ABSTRACT
The effect of silicon (Si) was studied in strawberry (Fragaria × ananassa) plants grown under greenhouse conditions and harvested at vegetative, flowering and fruiting stages. Vegetative growth and fruit dry weight were improved by Si. The concentration of carbohydrates decreased in the leaves but increased in the roots upon Si treatment. However, the level of the soluble protein pool increased in both leaves and roots at the expense of free amino acids at all three developmental stages. Analyses of phenolics by HPLC and assay of enzyme activity in both soluble and cell wall-bound fractions of the leaves, roots and fruits indicated that Si significantly modified the profile of phenolics and the activity of metabolising enzymes at all three developmental stages. All fruit quality parameters were remarkably improved by Si. According to our results, Si improves both quantitative and qualitative parameters in strawberries through stimulation of growth and modification of phenolics metabolism.
Introduction
Silicon (Si) is the second most abundant element in the earth’s crust, but it is not necessary for growth and metabolism in higher plants. As a beneficial element, Si is involved in the amelioration of various stresses, such as drought and salinity (Hajiboland Citation2012; Zhu and Gong Citation2014). In addition, Si can improve plant performance under optimal growth conditions. In tobacco, an improvement of water-relation parameters by Si helps plants to maintain higher turgor pressure supporting higher rates of leaf expansion and root elongation. This, in turn, leads to higher whole-plant photosynthesis and enhanced capacity for water and nutrient capture by the roots (Hajiboland et al. Citation2017b). Since Si applications are frequently related to stimulation of enzymatic defence strategies involved in detoxification of reactive oxygen species (Liu et al. Citation2009; Hajiboland et al. Citation2017b), its promoting effects could also be attributed to minimising the effects of latent stress factors, such as light and/or temperature stresses, a water or oxygen deficit and latent pathogen infection.
The stimulation of plant growth and performance under non-stressful conditions may also be primarily attributed to improved carbon and nitrogen metabolism. Enhancement of photosynthesis provides extra energy and carbon skeletons for other anabolic pathways requiring ATP and reducing equivalents, including nutrient assimilation. In addition, elevated protein synthesis is required for achievement and maintenance of a higher growth rate (Lawlor Citation2002; Nunes-Nesi et al. Citation2010). The growth-promoting effect of selenium, another beneficial element, observed particularly in Brassicaceae under optimum environmental and nutritional conditions, is mainly associated with activation of carbon and nitrogen metabolism (Malik et al. Citation2011; Hajiboland and Sadeghzadeh Citation2014).
The phenylpropanoid pathway in plants is responsible for the synthesis of a large variety of phenolic metabolites, including phenol esters, coumarins, flavonoids and lignin (Weaver and Herrmann Citation1997). All phenylpropanoids are derived from trans-cinnamic acid which is formed from l-phenylalanine by the action of phenylalanine ammonia lyase (PAL), the key enzyme in the biosynthesis of the phenolic compounds. Polyphenol oxidase (PPO) catalyses the oxidation of polyphenols and the hydroxylation of monophenols and lignification of plant cells. Peroxidases (POD) are involved in a broad range of biochemical processes, such as lignin and suberin formation, cross-linking of cell wall (CW) components and defence against pathogens (Siegel Citation1993; Weaver and Herrmann Citation1997). Increasing evidence shows that Si influences the concentrations of phenolic compounds and activity of metabolising enzymes (Maksimović et al. Citation2007; Schaller et al. Citation2012; Hajiboland et al. Citation2017a).
Strawberry (Fragaria × ananassa Duch.) is one of the most popular and widely consumed berries worldwide. Its fruit is exceptionally rich in phenolic compounds, such as flavonols (kaempferol and quercetin derivatives), anthocyanidins (cyanidin and pelargonidin derivatives), proanthocyanidins, hydrolysable tannins (galloylglucoses and ellagitannins) and phenolic acids (Amil-Ruiz et al. Citation2011; Giampieri et al. Citation2014). Research works on strawberry plants have mainly been undertaken on fruits and relatively little is known about the phytochemical constituents of leaves and roots.
The strawberry has been identified as a Si accumulator species (Miyake and Takahashi Citation1986). However, physiological and biochemical effects of Si have been poorly characterised in this species. Detailed information is also needed on dynamic changes in the structure and composition of the CW together with a functional analysis of CW-modifying enzymes as affected by Si in this fruit crop.
Our working hypothesis was that Si supplementation positively impacts the plants carbon and nitrogen metabolism and widely influences its phenolics quantity and quality. In this work, in addition to carbohydrates and nitrogenous compounds, the phenolics profile and metabolism were studied in the leaves and roots at three developmental stages. The effect of Si on the fruit quality parameters and phenolics profile was also studied at the full-red stage in this Si accumulator species.
Materials and methods
Plants culture and treatments
Strawberry (F. × ananassa cv. Paros) plants were provided as rooted daughter plants by the Horticulture Department, Ferdowsi University of Mashhad, Iran. The young daughter plants with two expanded leaves were transferred to 3-L plastic pots filled with washed perlite and one plant was cultivated in each pot. The pots were irrigated daily at 85% field capacity after weighing with Hoagland nutrient solution (pH 5.8–6.0) or water at intervals. The total volume of nutrient solution applied to each pot started with 50 mL pot−1 week−1 and reached 250 mL pot−1 week−1 at the fruiting stage. To avoid the accumulation of salts in the substrate, electric conductivity in the perlite was measured in samples taken weekly from the bottom of the pots.
One week after transplanting, Si (Na2SiO3, Sigma) treatment was started. The Si solution (adjusted to pH 6.1 using HCl) was added gradually to the pots by irrigation within three weeks to reach 3 mmol L−1 perlite (∼84 mg L−1 perlite) for vegetative plants. For flowering and fruiting plants, Si treatment was continued and reached the final concentration of 5 and 7 mmol L−1 perlite (∼140 and 196 mg L−1 perlite) at harvest, respectively (for details, please see ). The content of water-soluble Si in the used perlite substrate before planting was 336 ± 128 µg g−1 perlite dry weight.
Plants were grown under controlled environmental conditions with a temperature regime of 25°/18°C day/night, 14/10 h light/dark period, a relative humidity of 50–60% and at a photon flux density of about 400 µmol m−2 s−1.
Harvest of plants
Plants were harvested at three developmental stages, namely vegetative, flowering and fruiting stage, i.e. 6, 10 and 14 weeks after transfer of daughter plants to the pots. Harvest of fruiting stage was performed when fruits were at the full-red stage. Shoots and roots were separated, washed with distilled water, blotted dry on filter paper and weighed. For biochemical assays, mature leaflets, young light-coloured roots and full-red fruits (for fruiting plants) were harvested, weighed and stored in liquid nitrogen before analyses.
Determination of Si, zinc and manganese
For determination of zinc (Zn) and manganese (Mn), the dried leaf material was ashed in a muffle furnace at 500°C for 8 h. After cooling, the samples were extracted twice with 2.5 mL of 3.4 M HNO3 until dryness. The ash was dissolved in 2.5 mL of 4 M HCl, subsequently diluted 10 times with hot deionised water and boiled for 2 min. Concentrations of Zn and Mn were measured by atomic absorption spectrometry (UNICAM 939, Germany). Silicon was determined by a colorimetric method using ammonium molybdate after autoclave-induced digestion (Elliott and Snyder Citation1991).
Determination of carbon and nitrogen compounds
For determination of carbohydrates, leaf and root samples were homogenised in 100 mmol L−1 phosphate buffer (pH 7.5) at 4°C. After centrifugation at 12,000g for 15 min, the supernatant was used for determination of total soluble sugars whereas the pellets were kept for starch analysis (Yemm and Willis Citation1954). Total soluble protein was determined using a commercial reagent (Sigma) and bovine serum albumin as standard. Total free α-amino acid content was assayed using a ninhydrin colorimetric method (Yemm et al. Citation1955). Glycine (Merck) was used for the production of a standard curve.
Assay of enzyme activities
The activity of enzymes was assayed according to the methods described previously (Hajiboland et al. Citation2013). PAL (EC 4.3.1.5) activity was expressed as the rate of conversion of l-phenylalanine to trans-cinnamic acid. PPO (EC 1.14.18.1) activity was determined by following the change in the absorbance (ΔAbs) at 334 nm due to oxidation of pyrogallol. POD (EC 1.11.1.7) was extracted and the activity was assayed with guaiacol for the soluble fraction and with syringaldazine for the CW-bound fraction of POD (Hajiboland et al. Citation2013).
Determination of soluble and CW-bound phenolics
Leaf and root samples were used for extraction of water-soluble and CW-bound phenolics, according to the method described elsewhere (Hajiboland et al. Citation2013). Phenolic contents in the cytoplasmic and CW-bound fractions were determined either spectrophotometrically at 750 nm using Folin–Ciocalteu reagent and gallic acid as standard (Swain and Hillis Citation1959) or with HPLC, as described below.
Analyses of phenolic compounds using HPLC
The qualitative and quantitative profiles of phenolic compounds were studied using an HPLC system, Agilent Technologies SL 1200 Series (Waldbronn, Germany) equipped with a binary pump and UV–Vis detector and a reversed-phase Hypersil Gold C18 column (5 µm particle size, 250 * 4.6 mm). The mobile phase consisted of 1% aqueous acetic acid solution and methanol. Chromatograms were acquired at four different wavelengths (254, 278, 300 and 370 nm), according to absorption maxima of analysed compounds. Each compound was identified by its retention time and by spiking with standards under the same conditions. The identities of constituents were also confirmed with a photodiode array detector by comparison with UV spectra of the standards (purchased from Sigma-Aldrich) in the wavelength range of 220–450 nm. Two independent analyses were performed within each biological replicate (Nour et al. Citation2013).
Evaluation of fruit quality parameters
Evaluation of free radical-scavenging activity of fruit extract was performed using the DPPH (2,2-diphenyl-1-picrylhydrazyl) quenching efficacy index calculated on the basis of the observed decrease of the absorbance in the presence of fruit extract relative to a blank sample (Panico et al. Citation2009). Determination of ascorbic acid was undertaken using dinitrophenylhydrazine (DNP) reagent (DNP-thiourea-copper sulphate) at 520 nm and concentration was calculated using a standard curve for ascorbic acid (Sigma) created in the range of 0.002–200 mg L−1 (Klopotek et al. Citation2005). Titratable acidity of fruit extract was obtained through titration of 10 mL juice with 0.1 mmol L−1 NaOH to a pH of 8.2–8.3. The results were expressed as citric acid equivalents (Helrich Citation1990).
Statistical analyses
The experimental design was a complete randomised block with four independent pots (one plant per pot) as four biological replicates. The Sigma stat (3.02) was used for statistical analysis using Tukey’s test (P < .05).
Results and discussion
Effect of Si on the growth and fruit production
Shoot and root dry weights were improved by Si supplementation. This effect was significant for root dry weight at all three developmental stages, while for shoot dry weight, a significant influence was observed only at the vegetative stage. Fruit weight was slightly improved by Si treatment (). This effect observed under conditions assumed to be optimal for plant growth, contrasts with previous reports describing beneficial effects of Si on plant growth only under stressful conditions (Zhu and Gong Citation2014). In plants grown as an unstressed control, enhancement of light interception and net CO2 assimilation rate and improvement of water-relation parameters have been found to be the main reasons for Si-mediated growth stimulation, partly through decrease of transpiration and activation of antioxidative defence (Hajiboland Citation2012; Savvas and Ntatsi Citation2015; Hajiboland et al. Citation2017b).
Figure 1. Shoot and root dry weight (g plant−1) at three developmental stages and fruit dry weight (g fruit−1) at the full-red stage in strawberry (F. × ananassa) plants grown in the absence (–Si) or presence (+Si) of silicon (as Na2SiO4) under greenhouse conditions. Data are mean ± standard deviation (n = 4). Bars indicated by the same letter are not statistically different (t-test, P < .05).
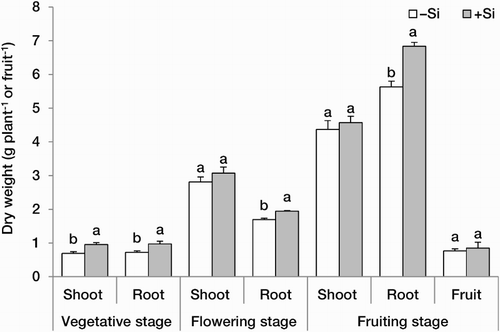
Silicon increasingly accumulated in the leaves during all three growth stages, not only in the +Si, but also in the –Si plants (). In the –Si plants, Si was primarily derived from the distilled water used for irrigation and making nutrient solutions. Leaf concentrations of Zn were higher in +Si plants in the flowering and fruiting stages, while the changes in Mn concentrations were not significant (). The higher Zn uptake in +Si plants is likely attributable to the effect of Si on Zn-transporters (ZIP family), which are similar to Fe transporters, such as iron-regulated transporter (IRT)1 and IRT2. Recently, it has been shown that IRT genes are upregulated by Si (Pavlovic et al. Citation2013). Alternatively, the effect of Si on the improvement of leaf micronutrient status may be related to enhanced membrane functionality and/or indirectly from the stimulation of root growth. Higher uptake of K, P, Fe and Zn in Si-treated plants has been observed in other plant species (Mehrabanjoubani et al. Citation2015; Hajiboland et al. Citation2017b). Nevertheless, the positive effects of Si on plant growth could not be attributed to increased concentrations of micronutrients. Even in ‒Si plants, the nutritional status exceeded the critical levels for the respective micronutrient deficiencies.
Table 1. Leaf silicon (Si), zinc (Zn) and manganese (Mn) concentrations at three developmental stages in strawberry (F. × ananassa) plants grown in the absence (–Si) or presence (+Si) of silicon (as Na2SiO4) under greenhouse conditions.
Effect of Si on the carbon and nitrogen compounds
Leaf concentration of soluble carbohydrates was lower in +Si plants; this effect was significant at flowering and fruiting stages. Leaf starch concentration in +Si plants decreased at flowering stage and increased at fruiting stage, while it remained unchanged at vegetative stage ((A)). In contrast to the leaves, the concentration of soluble carbohydrates in the roots increased upon Si treatment; however, there was no significant effect of Si on the root starch concentration ((B)). Reduction of non-structural carbohydrates in the leaves (except for starch at fruiting stage) could result from reduced rates of photosynthesis, increased utilisation for growth and/or allocation to the roots. We observed earlier that leaf photosynthesis of strawberry plants at vegetative stage increased significantly by Si treatment (Moradtalab & Hajiboland, unpublished data). Thus, reduction of leaf carbohydrates in this study by Si treatment is attributable to an increased utilisation of carbon resources for growth and/or higher allocation to the roots. The latter assumption was confirmed by a higher root carbohydrate pool in +Si plants.
Figure 2. Concentrations (mg g−1 FW) of carbohydrates in A, the leaves and B, roots at three developmental stages in strawberry (F. × ananassa) plants grown in the absence (–Si) or presence (+Si) of silicon (as Na2SiO4) under greenhouse conditions. Data are mean ± standard deviation (n = 4). Bars indicated by the same letter are not statistically different (t-test, P < .05).
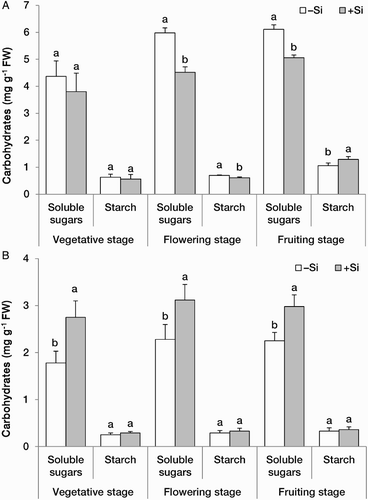
Higher starch concentration in the leaves of plants at fruiting stage might be in turn the result of an excess carbon pool that could not be consumed in the production of dry weight, likely because of cessation of overall growth rate at this stage. It has been reported that application of potassium silicate as leaf spray in strawberry plants results in increases in citric acid and malic acid levels, and decreases in fructose, glucose and sucrose contents (Wang and Galletta Citation1998). In addition, considering the significant effect of Si on the accumulation of phenolic compounds (see below), reduction of carbohydrates could be due also to an increased recruitment of carbon pools to the phenolics biosynthesis pathway.
Concentrations of free amino acids in the leaves decreased in +Si plants at vegetative and flowering stages, while the concentration of total soluble proteins increased significantly upon Si supplementation at all three developmental stages ((A)). Similar to the leaves, concentrations of free amino acids in the roots decreased while that of soluble proteins increased by Si addition ((B)). Changes in the concentration of amino acids and proteins in the opposite direction revealed that the Si-mediated increase in the protein synthesis occurred at the expense of the free amino acid pool. Higher protein synthesis may support growth and is another mechanism for the observed improvement of dry matter production in +Si strawberry plants. The mechanism for an elevated level of total soluble protein pool by Si is not known but is likely similar to that observed for selenium, i.e. through, at least partly, improved nitrogen assimilation (Hajiboland and Sadeghzadeh, Citation2014). We observed higher activity of nitrate reductase in Si-treated tobacco (Hajiboland & Cheraghvareh, unpublished data). It appears that in addition to improving the efficiency of protein synthesis, Si regulates expression of particular proteins as observed in a proteomic study in rice (Nwugo and Huerta Citation2011).
Figure 3. Concentrations (mg g−1 FW) of nitrogenous compounds in A, the leaves and B, roots at three developmental stages in strawberry (F. × ananassa) plants grown in the absence (–Si) or presence (+Si) of silicon (as Na2SiO4) under greenhouse conditions. Data are mean ± standard deviation (n = 4). Bars indicated by the same letter are not statistically different (t-test, P < .05).
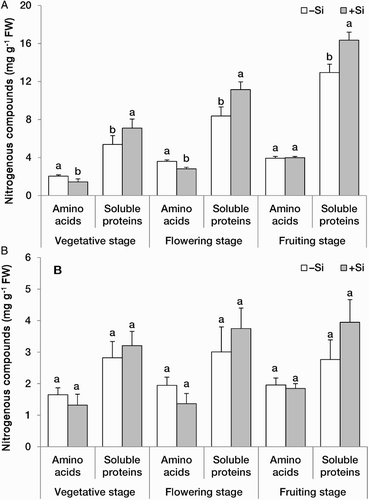
Strawberries are a perennial species, thus, higher carbohydrates accompanied by higher protein levels in the roots in this work may be of great importance for supporting growth of Si-supplemented plants in the next season. Early spring growth of perennial species is supported by the carbohydrate and nitrogen reserves accumulated in the roots from the previous growing season. The limitations of these reserves inhibit the initial spring growth and yield of perennial strawberries (Acuña-Maldonado and Pritts Citation2008). It has been reported that strawberry transplants with higher carbohydrate content in reserve organs lead to higher early and total fruit production (Cocco et al. Citation2016).
Effect of Si on the phenolics concentration and metabolism
Total soluble ((A)) and CW-bound ((B)) phenolics were higher in the leaves and roots of +Si plants. Effect of Si was more pronouncedly observed in the leaves than in the roots and for the soluble phenolics compared with CW-bound phenolics ((B)). Soluble phenolics in the leaves and roots may contribute to the antioxidative and free radical-scavenging activities and protect cells against abiotic stresses, such as high light and drought stress conditions (Winkel-Shirley Citation2002; Gill and Tuteja Citation2010; Quideau et al. Citation2011). CW-bound phenolics, by contrast, are mainly involved in the defence mechanisms against pathogens and the attack of pests (de Ascensao and Dubery Citation2003).
Figure 4. Concentrations (mg g−1 FW) of total soluble (A) and CW-bound (B) fractions of phenolic compounds in the leaves and roots of strawberry (F. × ananassa) plants at three developmental stages grown in the absence (–Si) or presence (+Si) of silicon (as Na2SiO4) under greenhouse conditions. Data are mean ± standard deviation (n = 4). Bars indicated by the same letter are not statistically different (t-test, P < .05).
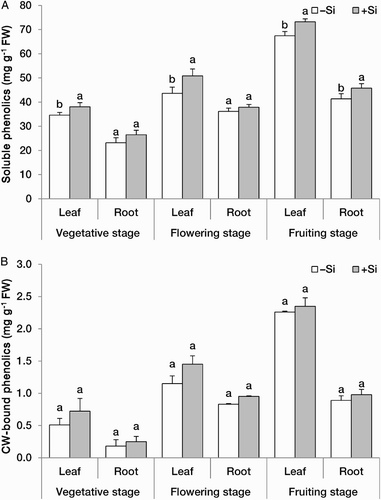
Activity of PAL was higher in +Si plants; a significant effect of Si was observed at vegetative and fruiting stages (). Activity of PPO, however, increased only at flowering and fruiting stages while it decreased significantly in vegetative plants upon Si treatment. Activity of cytosolic POD and covalently bound POD tended to be higher in the presence of Si without statistically significant difference with –Si plants. The activity of ionically bound POD, in contrast, decreased in +Si plants and was significant at vegetative and flowering stages (). Cytosolic POD is mainly involved in the scavenging of reactive oxygen species while CW-bound POD is responsible for polymerisation of monolignolyl compounds and lignin synthesis (Siegel Citation1993). Thus, our data of enzyme activities suggest that Si treatment results in upregulation of phenolics synthesis (mediated by PAL) and their further interconversions (mediated by PPO), while their utilisation in polymerising reactions for lignin synthesis was not stimulated, or rather was downregulated, by Si. From the growth-stimulating effect of Si, it was also expected that the excess phenolics that were synthesised in the presence of Si were not utilised for lignin formation in the strawberry plants of this study, because CW strengthening by lignin would hamper growth of the cells. In cucumber, another accumulating species, Si modulates the metabolism and utilisation of phenols by stimulating the formation of Si polyphenol complexes, thus reducing concentrations of phenolic compounds available to act as substrates for PPO and POD (Maksimović et al. Citation2007). In the absence of adequate activity of lignin biosynthetic enzymes in the +Si strawberry plants; however, a high concentration of phenolics found in the CW-bound fraction is likely to be a reflection of joining a considerable portion of phenolics to the CW without polymerisation. The esterification of phenols to CW materials and cross-linking of phenylpropanoid esters increases resistance to fungal enzymes and acts as a physical barrier against fungal penetration (de Ascensao and Dubery Citation2003). Thus, although CW-bound phenolic acids may not contribute in the lignin synthesis as substrate, they play still a conspicuous role in the structural protection of tissues against biotic attackers. It is likely that Si binding to the CW forms new negative sites for binding of phenolic acids. Using inductively coupled plasma mass spectrometry of the CW fractions in rice, it has recently been observed that 64% of the total Si in the CWs was bound to hemicellulose constituents; this hemicellulose-bound form of Si has net negative charges (Ma et al. Citation2015). Reduction of CW-bound POD activity by Si simultaneous with higher CW-bound phenolics may confirm again the probably lower tissue lignin content in +Si plants in favour of low molecular weight CW-bound phenolic acids. In addition to phenolic acids, Si by itself is an efficient structural protectant in the CW. Lower lignin content observed in the Si-treated plants may reflect the role of Si in the enhancing mechanical strength of CW, allowing a trade-off between lignin and Si accumulation that may be advantageous because Si is cheaper than lignin as a source of structural material for providing the structural resistance of the CWs (Hajiboland et al. Citation2017a).
Table 2. Leaf activity of PAL, PPO, and cytosolic (PODCyt), ionically bound (PODI-bound) and covalently bound (PODC-bound) fractions of POD at three developmental stages in strawberry (F. × ananassa) plants grown in the absence (–Si) or presence (+Si) of silicon (as Na2SiO4) under greenhouse conditions.
Effect of Si on the phenolics profile and fractionation
On analyses of phenolics in both soluble and CW-bound fraction of the leaves, roots and fruits, we found gallic acid, caffeic acid, epicatechin, chlorogenic acid, p-coumaric acid, quercetin, ellagic acid and kaempferol as the major phenolic components. In high-performance liquid chromatography with diode-array detection and high-performance liquid chromatography/electrospray ionization tandem mass spectrometry analyses of strawberry leaf, 32 phenolic compounds were detected including those found in our study and their close derivatives (Kårlund et al. Citation2014).
Silicon significantly modified the profile of phenolics in the leaves and roots at all three developmental stages (). Leaf concentrations of gallic acid, caffeic acid, epicatechin and chlorogenic acid were consistently higher in +Si plants at all three developmental stages. In contrast, the concentration of p-coumaric acid decreased in +Si plants that was significant at the fruiting stage for both fractions. Si-mediated increase in the leaf concentration of quercetin was observed only in the soluble fraction. Ellagic acid concentration responded differently depending on the cell fraction; it was decreased in the soluble fraction and increased in the CW-bound fraction by Si; this increase and decrease were mainly significant at vegetative and flowering stages. Similar but non-significant difference was observed for kaempferol between two fractions in response to Si ().
Table 3. Phenolics profile and concentrations in the soluble (µg mg−1 total phenol) and CW-bound (ng mg−1 total phenol) fractions of leaves and roots at three developmental stages in strawberry (F. × ananassa) plants grown in the absence (–Si) or presence (+Si) of silicon (as Na2SiO4) under greenhouse conditions.
The same trend, but to a lesser extent, was observed in the roots supplemented with Si. Gallic acid, caffeic acid, epicatechin and chlorogenic acids concentrations in both fractions showed an increase in +Si compared with –Si plants. Similar with the leaf, p-coumaric acid concentration decreased upon Si treatment in both cell fractions while in contrast with the leaf, quercetin concentration was increased in both fractions by Si except in the CW-bound fraction of flowering plants. Ellagic acid decreased in the soluble but increased in the CW-bound fractions by Si; however, the majority of these changes were statistically non-significant. The effect of Si on kaemferol concentration was not consistent and a significant reduction at the vegetative stage but an increase at the fruiting stage was observed in its concentration in the CW-bound fraction upon Si treatment ().
The biological significance of the changes in the phenolics profile mediated by Si in this study is not known. In a study on the water extract of strawberry leaf, it was found that the highest antioxidative activity belongs to the catechin followed by ellagic acid (Buricova et al. Citation2011). In aqueous solution at physiological pH, ellagic acid anion is capable of deactivating a wide variety of free radicals and is efficiently and continuously regenerated after scavenging two free radicals per cycle. This feature of ellagic acid contributes to its higher antioxidant activity at low concentrations without reduction after being metabolised through a free radical-scavenging cascade (Galano et al. Citation2014). Detailed studies on the structural features associated with free radical-scavenging activity of phenolic compounds have revealed that there are numerous criteria that are important for free radical-scavenging activity, such as the frequency of intramolecular hydrogen bonds, planarity of the molecule and the number of hydroxyl substitutes (Alov et al. Citation2015). Flavones with a single OH substitution had scavenging activities below 1.0, whereas kaempherol and quercetin which have four and five OH substitutions, respectively, had free-oxygen absorbing activities of 2.7 and 3.3, respectively (Cao et al. Citation1997). Among various kaempferol compounds, there is also a considerable difference in the oxygen radical absorbing activity depending on the number of free OH substitutions (Cao et al. Citation1997). Since different derivatives of each phenolic compound could not be identified by the HPLC method used in this study, we could not precisely verify the effectiveness of Si on the improvement of antioxidative activity of each individual compound.
The rapid synthesis of low molecular weight phenols, triggered by the exposure of plant tissues to CW-derived elicitors of pathogen origin, is a well-described phenomenon, forming part of the defence mechanism of plants (Nicholson and Hammerschmidt Citation1992). However, the effect of exogenous Si on the activation of plant chemical defence has not attracted adequate attention. The increased production of POD and phenolic compounds may be of key importance in the resistance process (Lattanzio et al. Citation2006; Amil-Ruiz et al. Citation2011) and considering that the accumulation of structural substances may increase the mechanical strength of the host CW, the induction of such defence mechanisms by Si would likely inhibit or at least restrict pathogen invasion. In banana plants, it has been observed that the phenylpropanoid pathway is potentiated by Si in the roots during infection by Fusarium (Fortunato et al. Citation2014). However, information is lacking on the probable differences in the efficiency of various sub-classes of phenolics against biotic factors. Attempts were made on the induction of phenolics accumulation by experimental application of various natural or synthetic elicitors (Kårlund et al. Citation2014). Our results suggest that in strawberry plants, application of Si for induction of defence-related phenolics machinery has an advantage over application of other compounds because of its improving effect on the growth and quality of fruits.
From a metabolic point of view, the differential change of phenolics concentration by Si could be explained by the upregulation of the pathways that ultimately produces flavonols (kaempferol and quercetin) and flavanols (epicatechin). In addition, various pathways for synthesis of phenolic acids (gallic acid, caffeic acid, chorogenic acid and ellagic acid) all seem to be upregulated by Si (Figure S1). The reduction of caumaric acid by Si treatment observed consistently in all tested plant fractions and at all developmental stages, appears to be the consequence of higher utilisation of this intermediate metabolite for synthesis of flavonols and flavanols rather than a specific downregulation of its synthesis in +Si treatments. Similarly, reduction of ellagic acid concentration in the cytosolic fraction of leaf simultaneous with an increase in its concentration in the CW-bound fraction suggests a Si-mediated change in the partitioning of this phenolic acid but not a reduction of its synthesis by Si. The underlying mechanisms for Si-mediated modification of the phenylpropanoid pathway are obscure. The changes in the activity of enzymes upon Si treatment are mainly attributed to the post-transcriptional and/or allosteric regulation of enzymes (Van Bockhaven et al. Citation2013). Silicon is increasingly being associated with modulation of primary signal transduction (Detmann et al. Citation2013; Van Bockhaven et al. Citation2013; Hajiboland et al. Citation2017a). Silicon may influence plant mechanisms through hormone signalling as was observed in the leaves challenged with pathogens (Van Bockhaven et al. Citation2013).
The most prominent effect of Si in this work was observed on the fruit quality. All fruit quality parameters were significantly increased by Si supplementation except for titratable acidity, which was slightly decreased in +Si plants (). Lower acidity has been assumed to be a quality parameter (Mitcham Citation1996). In contrast to the leaves and roots with differential change of phenolics, concentrations of all phenolic constituents in the fruit in both soluble and CW-bound fractions were higher in +Si compared with –Si plants, except for p-coumaric acid (). In addition to an increase in quality and health promoting effects through the phenolics accumulated in the fruits of +Si plants, an elevated resistance against biotic and abiotic factors during the postharvest period is also expected in the fruits of Si-treated plants. It has been observed that postharvest application of Si on avocado fruits results in increased fruit firmness, total phenolics concentration and lowers lipid peroxidation in the mesocarp (Tesfay et al. Citation2011).
Figure 5. Phenolics profile and concentrations in the soluble (A) (µg mg−1 total phenol) and CW-bound (B) (ng mg−1 total phenol) fractions in the fruit of strawberry (F. × ananassa) plants grown in the absence (–Si) or presence (+Si) of silicon (as Na2SiO4) under greenhouse conditions. Data are mean ± standard deviation (n = 4). Bars indicated by the same letter are not statistically different (t-test, P < .05).
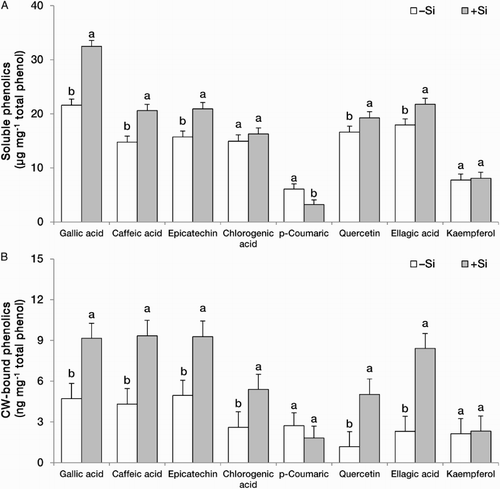
Table 4. Fruit quality parameters including concentration of soluble sugars (mg g−1 FW), soluble (mg g−1 FW) and CW-bound (mg g−1 FW) phenolics, total antioxidative activity (DPPH radical quenching, %), concentration of ascorbic acid (mg g−1 FW), titratable acidity (g citric acid g−1 FW), concentration of antocyanines (mg cyanidin-3-glucoside g−1 FW) and firmness (%) in strawberry (F. × ananassa) plants grown in the absence (–Si) or presence (+Si) of silicon (as Na2SiO4) under greenhouse conditions.
Figure S1. An illustration of phenylpropanoid pathway and effect of Si on the levels of phenolic compounds found in the strawberry plants.
Download TIFF Image (331 KB)Disclosure statement
No potential conflict of interest was reported by the authors.
Additional information
Funding
References
- Acuña-Maldonado LE, Pritts MP. 2008. Carbon and nitrogen reserves in perennial strawberry affect plant growth and yield. J Am Soc Hortic Sci. 133:735–742.
- Alov P, Tsakovska I, Pajeva I. 2015. Computational studies of free radical-scavenging properties of phenolic compounds. Curr Top Med Chem. 15:85–104. doi: 10.2174/1568026615666141209143702
- Amil-Ruiz F, Blanco-Portales R, Muñoz-Blanco J, Caballero JL. 2011. The strawberry plant defense mechanism: a molecular review. Plant Cell Physiol. 52:1873–1903. doi: 10.1093/pcp/pcr136
- Buricova L, Andjelkovic M, Cermakova A, Reblova Z, Jurcek O, Kolehmainen E, Verhe R, Kvasnicka F. 2011. Antioxidant capacities and antioxidants of strawberry, blackberry and raspberry leaves. Czech J Food Sci. 29:181–189. doi: 10.17221/300/2010-CJFS
- Cao G, Sofic E, Prior RL. 1997. Antioxidant and prooxidant behavior of flavonoids: structure-activity relationships. Free Radical Biol Med. 22:749–760. doi: 10.1016/S0891-5849(96)00351-6
- Cocco C, Gonçalves MA, Reisser Junior C, Marafon AC, Antunes LEC. 2016. Carbohydrate content and development of strawberry transplants from Rio Grande Do Sul and imported. Rev Bras Frutic. 38:156. doi: 10.1590/0100-29452016581
- de Ascensao AR, Dubery IA. 2003. Soluble and wall-bound phenolics and phenolic polymers in Musa acuminata roots exposed to elicitors from Fusarium oxysporum f. sp. cubense. Phytochem. 63:679–686. doi: 10.1016/S0031-9422(03)00286-3
- Detmann KC, Araújo WL, Martins SCV, Fernie AR, Fábio M, DaMatta FM. 2013. Metabolic alterations triggered by silicon nutrition. Is there a signaling role for silicon? Plant Signal Behav. 8:71–74. doi: 10.4161/psb.22523
- Elliott CL, Snyder GH. 1991. Autoclave-induced digestion for the colorimetric determination of silicon in rice straw. J Agr Food Chem. 39:1118–1119. doi: 10.1021/jf00006a024
- Fortunato AA, da Silva WL, Rodrigues FÁ. 2014. Phenylpropanoid pathway is potentiated by silicon in the roots of banana plants during the infection process of Fusarium oxysporum f. sp. cubense. Phytopathol. 104:597–603. doi: 10.1094/PHYTO-07-13-0203-R
- Galano A, Francisco Marquez M, Pérez-González A. 2014. Ellagic acid: an unusually versatile protector against oxidative stress. Chem Res Toxicol. 27:904–918. doi: 10.1021/tx500065y
- Giampieri F, Alvarez-Suarez JM, Battino M. 2014. Strawberry and human health: effects beyond antioxidant activity. J Agric Food Chem. 62:3867–3876. doi: 10.1021/jf405455n
- Gill SS, Tuteja N. 2010. Reactive oxygen species and antioxidant machinery in abiotic stress tolerance in crop plants. Plant Physiol Biochem. 48:909–930. doi: 10.1016/j.plaphy.2010.08.016
- Hajiboland R. 2012. Effect of micronutrient deficiencies on plants stress responses. In: Ahmad P, Prasad MNV, editors. Abiotic stress responses in plants. New York, NY: Springer; p. 283–329.
- Hajiboland R, Bahrami-Rad S, Bastani S. 2013. Phenolics metabolism in boron-deficient tea [Camellia sinensis (L.) O. Kuntze] plants. Acta Biol Hung. 64:196–206. doi: 10.1556/ABiol.64.2013.2.6
- Hajiboland R, Bahrami-Rad S, Poschenrieder C. 2017a. Silicon modifies both a local response and a systemic response to mechanical stress in tobacco leaves. Biol Plant. 61:187–191. doi: 10.1007/s10535-016-0633-3
- Hajiboland R, Cheraghvareh L, Poschenrieder C. 2017b. Improvement of drought tolerance in tobacco (Nicotiana rustica L.) plants by silicon. J Plant Nutr. doi:10.1080/01904167.2017.1310887.
- Hajiboland R, Sadeghzade N. 2014. Effect of selenium on CO2 and NO3− assimilation under low and adequate nitrogen supply in wheat (Triticum aestivum L.). Photosynthetica. 52:501–510. doi: 10.1007/s11099-014-0058-1
- Helrich K. 1990. Official methods of analysis, 15th ed. Arlington, VA, USA: Association of Official Analytical Chemists (AOAC).
- Kårlund A, Salminen JP, Koskinen P, Ahern JR, Karonen M, Tiilikkala K, Karjalainen RO. 2014. Polyphenols in strawberry (Fragaria× ananassa) leaves induced by plant activators. J Agric Food Chem. 62:4592–4600. doi: 10.1021/jf405589f
- Klopotek Y, Otto K, Böhm V. 2005. Processing strawberries to different products alters contents of vitamin C, total phenolics, total anthocyanins, and antioxidant capacity. J Agric Food Chem. 53:5640–5646. doi: 10.1021/jf047947v
- Lattanzio V, Lattanzio VM, Cardinali A. 2006. Role of phenolics in the resistance mechanisms of plants against fungal pathogens and insects. In: Imperato F, editor. Phytochemistry: advances in research. Kerala: Research Signpost; p. 23–67.
- Lawlor DW. 2002. Carbon and nitrogen assimilation in relation to yield: mechanisms are the key to understanding production systems. J Exp Bot. 53:773–787. doi: 10.1093/jxb/53.370.773
- Liu JJ, Lin SH, Xu PL, Wang XJ, Bai JG. 2009. Effects of exogenous silicon on the activities of antioxidant enzymes and lipid peroxidation in chilling-stressed cucumber leaves. Agr Sci China. 8:1075–1086. doi: 10.1016/S1671-2927(08)60315-6
- Ma J, Cai H, He C, Zhang W, Wang L. 2015. A hemicellulose-bound form of silicon inhibits cadmium ion uptake in rice (Oryza sativa) cells. New Phytol. 206:1063–1074. doi: 10.1111/nph.13276
- Malik JA, Kumar S, Thakur P, Sharma S, Kaur N, Kaur R, Pathania D, Bhandhari K, Kaushal N, Singh K, et al. 2011. Promotion of growth in mungbean (Phaseolus aureus Roxb.) by selenium is associated with stimulation of carbohydrate metabolism. Biol Trace Elem Res. 143:530–539.
- Maksimović DJ, Bogdanović J, Maksimović V, Nikolic M. 2007. Silicon modulates the metabolism and utilization of phenolic compounds in cucumber (Cucumis sativus L.) grown at excess manganese. J Plant Nutr Soil Sci. 170:739–744. doi: 10.1002/jpln.200700101
- Mehrabanjoubani P, Abdolzadeh A, Sadeghipour HR, Aghdasi M. 2015. Silicon affects transcellular and apoplastic uptake of some nutrients in plants. Pedosphere. 25:192–201. doi: 10.1016/S1002-0160(15)60004-2
- Mitcham B. 1996. Quality assurance for strawberries: a case study. Perishables Handling Newsletter. 85:6–9.
- Miyake Y, Takahashi E. 1986. Effect of silicon on the growth and fruit production of strawberry plants in a solution culture. Soil Sci Plant Nutr. 32:321–326. doi: 10.1080/00380768.1986.10557510
- Nicholson RL, Hammerschmidt R. 1992. Phenolic compounds and their role in disease resistance. Annu Rev Phytopathol. 30:369–389. doi: 10.1146/annurev.py.30.090192.002101
- Nour V, Trandafir I, Cosmulescu S. 2013. HPLC determination of phenolic acids, flavonoids and juglone in walnut leaves. J Chromatogr Sci. 51:883–890. doi: 10.1093/chromsci/bms180
- Nunes-Nesi A, Fernie AR, Stitt M. 2010. Metabolic and signaling aspects underpinning the regulation of plant carbon nitrogen interactions. Mol Plant. 3:973–996. doi: 10.1093/mp/ssq049
- Nwugo CC, Huerta AJ. 2011. The effect of silicon on the leaf proteome of rice (Oryza sativa L.) plants under cadmium-stress. J Proteome Res. 10:518–528. doi: 10.1021/pr100716h
- Panico AM, Garufi F, Nitto S, Di Mauro R, Longhitano RC, Magrì G, Catalfo A, Serrentino ME, De Guidi G. 2009. Antioxidant activity and phenolic content of strawberry genotypes from Fragaria × ananassa. Pharmaceutical Biol. 47:203–208. doi: 10.1080/13880200802462337
- Pavlovic J, Samardzic J, Maksimović V, Timotijevic G, Stevic N, Laursen KH, Hansen TH, Husted S, Schjoerring JK, Liang Y, et al. 2013. Silicon alleviates iron deficiency in cucumber by promoting mobilization of iron in the root apoplast. New Phytol. 198:1096–1107. doi: 10.1111/nph.12213
- Quideau S, Deffieux D, Douat-Casassus C, Pouysegu L. 2011. Plant polyphenols: chemical properties, biological activities, and synthesis. Angew Chem Int Ed. 50:586–621. doi: 10.1002/anie.201000044
- Savvas D, Ntatsi G. 2015. Biostimulant activity of silicon in horticulture. Sci Hortic. 196:66–81. doi: 10.1016/j.scienta.2015.09.010
- Schaller J, Brackhage C, Dudel EG. 2012. Silicon availability changes structural carbon ratio and phenol content of grasses. Environ Exp Bot. 77:283–287. doi: 10.1016/j.envexpbot.2011.12.009
- Siegel BZ. 1993. Plant peroxidases–an organismic perspective. Plant Growth Regul. 12:303–312. doi: 10.1007/BF00027212
- Swain T, Hillis EE. 1959. The phenolic constituents of Prunus domestica I. The quantitative analysis of phenolic constituents. J Sci Food Agric. 10:63–68. doi: 10.1002/jsfa.2740100110
- Tesfay SZ, Bertling I, Bower JP. 2011. Effects of postharvest potassium silicate application on phenolics and other anti-oxidant systems aligned to avocado fruit quality. Postharvest Biol Technol. 60:92–99. doi: 10.1016/j.postharvbio.2010.12.011
- Van Bockhaven J, De Vleesschauwer D, Höfte M. 2013. Towards establishing broad-spectrum disease resistance in plants: silicon leads the way. J Exp Bot. 64:1281–1293. doi: 10.1093/jxb/ers329
- Wang SY, Galletta GJ. 1998. Foliar application of potassium silicate induces metabolic changes in strawberry plants. J Plant Nutr. 21:157–167. doi: 10.1080/01904169809365390
- Weaver LM, Herrmann KM. 1997. Dynamics of the shikimate pathway in plants. Trends Plant Sci. 2:346–351. doi: 10.1016/S1360-1385(97)84622-5
- Winkel-Shirley B. 2002. Biosynthesis of flavonoids and effects of stress. Curr Opin Plant Biol. 5:218–223. doi: 10.1016/S1369-5266(02)00256-X
- Yemm EW, Cocking EC, Ricketts RE. 1955. The determination of amino acids with ninhydrin. Analyst. 80:209–213. doi: 10.1039/an9558000209
- Yemm EW, Willis AJ. 1954. The estimation of carbohydrates extracts by anthrone. Biochem J. 57:508–514. doi: 10.1042/bj0570508
- Zhu Y, Gong H. 2014. Beneficial effects of silicon on salt and drought tolerance in plants. Agron Sustain Dev. 34:455–472. doi: 10.1007/s13593-013-0194-1