ABSTRACT
Plants are known to develop cross-tolerance in which plants tolerate to one stress following prior exposure to another stress. In this study, we investigated this phenomenon in rice (Oryza sativa L.) to elucidate the underlying physiological mechanisms. Twenty-one (21) day-old seedlings were pre-exposed to various concentrations of NaCl, NaHCO3, and H2O2 for 7 days and then subjected to saline-alkaline stress (SAS) for 14 days. The present findings report that pre-exposure to 10 mM NaCl and 10 µM H2O2 develops cross-tolerance to SAS by triggering systemic physiological processes involved in Fe uptake and ROS homeostasis. While the mechanisms of this tolerance vary among pre-treatments, a consensus suggests the relevance of the following processes: (1) the ability to maintain a lower growth medium pH, (2) ability to suppress root K+ losses, (3) maintenance of a stable acquisition of Fe and P and (4) regulated generation of reactive oxygen species (ROS) via enhanced antioxidant enzyme activities. Taken together, these results are consistent with the hypothesis that a crosstalk exists between salinity stress, oxidative stress, and SAS, triggering adaptive physiological responses that develop tolerance to SAS in rice, a phenomenon that offers a prospect for developing plants tolerant to multiple stressors.
1. Introduction
Saline-alkaline stress (SAS) is a serious environmental threat that limits agricultural productivity worldwide. It is characterized by an inordinately high soil pH (Zhang et al. Citation2017), hyperosmotic stress, ionic toxicity (Na+), and oxidative stress. High pH limits the bioavailability of some essential micronutrients, particularly iron, and most efforts aimed at increasing tolerance to high pH in rice entailed increasing iron uptake (Masuda et al. Citation2008, Citation2009; Suzuki et al. Citation2008). Rice (Oryza sativa L.) is a cereal crop that is extremely sensitive to salt stress (Munns and Tester Citation2008) and exhibits poor iron uptake capacities under high pH. Therefore, SAS poses a much greater risk to rice growth than sole salinity stress, necessitating the identification of agronomic approaches that can increase the resistance of plants under mixed SAS conditions.
Plants have remarkable abilities to acclimate to lethal stress events when subjected to prior mild stress (Pandolfi, Mancuso, and Shabala Citation2012). This is referred to as acclimation, which describes the physiological ability of plants to adapt to stressful events. Acclimation has been demonstrated as an effective and quicker way to improve plant tolerance to various abiotic stresses such as salinity stress (Kamanga et al. Citation2020; Sriskantharajah et al. Citation2020; Umezawa et al. Citation2000). In a primed state, plants are typified by faster and stronger responses triggered by systemic defense systems that confer enhanced resistance.
Under SAS conditions, Na+ toxicity, osmotic stress, oxidative stress, and high pH are key stress factors responsible for growth inhibition and plant death (Yang et al. Citation2009; Zhang et al. Citation2019); hence, the choice of acclimating agents should aim at those that may enhance defense responses against these stress factors. Studies have shown that plant leaves accumulate multiple folds higher Na+ under SAS conditions compared to neutral salinity stress (Cao et al. Citation2020; Jia et al. Citation2017). While it remains unclear, it is speculated that high pH either results in uncontrollable uptake of Na+ by roots or reduces the SOS1-mediated Na+ efflux in plant roots because of a deficiency in the transmembrane proton gradient required for SOS1 activity (Haruta and Sussman Citation2012; Yang et al. Citation2019). Therefore, to address the latter, the ability to maintain a lower growth medium pH is a prerequisite for the regulation of leaf Na+ toxicity through root Na+ extrusion. Moreover, rhizospheric acidification is also necessary for iron uptake in plants utilizing strategy I, solubilizing ferric iron (Fe3+) to utilizable ferrous iron (Fe2+) for uptake by plant roots.
The key components in a typical stress-response relationship involve stress stimulus, perception of stress by signals, expression of stress-induced genes, and resultant changes at morphological, biochemical, and physiological levels (Pastori and Foyer Citation2002). The signaling and response pathways have been reported to overlap during exposure to different abiotic stresses, including reactive oxygen species (ROS), hormones, protein kinase cascades, and calcium gradients as common elements (Atkinson and Urwin Citation2012). ROS are unstable molecules inevitably produced by plants, both as a result of normal aerobic metabolism and in response to abiotic stresses. Meanwhile, more evidence suggests the beneficial roles of ROS, such as stress signaling (Apel and Hirt Citation2004; Mittler Citation2017). Therefore, their fate relies on tight regulation of the equilibrium between their generation and detoxification. However, this delicate balance is upset under abiotic stress, resulting in oxidative stress, one of the prime suspected factors of lethality under SAS conditions (Guo et al. Citation2014; Zhang et al. Citation2017). Therefore, plants acclimated to oxidative stress may show better resistance to SAS by activating systemic defense systems to counteract the stress. This illustrates the concept of induced cross-tolerance in which priming of plants to one stress type enhances tolerance to another stress type (Foyer et al. Citation2016; Perez and Brown Citation2014), offering a prospect for breeding crop plants resistant to multiple forms of stress.
While studies of simultaneous stress exposure and subsequent exposure to similar stress are widespread, little is known regarding one-at-a-time exposure to different stresses. It is speculated that the crosstalk between multiple stresses partly involves the accumulation of ROS (Pastori and Foyer Citation2002; Perez and Brown Citation2014). This recognition has led agronomists to pursue chemical means to activate ROS-induced systemic resistance. This includes the direct application of ROS, particularly H2O2 (Fedina, Nedeva, and Çiçek Citation2009; Wahid et al. Citation2007), and the application of salts such as NaCl (Kamanga et al. Citation2020; Sriskantharajah et al. Citation2020) and NaHCO3 (Liu and Saneoka Citation2019), which are hypothesized to activate antioxidant defense systems. Nevertheless, little is known about the physiological mechanisms governing cross-tolerance between oxidative stress, salinity stress, and SAS. We hypothesized that acclimation to either or a combination of the four key saline-alkalinity stress factors, namely, Na+ toxicity, osmotic stress, oxidative stress, and high pH, may trigger inter-stress crosstalk that may upsurge resistance of rice seedlings to alkaline stress.
2. Materials and methods
2.1. Plant materials and growth conditions
Seeds of rice (Oryza sativa L.) cultivar Hinohikari were incubated, surface-sterilized, and germinated according to Sriskantharajah et al. (Citation2020). After one week, a slightly modified Kimura B nutrient solution was added to the hydroponic culture following Chuamnakthong, Nampei, and Ueda (Citation2019). The nutrient solution was provided by immersing the plant roots directly in the hydroponic solution. The experiment was conducted in a greenhouse at Hiroshima University’s Faculty of Biological Sciences Experimental Field. The hydroponic culture temperature was maintained at 28°C, and the average day air temperature was 30°C. After 18 days, seedlings were transplanted from a nylon mesh wire to 20 L buckets.
2.2. Treatment conditions
Twenty-six (26) day-old seedlings were subjected to three concentrations of each of the following three sets (N = 20) of pre-treatments for 7 days: (1) NaCl (S) (2.5, 5.0, and 10 mM), (2) H2O2 (OX) (1.0, 5.0, and 10 µM), and (3) NaHCO3 (SA) (0.5, 1.0, and 2.5 mM). A fourth (4) set of plants was non-pre-treated and maintained as controls (N = 40). Out of these, after 7 days acclimation period, half were subjected to saline-alkalinity (NPT) while the remaining were maintained as control plants (no pre-treatment and no saline-alkaline stress). Except for set 3, all sets were maintained at a pH of 5.0–5.5 using 2.0 N HCl and 2.0 N KOH. After 7 days, pre-treated (PT) and non-pre-treated (NPT) plants were subjected to main SAS treatment (50 mM Na, pH 8.25) using a mixture of 0.75 mM Na2CO3, 25 mM NaHCO3, and 23.5 mM NaCl. Growth medium pH was monitored every 24 hours but was left unadjusted except for control conditions adjusted to 5.0–5.5 daily. To evaluate whether pre-treatments facilitate a quicker recovery after the disappearance of SAS conditions, all PT plants were subjected to 50 mM Na, pH 8.25 for 14 days, at the conclusion of that all stressed plants were recovered when exposed to 0 mM Na (1x Kimura B), pH 5.0–5.5 for 10 days. Shoot and root dry weight, chlorophyll concentration, and membrane integrity were measured.
2.3. Determination of plant fresh and dry weight
Seedlings were harvested in replicates of 4–5 after 7 days of pre-treatment period, after 14 days of main stress, and after 10 days of the recovery period. Plant height and root length were measured using a plant-measuring ruler. Fresh weight (FW) was measured immediately after dissecting plants into leaf blades, leaf sheaths, and roots. Dry weights (DW) were obtained after oven-drying the samples for at least 72 hours at 70°C.
2.4. Chlorophyll concentration (SPAD)
Leaf-blade chlorophyll concentration was determined using a chlorophyll meter (SPAD – 502, Minolta Camera Co. Ltd., Japan) on three fresh fully expanded leaf blades of the same positions in all plants according to Kamanga et al. (Citation2020).
2.5. Determination of macro and micronutrient elements
Elemental analysis was performed on dried leaf blades and root samples after 14 days of stress imposition using inductively coupled plasma optical emission spectrometry (ICP-OES). For this purpose, 100 mg of dried leaf blades and roots were crushed using a freeze crusher (µT – 48, Taitec, Saitama, Japan). Acid digestion was conducted using 2 mL of H2SO4 followed by repetitive cycles of 1 mL of H2O2 addition and heating in a heat block at 150–200°C until the sample turned colorless. An extra hour of heating was performed to remove the remaining H2O2 after which the sample was filled to 25 mL using Milli-Q water. ICP analysis was performed using iCAP – 6300 (Thermo-Scientific, USA) at wavelengths 766.4, 818.3, 177.4, 259.9, 249.7, and 213.8, for K, Na, P, Fe, B, and Zn, respectively. Quantification was performed using various elemental standard solutions prepared in 1% HNO3.
2.6. K+ leakage and Na+ efflux experiment
In order to determine plant roots’ ability to retain K+, leakage of K+ was assessed by a method previously described in Pandolfi et al. (Citation2016) with slight modifications. Plants were pre-treated with various pre-treatments for 7 days, at the conclusion of which, four uniform seedlings of pre-treated and non-pre-treated plants were transferred into 50 mL falcon tubes containing 30 mL of 50 mM Na+, pH 8.5 (48 mM NaHCO3 + 1 mM Na2CO3) for 24 h. Four more seedlings were transferred into 30 mL deionized water as control plants. After 24 hours, solution was sampled for K+ analysis.
Efflux of Na+ from roots was assessed by a previously outlined method (Pandolfi et al. Citation2016; Shabala et al. Citation2010) with some modifications. Pre-treated and non-pre-treated plants were subjected to 30 mL of 50 mM Na+, pH 8.5 (48 mM NaHCO3 + 1 mM Na2CO3) for 24 hours, after which plants were transferred into bathing medium containing 0.1 mM CaCl2, 0.5 mM KCl and 50 mM Na+ of pH 8.5 (48 mM NaHCO3 + 1 mM Na2CO3). After 1 hour, the solution was poured off and roots were rinsed with 10 mM CaCl2 three times. Then, the roots were transferred into 10 mL bathing medium containing 0.1 mM CaCl2 and 0.5 mM KCl for 4 hours. The solution was then sampled and Na+ concentration was measured. Both K+ and Na+ analysis were done using flame photometry (ANA – 135, Tokyo Photoelectric, Tokyo, Japan).
2.7. Na+ uptake in excised leaves
In order to exclude contribution of Na+ exclusion from uptake by roots, rice seedlings were pre-treated with various pre-treatments for 7 days, thereafter uniform leaf blades were excised under running water, and immersed into 30 mM Na+, pH 8.0 at about the same depth (30 mm) for 3 days following a method described in Shabala et al. (Citation2010). Thereafter, immersed cut ends of leaf blades were thoroughly washed using deionized water, and dried at 65°C for 72 hours. Then, 50 mg of leaf blades was agitated on a shaker in 1.0 N HCl for 24 hours and measured for Na+ concentration using flame photometry according to Kamanga et al. (Citation2020).
2.8. Determination of membrane integrity
Membrane integrity was measured using electrolyte leakage method and lipid peroxidation at the end of 14 days of main stress and 7 days of recovery. Freshly harvested leaf blade disks and roots (200 mg) were cut into 2 cm pieces and fully immersed into 25 mL deionized water in 50 mL falcon tubes and kept at room temperature for 24 hours. Electrical conductivity (EC) was then obtained (EC1) using an EC meter (CM-31P, DKK Toa Co., Tokyo, Japan). Immediately, the samples were killed by incubating at 100°C for 15 minutes, cooled and EC2 was obtained. Electrolyte leakage rate was calculated according to Farooq and Azam (Citation2006).
Lipid peroxidation was determined by obtaining malondialdehyde (MDA) concentration using the improved thiorbarbituric acid (TBA) reaction previously described in Hodges et al. (Citation1999). For this purpose, 100 mg of fresh leaf and root samples were ground in presence of liquid nitrogen using mortar and pestle and extracted with 3 mL 80% ethanol after incubating under room temperature for 20 minutes and centrifuging at 3,000 x g for 10 minutes. Thereafter, two sets of 1 mL of the supernatant were transferred into two glass tubes respectively, one containing 1 mL TBA (-) solution (20% trichloro acetic acid and 0.01% buthyl hydroxy toluene) and another containing 1 mL TBA (+) solution (20% trichloro acetic acid, 0.01% buthyl hydroxy toluene and 0.65% thiobarbituric acid). MDA concentration was calculated from the absorbances of the samples measured spectrophotometrically (U-3310, Hitachi, Tokyo, Japan) using a 155 mM−1 cm−1 extinction coefficient according to Hodges et al. (Citation1999).
2.9. Measurement of root and leaf cell viability
Root and leaf cell viability were assessed using Evans Blue staining procedure as previously described in Zhang et al. (Citation2017). In brief, fresh root tips and leaf blades were cut into 2 cm long pieces, 100 mg of which were infiltrated with 1.5 mL of 0.25% aqueous solution of Evans Blue for 30 min under room temperature. Upon absorption of the stain, leaf and root samples were rinsed with deionized water until no further blue dye was eluted from the samples. To quantify the Evans Blue stain absorbed, the stain was solubilized by incubating in 4 mL of 1% (w/v) sodium dodecyl sulfate (SDS) prepared in 50% (v/v) methanol at 50°C for 30 minutes, and measure absorbance at 600 nm using SDS as a blank. Stain absorption was then quantified using Evans Blue standard solutions.
2.10. Determination of hydrogen peroxide concentration
H2O2 concentration was determined using ground frozen samples based on ferrous oxidation of xylenol orange (FOX) as previously described in Suharsono et al. (Citation2002). For this purpose, 100 mg of leaf blade and root samples were homogenized with 1 mL cold acetone. The homogenate was centrifuged at 8,000 x g for 15 minutes at 4°C, and 100 µL of the supernatant was added to 1 mL FOX reagent comprising 0.25 mM FeSO4, 0.25 mM (NH4)2SO4, 25 mM H2SO4, 125 µM xylenol orange, and 10 mM sorbitol. The reaction mixture was left at room temperature for 1 hour and H2O2 concentrations were determined spectrophotometrically at 560 nm and quantified using H2O2 standard solutions.
2.11. Antioxidant enzyme activity essays
To analyze antioxidant enzyme activities, 300 mg of frozen leaf blades and root samples were ground in the presence of liquid nitrogen using a mortar and pestle, extracted using 3 mL potassium phosphate (KPB) buffer (pH 7.0), and centrifuged at 10,000 × g for 15 minutes at 4°C. Catalase (CAT, EC 1.11.1.6) activity was measured as previously described (Mekawy, Abdelaziz, and Ueda Citation2018; Takagi and Yamada Citation2013). One unit of CAT activity was determined as mmol of H2O2 consumed min−1 mg−1 protein using an H2O2 extinction coefficient of 0.0436 mM−1 cm−1 at 240 nm. Ascorbate peroxidase (APX, EC 1.11.1.11) was assayed according to Mekawy, Abdelaziz, and Ueda (Citation2018). One unit of APX activity was determined as µmol of ascorbate oxidized min−1 mg−1 protein using an extinction coefficient of 2.8 mM−1 cm−1 at 290 nm. Glutathione reductase (GR, EC 1.8.1.7) activity was assayed according to the outlined method in Liu and Saneoka (Citation2019). One unit of GR activity was determined as µmol NADPH oxidized min−1 mg−1 protein using an extinction coefficient of 6.22 mM−1 cm−1 at 340 nm. Superoxide dismutase (SOD, EC.1.15.1.1) activity was assayed based on its ability to inhibit photochemical reduction of nitro blue tetrazolium (NBT), as outlined in Beauchamp and Fridovich (Citation1971). One unit of enzyme activity was defined as the quantity of enzyme that caused 50% inhibition of NBT photoreduction at 560 nm. Protein concentration in the enzyme extract was measured using the Bradford reagent (Bradford Citation1976) with bovine serum albumin as a standard.
2.12. Statistical analyses
The experiment was arranged in a completely randomized design (CRD). Each measurement was performed using four to five biological replicates. Inferential statistics were performed using analysis of variance (ANOVA) at a significance level of 0.05. Multiple comparison tests were performed using Tukey’s test. All data were analyzed using R Studio.
3. Results
3.1. Effectiveness of pre-treatments on plant growth
After 7 days of pre-treatments, no significant differences in growth were recorded among pre-treated and non-pre-treated plants (). At the end of 14 days of main stress, shoot and root dry weights were considerably decreased in NPT plants (). However, pre-treatment with 5 mM and 10 mM NaCl, and all concentrations of H2O2 (1, 5, and 10 µM) significantly increased shoot DW. Interestingly, acclimation to mild NaHCO3 (SA) pre-treatments was not effective (), decreasing growth except for 0.5 mM NaHCO3, which slightly increased both shoot and root growth. Overall, 10 mM NaCl, 10 µM H2O2, and 0.5 mM NaHCO3 were the most effective in their respective groups; hence, these pre-treatments were chosen for subsequent experiments and will be the main focus of this study.
Figure 1. Vegetative growth and physiological data showing dry weight after a 7-day acclimation period (A) and dry weight (B) and leaf chlorophyll concentration shown as SPAD units (C) after 14 days of exposure to main saline-alkaline stress (SAS) treatment (B). plants were pre-treated with various concentrations of NaCl (S) (2.5, 5.0, and 10 mM), H2O2 (OX) (1.0, 5.0, and 10.0 µM), and NaHCO3 (SA) (0.5, 1.0, and 2.5 mM) for 7 days and subjected to 50 mM Na, pH 8.25 for 14 days. the data represents means ± standard errors from five biological replicates. different letters indicate significant differences by tukey’s multiple comparison tests at P < 0.05.
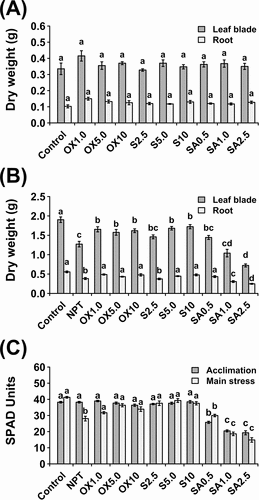
3.2. Acclimation to NaCl and H2O2 avert chlorosis under SAS conditions
At the end of the pre-treatment period, chlorophyll concentration was significantly reduced by NaHCO3 pre-treatment, whereas no differences were observed between controls, NaCl, and H2O2 PT plants (). After 14 days of main stress, chlorophyll concentration was significantly reduced in NPT and NaHCO3 PT seedlings. However, the chlorophyll concentration was unchanged in NaCl and H2O2 PT plants ().
3.3. NaCl and H2O2 pre-treatments suppressed rise of growth medium pH
During the onset of stress, growth medium pH generally progressively increased, reaching 8.45, by the end of 14 days of stress from the initial 8.0, in NPT plants (). In comparison to NPT, pre-treatments with NaCl were the most effective in suppressing growth medium pH (), followed by H2O2 pre-treatments (). Strikingly, PT with 1.0 and 2.5 mM NaHCO3 had considerably higher growth medium pH of up to 8.7, by the end of the 14-day stress period, whereas 0.5 mM NaHCO3 did not significantly change it ().
Figure 2. Rhizosphere acidification data showing daily pH changes in the growth medium during a 14-day period of main saline-alkaline stress (SAS) treatment in plants pre-treated with various concentrations of NaCl (A), H2O2 (B), and NaHCO3 (C) versus non-pre-treated seedlings. plants were pre-treated with various concentrations of NaCl (S) (2.5, 5.0, and 10 mM), H2O2 (OX) (1.0, 5.0, and 10.0 µM), and NaHCO3 (SA) (0.5, 1.0, and 2.5 mM) for 7 days and subjected to 50 mM Na, pH 8.25 for 14 days.
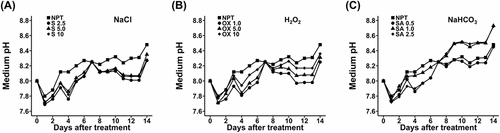
3.4. Pre-treatments regulate accumulation of K+, Na+ and p in roots and leaf blades
Significant reductions in K concentration were observed in both leaf blades and roots, which were particularly pronounced in roots (). However, in leaf blades, PT with 10 µM H2O2 and 0.5 mM NaHCO3 significantly increased K concentration, whereas, all pre-treatments significantly increased K concentration in roots (). This was coincidental with a significant increase in Na concentration, especially in the roots. In leaf blades, PT with 10 mM NaCl significantly reduced Na accumulation, as did NaHCO3, but H2O2 PT led to the highest accumulation of Na (). However, in roots 10 mM NaCl and 10 µM H2O2 pre-treatments significantly reduced the Na concentration. P concentration was significantly reduced in both leaves and roots, whereas NaHCO3 and H2O2 PT significantly increased it in roots ().
Figure 3. Macro and micronutrient analyses showing concentrations of potassium (A), sodium (B), phosphorus (C), iron (D), boron (E), and zinc (F) in leaf blades and roots after 14 days of exposure to main saline-alkaline stress (SAS) treatment. plants were pre-treated with 0 (NPT), 10 mM NaCl (S10), 10 µM H2O2 (OX10), and 0.5 mM NaHCO3 (SA0.5) for 7 days and subjected to 50 mM Na, pH 8.25 for 14 days. the data represents means ± standard errors from four biological replicates. different letters indicate significant differences by tukey’s multiple comparison tests at P < 0.05.
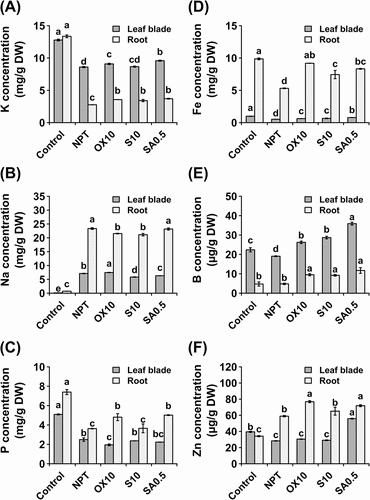
3.5. Stable micronutrient acquisition was critical for tolerance to SAS conditions
All three pre-treatments significantly increased Fe concentrations in both organs; however, this was most pronounced in roots (). The same was observed for boron (B) in both leaf blades and roots (). In leaf blades, Zn concentration was significantly reduced by SAS treatment, except in plants acclimated using 0.5 mM NaHCO3, which accumulated nearly two-fold higher Zn than NPT, NaCl, and H2O2 PT plants (), whereas, Zn concentration was significantly increased by SAS treatment in the roots of all PT plants ().
3.6. Root K+ leakage and Na+ efflux
Plant roots’ K+ leakage was extremely high under SAS conditions regardless of pre-treatments (> 60-folds higher) (). The efflux of Na+ was studied in a 4-hour period, and showed that saline-alkaline stress triggered significantly higher Na+ efflux (); however, this was more pronounced in pre-treated plants, particularly by 10 mM NaCl in part, elucidating its lower leaf blade and root Na+ concentrations.
Figure 4. Physiological experiments for studying K+ and Na+ homeostasis. K+ leakage in roots of 7-day pre-treated (PT) and non-pre-treated (NPT) seedlings subjected to 50 mM Na, pH 8.50 for 24 h (A). Efflux of Na+ from roots of 7-day PT and NPT seedlings, subjected to 50 mM Na, pH 8.50 for 24 h and deionized water for 2 h (B). Concentrations of Na+ (C) and chlorophyll (D) in excised leaves from 7-day PT and NPT plants subjected to 30 mM Na, pH 8.0 for 3 days. Plants were pre-treated with 0 (NPT), 10 mM NaCl (S10), 10 µM H2O2 (OX10), and 0.5 mM NaHCO3 (SA0.5) for 7 days and subjected to the above experiments. the data represents means ± standard errors from four biological replicates. different letters indicate significant differences by tukey’s multiple comparison tests at P < 0.05.
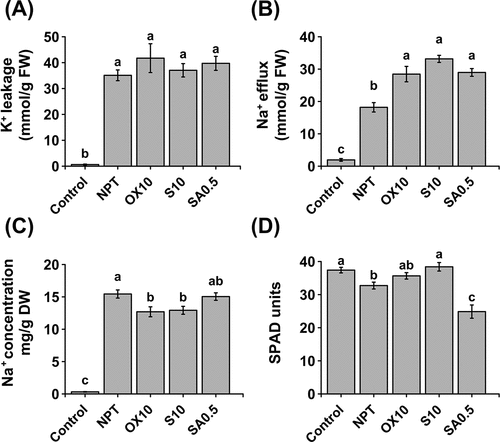
3.7. Na+ uptake in excised leaves
When we eliminated the ability of the plant roots to control Na+ uptake, 10 mM NaCl and 10 µM H2O2 significantly reduced leaf blade Na+ concentration, whereas NPT and 0.5 mM NaHCO3 PT plants accumulated significantly higher amounts of Na+ (). Chlorophyll concentration in the excised leaves showed significantly lower chlorophyll concentrations in NPT and 0.5 mM NaHCO3 PT leaf blades (), which could be attributed to the higher Na+ accumulation.
3.8. Oxidative stress, membrane integrity and cell viability
In this study, leaves of NPT plants exhibited considerably higher leakage of electrolytes (), whereas, no significant differences were detected in the roots of PT plants, albeit with higher values than leaf blades (). This was further verified by measurement of MDA, a marker of lipid peroxidation that showed significant reductions in leaf blades, particularly by NaCl and H2O2 pre-treatments, whereas, only NaCl PT reduced MDA levels in roots (). Cell viability was significantly enhanced in the leaf blades of all PT plants (). In the roots, cell viability was enhanced only by NaCl and H2O2 pre-treatments (). The H2O2 concentration was significantly reduced in leaf blades by 10 µM H2O2 and 0.5 mM NaHCO2 pre-treatments, whereas, the H2O2 concentration in roots was significantly reduced by all pre-treatments ().
Figure 5. Oxidative stress, membrane integrity, and cell viability parameters showing leakage of electrolytes (A), malondialdehyde concentrations (B), Evans Blue staining (C), and concentrations of hydrogen peroxide (D) in leaf blades and roots after 14 days of main saline-alkaline stress (SAS) treatment. Plants were pre-treated with 0 (NPT), 10 mM NaCl (S10), 10 µM H2O2 (OX10), and 0.5 mM NaHCO3 (SA0.5) for 7 days and subjected to 50 mM Na, pH 8.25 for 14 days. The data represents means ± standard errors from four biological replicates. different letters indicate significant differences by Tukey’s multiple comparison tests at P < 0.05.
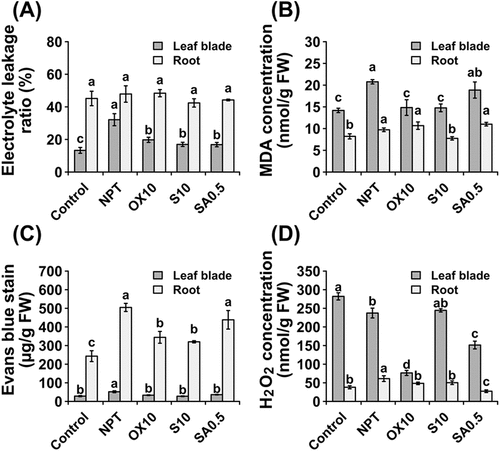
3.9. Enzymatic ROS antioxidant capacities
CAT activity was significantly increased in the leaf blades of NaCl and H2O2 PT plants, whereas it was significantly increased in the roots of NaCl and NaHCO3 PT plants (). APX activity was considerably increased in both the leaves and roots of NaCl and H2O2 PT plants (). Strikingly, the activities of APX were much higher in roots than in leaves (). Furthermore, GR activity was significantly induced in leaf blades; however, it was significantly induced only in PT plants (). In roots, no GR activity was detected in any of the samples. Finally, superoxide dismutase (SOD) activity was assayed; no significant differences in SOD activity were observed in the leaf blades among PT plants, except for H2O2 pre-treatment, which considerably decreased its activity (). However, SOD activity was significantly increased in the roots of PT plants. In NPT plants, SOD activity was considerably suppressed (). In leaf blades, NPT and NaCl PT plants had significantly higher protein concentrations, whereas, in roots, NaHCO3 PT plants had the highest protein concentration. Comparatively, the protein concentration was significantly higher in leaf blades than in roots ().
Figure 6. Antioxidative enzyme activities showing activities of catalase (A), guaiacol peroxidase (B), ascorbate peroxidase (C), glutathione reductase (D), and superoxide dismutase (E) and protein concentration (F) in leaf blades and roots after 14 days of exposure to main saline-alkaline stress (SAS) treatment. Plants were pre-treated with 0 (NPT), 10 mM NaCl (S10), 10 µM H2O2 (OX10), and 0.5 mM NaHCO3 (SA0.5) for 7 days and subjected to 50 mM Na, pH 8.25 for 14 days. The data represents means ± standard errors from four biological replicates. Different letters indicate significant differences by Tukey’s multiple comparison tests at P < 0.05.
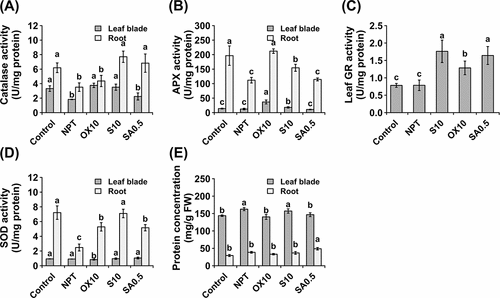
3.10. H2O2 pre-treatment facilitates a quicker recovery upon withdrawal of SAS conditions
The control plants grew approximately three times faster during recovery than during the stress period (-)). However, in previously stressed plants, despite subjecting them to similar recovery conditions, growth rates did not significantly change. However, both shoot and root growth rates were considerably increased in H2O2 PT plants (-)). Therefore, 10 µM H2O2 PT facilitates quicker recovery and resumption of growth when stress is concluded. Strikingly, in roots, NaHCO3 PT significantly reduced the root mean growth rate during recovery (), suggesting that irreparable damage was inflicted in NaHCO3 PT and NPT plants. Chlorophyll concentration was recovered in PT plants but not in NPT plants (). Furthermore, membrane integrity was recovered in all plants, regardless of PT (). These observations imply that during the recovery period, some plants could physiologically recover, despite the inability of some plants to resume rapid growth.
Figure 7. Effect of pre-treatments on recovery of plants after exposure to saline-alkaline stress. Comparative mean growth rates during and after stress in shoots (A) and roots (B). Chlorophyll concentration (C) and membrane integrity assessed by the electrolyte leakage method in shoots (D) at the end of the 10-day recovery period. ‘Stress’ group represents mean growth rate of plants during the 14 day growth period under main saline-alkaline stress (SAS) (50 mM Na+, pH 8.25) after pre-treatment with 0 (NPT), 10 mM NaCl (S10), 10 µM H2O2 (OX10), and 0.5 mM NaHCO3 (SA0.5) for 7 days. ‘Recovery’ group represents the growth rate of the same plants upon completion of main saline-alkaline stress (SAS) and subjecting them to recovery under normal conditions (0 mM Na+, pH 5.5) for 10 days. The data represents means ± standard errors from five biological replicates. different letters indicate significant differences by tukey’s multiple comparison tests at P < 0.05.
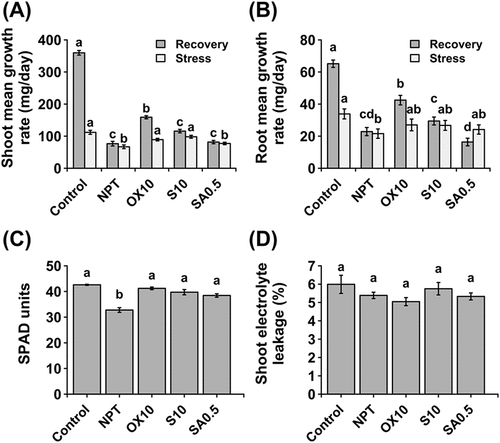
4. Discussion
Rice is extremely sensitive to salt stress, although it is one of the prominent staples in the world. Hence, it remains imperative to identify quicker, yet effective means to increase rice growth and yield in such environments. Plants are adept at acclimating to salt stress, characterized by quicker and stronger responses to subsequent lethal exposure to the same stress (Kamanga et al. Citation2020; Liu and Saneoka Citation2019; Sriskantharajah et al. Citation2020). While acclimation studies are widespread, the physiological processes governing responses of plants to multiple exposures of different stresses are unclear. Here, we have shown that plants pre-exposed to lower concentrations of NaCl and H2O2 show improved growth and physiological characteristics under SAS conditions. Contrary to the hypothesis, the failure of NaHCO3 pre-treatment to enhance growth suggests that the concentrations used were too high for pre-treatment in rice, likely causing physiological injuries. However, a similar concentration of 1 mM NaHCO3 in Rye (Secale cereale) effectively improved tolerance to SAS conditions (Liu and Saneoka Citation2019). Overall, 10 mM NaCl and 10 µM H2O2 were the most effective (). These latter results illustrate the concept of cross-tolerance, wherein pre-exposure to one stress, such as salinity or oxidative stress, promotes tolerance to another stress, such as saline-alkaline stress, as previously described (Foyer et al. Citation2016; Perez and Brown Citation2014). In light of a myriad of abiotic stresses as currently faced, this critical attribute offers a prospect for generating plants tolerant to multiple abiotic stresses.
Under SAS conditions, the first line of defense entails acidification of the rhizosphere through H+ ATPase-mediated proton efflux by plant roots (Li, Yang, and Zhang Citation2016). In the present study, the failure of NaHCO3 PT plants to suppress rise in growth medium pH () may partly elucidate their inability to effectively promote tolerance to SAS conditions (). In contrast, plants pre-treated with NaCl and H2O2 maintained a much lower growth medium pH throughout the growth period compared to the NPT plants, indicative of higher root activity. Lower rhizospheric pH is also advantageous for maintaining the transmembrane proton gradient required for SOS-mediated efflux of Na+ by roots SOS1 (Cao et al. Citation2020; Moriyama Citation2015). Experiments on Na+ efflux showed that all pre-treated plants had higher root Na+ efflux than non-pre-treated () including NaHCO3. Therefore, whether higher root Na+ efflux and resultant lower leaf blade Na+ accumulation () were as a result of lower growth medium pH are difficult to untangle. Furthermore, when we eliminated the contribution of Na+ uptake by roots using experiments on Na+ accumulation in excised leaf blades, both 10 mM NaCl and 10 µM H2O2 PT plants accumulated significantly lower Na in the leaf blades (). In H2O2 PT plants, notwithstanding higher leaf blade Na+ accumulation, the deleterious effects of Na+ were ameliorated and leaf photosynthetic competency () was preserved. Moreover, despite the ‘statistical’ significance of leaf blade Na+ accumulation among PTs, this result suggests that the observed differences may not have been ‘biologically significant.’
Na and K have similar physicochemical properties; hence, they exhibit competitive uptake systems (Marschner and Marschner Citation2012). Moreover, rice plant roots are leaky (Munns and Tester Citation2008), potentially losing significant amounts of K+ under SAS conditions. Retention of root K+ has been cited as a principal trait for salt tolerance (Chen et al. Citation2007). In the present study, pre-treatment with NaCl and H2O2 maintained a higher root K concentration (). Separate experiments revealed extremely high root K+ leakage under SAS (), which could however not be alleviated by pre-treatments. Therefore, while root K+ leakage was the primary cause of K deficiency under SAS, its contribution to the observed differences among various pre-treatments could not be verified. Nonetheless, considering the extreme loss of K+ in roots under SAS conditions, these results suggest that even the slightest improvements in root K+ retention may be beneficial under saline-alkaline stress, primarily for osmotic adjustments and maintenance of root growth as also suggested by Chen et al. (Citation2007). Additional benefits of acclimation included increased P uptake and accumulation especially in roots. Uptake of P is driven by H+ gradient across the plasma membrane, such that when the rhizospheric pH is lower than root cytosolic pH, P uptake is promoted (Cao et al. Citation2020). Hence, under saline-alkaline stress, maintenance of P uptake is an extremely important attribute for plant growth and development, and also dependant on plant roots ability to lower rhizospheric pH through H+ ATPases mediated proton efflux. However, further studies are required to elucidate the physiological mechanisms underlying this enhanced P uptake.
Fe deficiency, which is a typical feature of plants growing under high pH conditions, is characterized by chlorosis. Although iron is a naturally abundant element, it forms insoluble ferric compounds that are non-bioavailable (Marschner Citation1995; Nakanishi et al. Citation2004) for uptake under high pH conditions. As shown in , NPT plant leaves were chlorotic, which is a typical sign of Fe deficiency (Marschner and Marschner Citation2012). However, in NaCl and H2O2 PT plants, leaves were healthier () and accumulated higher Fe concentrations (). Therefore, it is tempting to attribute the high Fe concentration to the ability of plant roots to suppress rise in growth medium pH, as observed in , B. Lower growth medium pH is thought to increase the solubility of ferric ions or support the reducing capacity of ferric Fe on the root surface (Kobayashi and Nishizawa Citation2012). However, similar increases in Fe concentration were also observed in NaHCO3 pre-treated plants () which otherwise did not show different growth medium pH from NPT plants (), raising doubts on the causality between Fe concentration and lower rhizospheric pH. Alternative causes for the variations in Fe uptake could relate to differences in key enzyme activities involved in Fe uptake (Nakanishi et al. Citation2004; Kobayashi and Nishizawa Citation2012), however, further studies to elucidate the physiological and molecular bases of this acclimation induced increase in accumulation are imperative. In NaHCO3 pre-treated plants (SA0.5), despite having higher leaf blade and root Fe concentration (), leaf blades were relatively chlorotic (), similar to non-pre-treated plants, inconsistent with our expectations. This might have been as a result of considerably reduced chlorophyll concentration during the 7-day acclimation period (), such that enhanced Fe uptake and accumulation during the main stress could not fully recover chlorophyll concentration by the sampling time.
Several reports have implicated the lethality of SAS to ROS-mediated damage. In an alkaline tolerant (ALT1) rice mutant, Guo et al. (Citation2014) credited tolerance to enhanced defense against oxidative stress. Oxidative damage to membrane lipids causes peroxidation, which is analytically deduced from the concentration of malondialdehyde (MDA), an aldehyde by-product of lipid peroxidation (Ayala, Muñoz, and Argüelles Citation2014). In the present study, NaCl and H2O2 pre-treatments considerably alleviated SAS-induced membrane damage and cell death (–)). These results, in part, indicate mitigated oxidative stress, owing to the enhanced ROS scavenging capacity in PT plants. ROS generation in plants is an inevitable consequence of aerobic metabolism. Until recently, the traditional paradigm that considers ROS as deleterious to plant cells is gradually fading owing to recent revelations of their critical role as secondary messengers (Apel and Hirt Citation2004; Mittler Citation2017). We hypothesized that pre-treatments would spark a mild generation of ROS, which would eventually activate systemic antioxidant defense responses. In agreement, H2O2 generation was significantly reduced in roots in all PT plants, whereas only H2O2 and NaHCO3 pre-treatments were effective in leaf blades. In NaCl PT plants, despite having exceptional growth and lower Na concentration in leaf blades, the higher H2O2 in tandem cautions against the inevitable expectation that ROS results in poor growth, harmonizing with notions earlier expressed in Mittler (Citation2017). Perhaps the crucial aspect is maintaining a delicate balance between ROS generation and detoxification. Given that NaCl PT plants had higher antioxidant activities of CAT, APX, and GR (– ), this balance might have been attained, cushioning against vicious functions of ROS (Apel and Hirt Citation2004). Better antioxidant enzyme activities were also observed in H2O2 PT plants, in agreement with earlier observations (Fedina, Nedeva, and Çiçek Citation2009; Wahid et al. Citation2007). Moreover, all pre-treatments considerably increased the SOD activity (). Since H2O2 and hydroxyl radicals are superoxide radical derivatives, SOD offers a first line of defense against oxidative stress; hence, acclimation provided nearly complete protection against oxidative stress. Besides, H2O2 concentration () did not essentially correspond to the trends of electrolyte leakage, MDA concentration, and cell viability (–)) in some PTs, in part cementing the above notions. Apparently, this may be ascribed to different antioxidant enzyme capacities (–)) or capacities to repair ROS mediated damage. Strategies adopted by plants to confront oxidative stress mediated damage engage three lines of steps; avoidance of ROS generation, detoxification of ROS (scavenging) and repair of ROS mediated damage (Guo et al. Citation2014; Mittler Citation2017). Hence, measurement of ROS generation alone may not sufficiently predict resultant ROS mediated damage. Furthermore, this result may point to involvement of other ROS, such as superoxide and hydroxyl radicles that were otherwise not assayed in the present study.
In conclusion, the present study reports that acclimation to salinity stress or oxidative stress promotes cross-tolerance to SAS conditions by fostering efficient Na+ homeostasis, Fe acquisition, and ROS homeostasis. These processes are linked to the ability of plant roots to supress excessive increases in growth media pH, offering a first line of defense against SAS. We further suggest crosstalk between salinity stress, oxidative stress, and saline-alkalinity, likely functioning through common signals and pathways that trigger adaptive physiological responses, thereby developing cross-resistance to saline-alkalinity. Moreover, H2O2 pre-treated plants quickly recover physiologically and resume rapid growth upon the conclusion of stress (). This may be related to lower accumulation of H2O2 in the leaf blades (), as a result of higher antioxidant enzyme activities specifically CAT, APX and GR (–)) that detoxify cellular H2O2. Rapid resumption of growth after withdrawal of a stressor is apparently a fundamental attribute for recurrent stresses with prolonged recovery periods. Therefore, pre-treatment with optimal NaCl and H2O2 may be a recommended option for quick improvements in tolerance to SAS conditions in rice. This trait also offers a prospect for developing crop plants tolerant to multiple stressors.
Acknowledgments
This research was supported by JSPS KAKENHI Grant Numbers 15KK0283 and 20KK0129 to AU.
Disclosure statement
No potential conflict of interest was reported by the author(s).
Additional information
Funding
References
- Apel, K., and H. Hirt. 2004. “Reactive Oxygen Species: Metabolism, Oxidative Stress, and Signal Transduction.” Annual Review of Plant Biology 55 (1): 373–399. doi:10.1146/annurev.arplant.55.031903.141701.
- Atkinson, N. J., and P. E. Urwin. 2012. “The Interaction of Plant Biotic and Abiotic Stresses: From Genes to the Field.” Journal of Experimental Botany 63 (10): 3523–3544. doi:10.1093/jxb/ers100.
- Ayala, A., M. F. Muñoz, and S. Argüelles. 2014. “Lipid Peroxidation: Production, Metabolism, and Signaling Mechanisms of Malondialdehyde and 4-Hydroxy-2-Nonenal.” Oxidative Medicine and Cellular Longevity 2014: 360438. doi:10.1155/2014/360438.
- Beauchamp, C., and I. Fridovich. 1971. “Superoxide Dismutase: Improved Assays and an Assay Applicable to Acrylamide Gels.” Analytical Biochemistry 44 (1): 276–287. doi:10.1016/0003-2697(71)90370-8.
- Bradford, M. M. 1976. “A Rapid and Sensitive Method for the Quantitation of Microgram Quantities of Protein Utilizing the Principle of Protein-Dye Binding.” Analytical Biochemistry 72 (1–2): 248–254. doi:10.1016/0003-2697(76)90527-3.
- Cao, Y., M. Zhang, X. Liang, F. Li, Y. Shi, X. Yang, and C. Jiang. 2020. “Natural Variation Af an EF-Hand Ca2+-Binding-Protein Coding Gene Confers Saline-Alkaline Tolerance in Maize.” Nature Communications 11: 1–14.
- Chen, Z., I. I. Pottosin, T. A. Cuin, A. T. Fuglsang, M. Tester, D. Jha, and S. Shabala. 2007. “Root Plasma Membrane Transporters Controlling K+/Na+ Homeostasis in Salt Stressed Barley.” Plant Physiology 145 (4): 1714–1725. doi:10.1104/pp.107.110262.
- Chuamnakthong, S., M. Nampei, and A. Ueda. 2019. “Characterization of Na+ Exclusion Mechanism in Rice under Saline-Alkaline Stress Conditions.” Plant Science 287: 110171. doi:10.1016/j.plantsci.2019.110171.
- Farooq, S., and F. Azam. 2006. “The Use of Cell Membrane Stability (CMS) Technique to Screen for Salt Tolerant Wheat Varieties.” Journal of Plant Physiology 163 (6): 629–637. doi:10.1016/j.jplph.2005.06.006.
- Fedina, I. S., D. Nedeva, and N. Çiçek. 2009. “Pre-treatment with H2O2 Induces Salt Tolerance in Barley Seedlings.” Biologia Plantarum 53 (2): 321–324. doi:10.1007/s10535-009-0058-3.
- Foyer, C. H., B. Rasool, J. W. Davey, and R. D. Hancock. 2016. “Cross-Tolerance to Biotic and Abiotic Stresses in Plants: A Focus on Resistance to Aphid Infestation.” Journal of Experimental Botany 67 (7): 2025–2037. doi:10.1093/jxb/erw079.
- Guo, M., R. Wang, J. Wang, K. Hua, Y. Wang, X. Liu, and S. Yao. 2014. “ALT1, a Snf2 Family Chromatin Remodeling ATPase, Negatively Regulates Alkaline Tolerance through Enhanced Defense against Oxidative Stress in Rice.” PLoS ONE 9 (12): e112515. doi:10.1371/journal.pone.0112515.
- Haruta, M., and M. R. Sussman. 2012. “The Effect of a Genetically Reduced Plasma Membrane Protonmotive Force on Vegetative Growth of Arabidopsis.” Plant Physiology 158 (3): 1158–1171. doi:10.1104/pp.111.189167.
- Hodges, D. M., J. M. DeLong, C. F. Forney, and R. K. Prange. 1999. “Improving the Thiobarbituric Acid-Reactive-Substances Assay for Estimating Lipid Peroxidation in Plant Tissues Containing Anthocyanin and Other Interfering Compounds.” Planta 207 (4): 604–611. doi:10.1007/s004250050524.
- Jia, B., M. Sun, H. Duanmu, X. Ding, B. Liu, Y. Zhu, and X. Sun. 2017. “GsCHX19.3, A Member of Cation/H+ Exchanger Superfamily from Wild Soybean Contributes to High Salinity and Carbonate Alkaline Tolerance.” Scientific Reports. 7
- Kamanga, R. M., K. Echigo, K. Yodoya, A. M. M. Mekawy, and A. Ueda. 2020. “Salinity Acclimation Ameliorates Salt Stress in Tomato (Solanum Lycopersicum L.) Seedlings by Triggering a Cascade of Physiological Processes in the Leaves.” Scientia Horticulturae 270: 109434. doi:10.1016/j.scienta.2020.109434.
- Kobayashi, T., and N. K. Nishizawa. 2012. “Iron Uptake, Translocation, and Regulation in Higher Plants.” Annual Review of Plant Biology 63 (1): 131–152. doi:10.1146/annurev-arplant-042811-105522.
- Li, Q., A. Yang, and W. H. Zhang. 2016. “Efficient Acquisition of Iron Confers Greater Tolerance to Saline-alkaline Stress in Rice (Oryza Sativa L.).” Journal of Experimental Botany 67 (22): 6431–6444. doi:10.1093/jxb/erw407.
- Liu, L., and H. Saneoka. 2019. “Effects of NaHCO3 Acclimation on Rye (Secale Cereale) Growth under Sodic-Alkaline Stress.” Plants 8 (9): 314. doi:10.3390/plants8090314.
- Marschner, H. 1995. “Adaptation of Plants to Adverse Chemical Soil Conditions.” In Mineral Nutrition of Higher Plantsedited by Horst Marschner. 596-680. Cambridge, Massachusetts: Academic Press.
- Marschner, H., and P. Marschner. 2012. Marschner’s Mineral Nutrition of Higher Plants. Cambridge, Massachusetts: Academic Press.
- Masuda, H., M. Suzuki, K. C. Morikawa, T. Kobayashi, H. Nakanishi, M. Takahashi, and N. K. Nishizawa. 2008. “Increase in Iron and Zinc Concentrations in Rice Grains via the Introduction of Barley Genes Involved in Phytosiderophore Synthesis.” Rice 1 (1): 100–108. doi:10.1007/s12284-008-9007-6.
- Masuda, H., K. Usuda, T. Kobayashi, Y. Ishimaru, Y. Kakei, M. Takahashi, and N. K. Nishizawa. 2009. “Overexpression of the Barley Nicotianamine Synthase Gene HvNAS1 Increases Iron and Zinc Concentrations in Rice Grains.” Rice 2 (4): 155–166. doi:10.1007/s12284-009-9031-1.
- Mekawy, A. M. M., M. N. Abdelaziz, and A. Ueda. 2018. “Apigenin Pretreatment Enhances Growth and Salinity Tolerance of Rice Seedlings.” Plant Physiology and Biochemistry 130: 94–104. doi:10.1016/j.plaphy.2018.06.036.
- Mittler, R. 2017. “ROS are Good.” Trends in Plant Science 22 (1): 11–19. doi:10.1016/j.tplants.2016.08.002.
- Moriyama, Y. 2015. „Vacuolar H+-ATPase and the Secondary Transporters: Their Identification, Mechanism, Function and Physiological Relevance.„ Yakugaku Zasshi : Journal of the Pharmaceutical Society of Japan 135 (7): 883–894. doi:10.1248/yakushi.15-00081.
- Munns, R., and M. Tester. 2008. “Mechanisms of Salinity Tolerance.” Annual Review of Plant Biology 59 (1): 651–681. doi:10.1146/annurev.arplant.59.032607.092911.
- Nakanishi, H., N. K. Nishizawa, S. Kim, M. Takahashi, N. K. Nishizawa, N. K. Nishizawa, and H. Yamaguchi. 2004. “Directed Evolution of Yeast Ferric Reductase to Produce Plants with Tolerance to Iron Deficiency in Alkaline Soils.” Soil Science and Plant Nutrition 50 (7): 1159–1165. doi:10.1080/00380768.2004.10408589.
- Pandolfi, C., E. Azzarello, S. Mancuso, and S. Shabala. 2016. “Acclimation Improves Salt Stress Tolerance in Zea Mays Plants.” Journal of Plant Physiology 201: 1–8. doi:10.1016/j.jplph.2016.06.010.
- Pandolfi, C., S. Mancuso, and S. Shabala. 2012. “Physiology of Acclimation to Salinity Stress in Pea (Pisum Sativum).” Environmental and Experimental Botany 84: 44–51. doi:10.1016/j.envexpbot.2012.04.015.
- Pastori, G. M., and C. H. Foyer. 2002. “Common Components, Networks, and Pathways of Cross-Tolerance to Stress. The Central Role of “Redox” and Abscisic Acid-Mediated Controls.” Plant Physiology 129 (2): 460–468. doi:10.1104/pp.011021.
- Perez, I. B., and P. J. Brown. 2014. “The Role of ROS Signaling in Cross-Tolerance: From Model to Crop.” Frontiers in Plant Science 5: 1–6. doi:10.3389/fpls.2014.00754.
- Shabala, S., S. Shabala, T. A. Cuin, J. Pang, W. Percey, Z. Chen, and L. H. Wegner. 2010. “Xylem Ionic Relations and Salinity Tolerance in Barley.” The Plant Journal 61 (5): 839–853. doi:10.1111/j.1365-313X.2009.04110.x.
- Sriskantharajah, K., S. Osumi, S. Chuamnakthong, M. Nampei, J. C. Amas, G. B. Gregorio, and A. Ueda. 2020. “Contribution of Two Different Na+ Transport Systems to Acquired Salinity Tolerance in Rice.” Plant Science 297: 110517. doi:10.1016/j.plantsci.2020.110517.
- Suharsono, U., Y. Fujisawa, T. Kawasaki, Y. Iwasaki, H. Satoh, and K. Shimamoto. 2002. “The Heterotrimeric G Protein Subunit Acts Upstream of the Small GTPase Rac in Disease Resistance of Rice.” Proceedings of the National Acadamy of Sciences of the United States of America 99 (20): 13307–13312. doi:10.1073/pnas.192244099.
- Suzuki, M., K. C. Morikawa, H. Nakanishi, M. Takahashi, M. Saigusa, S. Mori, and N. K. Nishizawa. 2008. “Transgenic Rice Lines that Include Barley Genes Have Increased Tolerance to Low Iron Availability in a Calcareous Paddy Soil.” Soil Science and Plant Nutrition 54 (1): 77–85. doi:10.1111/j.1747-0765.2007.00205.x.
- Takagi, H., and S. Yamada. 2013. “Roles of Enzymes in Anti-Oxidative Response System on Three Species of Chenopodiaceous Halophytes under NaCl-Stress Condition.” Soil Science and Plant Nutrition 59 (4): 603–611. doi:10.1080/00380768.2013.809600.
- Umezawa, T., K. Shimizu, M. Kato, and T. Ueda. 2000. “Enhancement of Salt Tolerance in Soybean with NaCl Pretreatment.” Physiologia Plantarum 110 (1): 59–63. doi:10.1034/j.1399-3054.2000.110108.x.
- Wahid, A., M. Perveen, S. Gelani, and S. M. A. Basra. 2007. “Pretreatment of Seed with H2O2 Improves Salt Tolerance of Wheat Seedlings by Alleviation of Oxidative Damage and Expression of Stress Proteins.” Journal of Plant Physiology 164 (3): 283–294. doi:10.1016/j.jplph.2006.01.005.
- Yang, C. W., H. H. Xu, L. L. Wang, J. Liu, D. C. Shi, and D. L. Wang. 2009. “Comparative Effects of Salt-Stress and Alkali-Stress on the Growth, Photosynthesis, Solute Accumulation, and Ion Balance of Barley Plants.” Photosynthetica 47 (1): 79–86. doi:10.1007/s11099-009-0013-8.
- Yang, Z., C. Wang, Y. Xue, X. Liu, S. Chen, C. P. Song, and Y. Guo. 2019. “Calcium-activated 14-3-3 Proteins as a Molecular Switch in Salt Stress Tolerance.” Nature Communications 10: 1–9.
- Zhang, H., X. L. Liu, R. X. Zhang, H. Y. Yuan, M. M. Wang, H. Y. Yang, and Z. W. Liang. 2017. “Root Damage under Alkaline Stress Is Associated with Reactive Oxygen Species Accumulation in Rice (Oryza Sativa L.).” Frontiers in Plant Science 8: 1580. doi:10.3389/fpls.2017.01580.
- Zhang, Z., K. He, T. Zhang, D. Tang, R. Li, and S. Jia. 2019. “Physiological Responses of Goji Berry (Lycium Barbarum L.) To Saline-Alkaline Soil from Qinghai Region, China.” Scientific Reports 9 (1): 12057. doi:10.1038/s41598-019-48514-5.