–669. doi:10.1104/pp.62.4.665
- Sawaki N, Tsujimoto R, Shigyo M, Konishi M, Toki S, Fujiwara T, Yanagisawa S 2013: A nitrate-inducible GARP family gene encodes an auto-repressible transcriptional repressor in rice. Plant Cell Physiol., 54, 506–517. doi:10.1093/pcp/pct007
- Schauser L, Roussis A, Stiller J, Stougaard J 1999: A plant regulator controlling development of symbiotic root nodules. Nature, 402, 191–195. doi:10.1038/46058
- Schauser L, Wieloch W, Stougaard J 2005: Evolution of NIN-like proteins in Arabidopsis, rice, and Lotus japonicus. J. Mol. Evol., 60, 229–237. doi:10.1007/s00239-004-0144-2
- Scheible WR, Gonzalez-Fontes A, Lauerer M, Müller-Rober B, Caboche M, Stitt M 1997a: Nitrate acts as a signal to induce organic acid metabolism and repress starch metabolism in tobacco. Plant Cell, 9, 783–798. doi:10.1105/tpc.9.5.783
- Scheible W-R, González-Fontes A, Morcuende R, Lauerer M, Geiger M, Glaab J, Gojon A, Schulze E-D, Stitt M 1997b: Tobacco mutants with a decreased number of functional nia genes compensate by modifying the diurnal regulation of transcription, post-translational modification and turnover of nitrate reductase. Planta, 203, 304–319. doi:10.1007/s004250050196
- Scheible WR, Morcuende R, Czechowski T, Fritz C, Osuna D, Palacios-Rojas N, Schindelasch D, Thimm O, Udvardi MK, Stitt M 2004: Genome-wide reprogramming of primary and secondary metabolism, protein synthesis, cellular growth processes, and the regulatory infrastructure of Arabidopsis in response to nitrogen. Plant Physiol., 136, 2483–2499. doi:10.1104/pp.104.047019
- Siyiannis VF, Protonotarios VE, Zechmann B, Chorianopoulou SN, Müller M, Hawkesford MJ, Bouranis DL 2012: Comparative spatiotemporal analysis of root aerenchyma formation processes in maize due to sulphate, nitrate or phosphate deprivation. Protoplasma, 249, 671–686. doi:10.1007/s00709-011-0309-y
- Smith FW, Jackson WA 1987a: Nitrogen enhancement of phosphate transport in roots of Zea mays L. I. Effects of ammonium and nitrate pretreatment. Plant Physiol., 84, 1314–1318. doi:10.1104/pp.84.4.1314
- Smith FW, Jackson WA 1987b: Nitrogen enhancement of phosphate transport in roots of Zea mays L. II. Kinetic and inhibitor studies. Plaant Physiol., 84, 1319–1324. doi:10.1104/pp.84.4.1319
- Sonoda Y, Ikeda A, Saiki S, Yamaya T, Yamaguchi J 2003: Feedback regulation of the ammonium transporter gene family AMT1 by glutamine in rice. Plant Cell Physiol., 44, 1396–1402. doi:10.1093/pcp/pcg169
- Soyano T, Kouchi H, Hirota A, Hayashi M 2013: Nodule inception directly targets NF-Y subunit genes to regulate essential processes of root nodule development in Lotus japonicus. PLoS Genet., 9, e1003352. doi:10.1371/journal.pgen.1003352
- Steinbauer GP, Grigsby B 1957: Interaction of temperature, light, and moistening agent in the germination of weed seeds. Weeds, 5, 175–182. doi:10.2307/4040187
- Sumimoto H, Kamakura S, Ito T 2007: Structure and function of the PB1 domain, a protein interaction module conserved in animals, fungi, amoebas, and plants. Sci. STKE, 2007, re6. doi:10.1126/stke.4012007re6
- Sun H, Tao J, Liu S, Huang S, Chen S, Xie X, Yoneyama K, Zhang Y, Xu G 2014: Strigolactones are involved in phosphate- and nitrate-deficiency-induced root development and auxin transport in rice. J. Exp. Bot., 65, 6735–6746. doi:10.1093/jxb/eru029
- Suzuki W, Konishi M, Yanagisawa S 2013: The evolutionary events necessary for the emergence of symbiotic nitrogen fixation in legumes may involve a loss of nitrate responsiveness of the NIN transcription factor. Plant Signal. Behav., 8, e25975. doi:10.4161/psb.25975
- Takei K, Takahashi T, Sugiyama T, Yamaya T, Sakakibara H 2002: Multiple routes communicating nitrogen availability from roots to shoots: a signal transduction pathway mediated by cytokinin. J. Exp. Bot., 53, 971–977. doi:10.1093/jexbot/53.370.971
- Takei K, Ueda N, Aoki K, Kuromori T, Hirayama T, Shinozaki K, Yamaya T, Sakakibara H 2004: AtIPT3 is a key determinant of nitrate-dependent cytokinin biosynthesis in Arabidopsis. Plant Cell Physiol., 45, 1053–1062. doi:10.1093/pcp/pch119
- Tang P-S, Wu H-Y 1957: Adaptive formation of nitrate reductase in rice seedlings. Nature, 179, 1355–1356. doi:10.1038/1791355a0
- Taylor AR, Bloom AJ 1998: Ammonium, nitrate, and proton fluxes along the maize root. Plant, Cell Environ., 21, 1255–1263. doi:10.1046/j.1365-3040.1998.00357.x
- Tian Q, Chen F, Liu J, Zhang F, Mi G 2008: Inhibition of maize root growth by high nitrate supply is correlated with reduced IAA levels in roots. J. Plant Physiol., 165, 942–951. doi:10.1016/j.jplph.2007.02.011
- Tsay Y-F, Ho C-H, Chen H-Y, Lin S-H 2011: Integration of nitrogen and potassium signaling. Annu. Rev. Plant Biol., 62, 207–226. doi:10.1146/annurev-arplant-042110-103837
- Vidal EA, Álvarez JM, Gutiérrez RA 2014a: Nitrate regulation of AFB3 and NAC4 gene expression in Arabidopsis roots depends on NRT1.1 nitrate transport function. Plant Signal. Behav., 9, e28501. doi:10.4161/psb.28501
- Vidal EA, Araus V, Lu C, Parry G, Green PJ, Coruzzi GM, Gutiérrez RA 2010: Nitrate-responsive miR393/AFB3 regulatory module controls root system architecture in Arabidopsis thaliana. Proc. Natl. Acad. Sci. USA, 107, 4477–4482. doi:10.1073/pnas.0909571107
- Vidal EA, Moyano TC, Canales J, Gutiérrez RA 2014b: Nitrogen control of developmental phase transitions in Arabidopsis thaliana. J. Exp. Bot., 65, 5611–5618. doi:10.1093/jxb/eru326
- Walch-Liu P, Forde BG 2008: Nitrate signalling mediated by the NRT1.1 nitrate transporter antagonises L-glutamate-induced changes in root architecture. Plant J., 54, 820–828. doi:10.1111/j.1365-313X.2008.03443.x
- Walch-Liu P, Neumann G, Bangerth F, Engels C 2000: Rapid effects of nitrogen form on leaf morphogenesis in tobacco. J. Exp. Bot., 51, 227–237. doi:10.1093/jexbot/51.343.227
- Wang H, Sun R, Cao Y et al. 2015: OsSIZ1, a SUMO E3 ligase gene, is involved in the regulation of the responses to phosphate and nitrogen in rice. Plant Cell Physiol., 56, 2381–2395. doi:10.1093/pcp/pcv162
- Wang R, Guegler K, LaBrie ST, Crawford NM 2000: Genomic analysis of a nutrient response in Arabidopsis reveals diverse expression patterns and novel metabolic and potential regulatory genes induced by nitrate. Plant Cell, 12, 1491–1509. doi:10.1105/tpc.12.8.1491
- Wang R, Okamoto M, Xing X, Crawford NM 2003: Microarray analysis of the nitrate response in Arabidopsis roots and shoots reveals over 1,000 rapidly responding genes and new linkages to glucose, trehalose-6-phosphate, iron, and sulfate metabolism. Plant Physiol., 132, 556–567. doi:10.1104/pp.103.021253
- Wang R, Tischner R, Gutiérrez RA, Hoffman M, Xing X, Chen M, Coruzzi G, Crawford NM 2004: Genomic analysis of the nitrate response using a nitrate reductase-null mutant of Arabidopsis. Plant Physiol., 136, 2512–2522. doi:10.1104/pp.104.044610
- Wang Y-Y, Hsu P-K, Tsay Y-F 2012: Uptake, allocation and signaling of nitrate. Trends Plant Sci., 17, 458–467. doi:10.1016/j.tplants.2012.04.006
- Watanabe K, Nishiuchi S, Kulichikhin K, Nakazono M 2013: Does suberin accumulation in plant roots contribute to waterlogging tolerance? Front. Plant Sci., 4, 178. doi:10.3389/fpls.2013.00178
- Williams RL, Angus JF 1994: Deep floodwater protects high-nitrogen rice crops from low-temperature damage. Aust. J. Exp. Agr., 34, 927–932. doi:10.1071/EA9940927
- Xiao J, Li C, Xu S, Xing L, Xu Y, Chong K 2015: JACALIN-LECTIN LIKE1 regulates the nuclear accumulation of GLYCINE-RICH RNA-BINDING PROTEIN7, influencing the RNA processing of FLOWERING LOCUS C antisense transcripts and flowering time in Arabidopsis. Plant Physiol., 169, 2102–2117.
- Xie W, Wang G, Yuan M et al. 2015: Breeding signatures of rice improvement revealed by a genomic variation map from a large germplasm collection. Proc. Natl. Acad. Sci. USA, 112, E5411–E5419. doi:10.1073/pnas.1515919112
- Xu G, Fan X, Miller AJ 2012: Plant nitrogen assimilation and use efficiency. Annu. Rev. Plant Biol., 63, 153–182. doi:10.1146/annurev-arplant-042811-105532
- Yan D, Easwaran V, Chau V et al. 2016: NIN-like protein 8 is a master regulator of nitrate-promoted seed germination in Arabidopsis. Nat. Commun., 7, 13179. doi:10.1038/ncomms13179
- Yan M, Fan X, Feng H, Miller AJ, Shen Q, Xu G 2011: Rice OsNAR2.1 interacts with OsNRT2.1, OsNRT2.2 and OsNRT2.3a nitrate transporters to provide uptake over high and low concentration ranges. Plant, Cell Environ., 34, 1360–1372. doi:10.1111/j.1365-3040.2011.02335.x
- Yan Y, Wang H, Hamera S, Chen X, Fang R 2014: MiR444a has multiple functions in the rice nitrate-signaling pathway. Plant J., 78, 44–55. doi:10.1111/tpj.2014.78.issue-1
- Yanagisawa S 2014: Transcription factors involved in controlling the expression of nitrate reductase genes in higher plants. Plant Sci., 229, 167–171. doi:10.1016/j.plantsci.2014.09.006
- Yoneyama K, Xie X, Kim HI, Kisugi T, Nomura T, Sekimoto H, Yokota T, Yoneyama K 2012: How do nitrogen and phosphorus deficiencies affect strigolactone production and exudation? Planta, 235, 1197–1207. doi:10.1007/s00425-011-1568-8
- Yuan S, Zhang Z-W, Zheng C et al. 2016: Arabidopsis cryptochrome 1 functions in nitrogen regulation of flowering. Proc. Natl. Acad. Sci. USA, 113, 7661–7666. doi:10.1073/pnas.1602004113
- Zhang H, Forde BG 1998: An Arabidopsis MADS box gene that controls nutrient-induced changes in root architecture. Science, 279, 407–409. doi:10.1126/science.279.5349.407
- Zhang H, Rong H, Pilbeam D 2007: Signalling mechanisms underlying the morphological responses of the root system to nitrogen in Arabidopsis thaliana. J. Exp. Bot., 58, 2329–2338. doi:10.1093/jxb/erm114
- Zhang J, Xu L, Wang F, Deng M, Yi K 2012: Modulating the root elongation by phosphate/nitrogen starvation in an OsGLU3 dependant way in rice. Plant Signal. Behav., 7, 1144–1145. doi:10.4161/psb.21334
- Zhuo D, Okamoto M, Vidmar JJ, Glass ADM 1999: Regulation of a putative high-affinity nitrate transporter (Nrt2;1At) in roots of Arabidopsis thaliana. Plant J., 17, 563–568. doi:10.1046/j.1365-313X.1999.00396.x
- Zou J-J, Wei F-J, Wang C, Wu J-J, Ratnasekera D, Liu W-X, Wu W-H 2010: Arabidopsis calcium-dependent protein kinase CPK10 functions in abscisic acid- and Ca2+-mediated stomatal regulation in response to drought stress. Plant Physiol., 154, 1232–1243. doi:10.1104/pp.110.157545