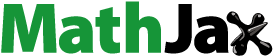
Abstract
This research has investigated fungal transformations of rock phosphate (RP) by geoactive fungi, with particular emphasis on Aspergillus niger. Direct hyphal interaction with RP particles induced morphological and mineralogical changes, as revealed by scanning electron microscopy (SEM) and X-ray diffraction (XRD) analysis. The formation of the oxalate mineral calcium oxalate monohydrate (whewellite, CaC2O4·H2O) on RP surfaces showed that mycogenic oxalic acid was driving the chemical dissolution of apatite, with consequent phosphate release and secondary mineral formation. This was supported by abiotic testing of common fungal excreted organic acids which confirmed that oxalic acid was the only effective RP transforming agent and therefore responsible for the morphological and mineralogical changes observed in RP when exposed to fungal colonization. Cryogenic SEM provided evidence of fungal penetration and tunneling through RP particles demonstrating that physical interactions are also important for RP bioweathering, as well as biochemical mechanisms. These findings emphasize the important role of fungi in P cycling, with active participation in the transformation of mineral phosphates through physicochemical mechanisms and secondary oxalate biomineral formation.
Introduction
Phosphates are most commonly found in sparingly-soluble mineral forms within terrestrial and marine environments and the breakdown of these minerals is required to provide bioavailable phosphate to natural ecosystems, particularly by organisms colonizing new environments such as volcanic flows, glacial deposits, and newly eroded or deposited land surfaces (Gadd Citation2007, Citation2017a; Pollard Citation2018). However, in many agricultural systems phosphorus (P) is a limiting nutritional factor for crop yield, so is frequently added to soil to compensate for inadequate levels of plant-available phosphate (Cordell et al. Citation2009; Roy et al. Citation2016). Such addition to preexisting total P levels in soil can have environmental repercussions, including effects on soil biodiversity, plant adaptation, and freshwater eutrophication (Cordell and Neset Citation2014; Rockström et al. Citation2009; Withers et al. Citation2015). Most of the P added to soil as fertilizer is derived from rock phosphate (RP), a natural multi-mineral substrate formed under high pressure over an extended geological time period (Oelkers and Valsami-Jones Citation2008). Much of this is industrially processed into fertilizer before soil application, but in developing countries or organic farming systems where fertilizer use is not possible or prohibited, then rock phosphates are often added as the primary source of additional P (Rajan et al. Citation1996; Zapata and Roy Citation2004).
Many microorganisms and plants are capable of bioweathering RP, and other insoluble phosphate-containing minerals, to liberate free phosphate (Arcand and Schneider Citation2006; Gadd Citation2007, Citation2017a; Kang et al. Citation2019, Citation2020; Richardson and Simpson Citation2011; Sullivan and Gadd Citation2019; Suyamud et al. Citation2020), and fungi are particularly important in this regard. Free-living and symbiotic fungi are intimately involved in influencing phosphate availability in the rhizosphere as well as playing a role in soil bioremediation (Adeyemi and Gadd Citation2005; Fomina et al. Citation2007; Hagerberg et al. Citation2003; Sullivan and Gadd Citation2019; Whitelaw Citation1999). Many species of fungi can release soluble phosphate and metal species from minerals, predominantly mediated by the excretion of organic acids and protons (Gadd Citation1999, Citation2007; Gadd et al. Citation2014; Jones Citation1998; Jones et al. Citation2003; Mendes et al. Citation2014; Suyamud et al. Citation2020; Whitelaw Citation1999). This can result in the formation of secondary biogenic minerals, for example, calcium oxalate (Burford et al. Citation2003; Fomina et al. Citation2010; Gadd Citation2017b; Gadd et al. Citation2014). Magnesium oxalate (glushinskite) resulted during solubilization and phosphate release from the insoluble phosphate mineral struvite (magnesium ammonium phosphate – MgNH4PO4.6H2O) by Aspergillus niger (Suyamud et al. Citation2020), La and Ce oxalates resulted from the transformation of monazite sand, a naturally occurring lanthanide (Ln) phosphate mineral [Lnx(PO4)y] (Kang et al. Citation2020), while lead oxalate was formed through fungal bioweathering of pyromorphite (Pb5(PO4)3Cl), a lead-containing phosphate mineral (Ceci et al. Citation2015; Fomina et al. Citation2004; Sayer et al. Citation1999). Other mechanisms of phosphate solubilization include acidification of the microenvironment by respiration-induced carbonic acid production (Burgstaller and Schinner Citation1993) and/or the production of other metal-complexing metabolites (Hoffland et al. Citation2004; Kucey et al. Citation1989).
Phosphate-solubilizing fungi are therefore able to promote the development of environmentally desirable metal-phosphate species and metal complexes which can reduce metal toxicity in polluted soils and improve plant yields by increasing P availability (Burgstaller and Schinner Citation1993; Fomina et al. Citation2004; Gadd Citation2017b; Goldstein et al. Citation1993; Mendes et al. Citation2015, Citation2017; Sullivan and Gadd Citation2019; Vassilev et al. Citation2013; Whitelaw Citation1999). Characterization of fungal interactions with a variety of P-containing minerals/metal compounds has been carried out (Fomina et al. Citation2004, Citation2005; Gadd Citation2017b; Jacobs et al. Citation2002; Liang and Gadd Citation2017; Mendes et al. Citation2014; Suyamud et al. Citation2020). Many studies have investigated the geochemical importance of fungi to nutrient mobilization, element cycling, and plant growth through bioweathering, including ectomycorrhizas (Hoffland et al. Citation2004; Landeweert et al. Citation2001), arbuscular mycorrhizas (Duponnois et al. Citation2005; Gosling et al. Citation2013), and rock-inhabiting microbial communities (Gleeson et al. Citation2010; Gorbushina Citation2007). Conversely, free-living saprotrophic fungi have received less attention concerning P mineral breakdown, despite the ability of many species to produce geoactive metabolites, such as citric and oxalic acid (Gadd Citation1999, Citation2017a; Gadd et al. Citation2014; Suyamud et al. Citation2020). Furthermore, studies on fungal RP solubilization have been predominantly conducted under liquid fermentation conditions, where phosphate is released from RP as a result of medium acidification by the tested fungi (Arcand and Schneider Citation2006; Illmer et al. Citation1995; Illmer and Schinner Citation1995; Mendes et al. Citation2014; Schneider et al. Citation2010; Vassilev et al. Citation2014). Another proposed strategy to take advantage of phosphate-solubilizing fungi is their application as soil inoculants (Alori et al. Citation2017; Sammauria et al. Citation2020; Vassilev et al. Citation2017; Vassilev and Mendes Citation2018). However, soil conditions are very different from liquid cultures, especially regarding soil pH buffering capacity (Nelson and Su Citation2010), and, therefore, fungal RP solubilization in the soil would require additional mechanisms. Although some solid-state fermentation studies on fungal RP solubilization have been carried out (Mendes et al. Citation2013, Citation2015; Vassileva et al. Citation2010; Vassilev and Mendes Citation2018), direct interactions between fungal hyphae and RP particles or the physical and biochemical bioweathering mechanisms involved have not been investigated. Therefore, the aim of this research was to investigate both chemical and physical bioweathering of RP, using a range of free-living saprotrophic fungi with known geoactive properties, to clarify the mechanisms involved and their significance in mediating phosphate release and secondary mineral formation.
Materials and methods
Organisms and media
Aspergillus niger ATCC 1015, Serpula himantioides, and Trametes versicolor, from the Geomicrobiology Group culture collection, were maintained on malt extract agar (MEA, lab M, Bury, UK) at 25 °C in the dark. All test fungi are known to produce organic acids and secondary minerals and all have the potential to solubilize various minerals or insoluble metal compounds (Adeyemi and Gadd Citation2005; Ferrier et al. Citation2019; Fomina and Gadd Citation2008; Gadd Citation2016; Gharieb et al. Citation1998).
Rock phosphate
The rock phosphate used (Gardener’s Direct, Hertford, UK) contained 13% P with an aqueous suspension having a pH of 6.6. The RP was milled in a 25 mm ceramic cylinder with 20 mm diameter ceramic balls at a frequency of 30 beats/s for 1 min in an MM2000 ball mill (Glen Creston Ltd., London, UK) and sieved to obtain particles of size 151–355 µm. Individual RP particles with visible morphological differences under light microscopy were analyzed using energy dispersive X-ray analysis (EDXA) to semi-quantitatively study the variation in P content. This analysis showed that the RP contained an average of ∼6% P and a maximum percentage of ∼11% P in a single particle (data not shown).
Fungal interaction with RP particles
MEA was prepared according to manufacturers’ instructions (Lab M, Bury, UK) and amended with RP at 0.5% (w/v), which corresponds to the dose of the P source usually adopted in microbial phosphate solubilization studies (Nautiyal Citation1999). The RP and MEA were sterilized separately by autoclaving at 121 °C for 15 min, before mixing once MEA had cooled to approximately 50 °C. Control plates were not inoculated while test plates were inoculated with 4 mm diameter plugs of fungal mycelium cut from the edge of a 7-day old actively growing colony using a sterile cork-borer. All plates were incubated at 25 °C in the dark. Colony diameters on control and test plates were measured by taking the means of the shortest and longest diameters of the colony at specific time periods, usually twice daily. Changes in colony diameter with time were used to calculate the average growth rate. To examine direct interactions between A. niger and RP particles, MEA plates were set up with RP particles (151–355 µm) placed on the agar surface. Plates were incubated for 3 days at 25 °C in the dark in the presence or absence of A. niger. Full plate images were taken using a Sony Cybershot DSC-H20 from a 10 cm distance. All experiments were carried out in triplicate and data subjected to analysis of variance (p < 0.05) using SigmaPlot 12.5. Normality and homoscedasticity of data were checked using Shapiro–Wilk and Brown–Forsythe tests, respectively.
Abiotic phosphate solubilization
To determine the significance of organic acids in mineral transformation under solid media conditions, abiotic experiments were carried out in 9-cm diameter Petri dishes containing MEA amended with 0.5% (w/v) RP. Plates containing 0.5% (w/v) of β-tricalcium phosphate (Ca3(PO4)2, >98%, Sigma-Aldrich, Darmstadt, Germany) were used as a positive control since Ca3(PO4)2 is an effective test for phosphate solubilization (Nautiyal Citation1999). Using a sterile cork borer, 4 mm diameter cylinders of agar were cut and removed from the agar, thereby creating a well. 20 µl aliquots of Milli-Q water (control) or a 0.5 mol/l solution of either citric, oxalic, or gluconic acid (all purchased from Sigma-Aldrich, Darmstadt, Germany) were added to the wells. These organic acids are significant metabolites of A. niger (Gadd Citation1999) and have previously shown to be involved in phosphate solubilization (Mendes et al. Citation2014). Plates were incubated overnight at 25 °C prior to measuring the solubilization halo diameters around the wells. Each plate had 3 wells, with triplicate plates being measured. Data were subjected to analysis of variance (p < 0.05).
Extraction of RP particles from solid media
RP particles embedded in MEA medium were extracted after incubation by melting the agar in Milli-Q water in a crystallizing dish. Samples were gently heated to around 80 °C to melt the agar and the liquid containing dissolved medium and mycelium were then siphoned off and replaced with fresh Milli-Q water. This procedure was repeated three times for each sample. Finally, extracted minerals and secondary myogenic minerals were transferred into Eppendorf tubes, centrifuged at 1150 g for 1 min (Centrifuge 5242, Eppendorf, Hamburg, Germany), and air-dried in a desiccator at ambient temperature for ≥48 h before analysis.
Scanning electron microscopy (SEM)
For SEM, air-dried RP samples were mounted on adhesive carbon tape on standard aluminum electron microscopy stubs. For energy dispersive X-ray analysis (EDXA), samples remained uncoated and were viewed and analyzed in the scanning electron microscope (XL30, Philips, Eindhoven, The Netherlands) using the Phoenix microanalysis system, operating at an accelerating voltage of 20.0 kV. To obtain SEM images, samples were coated with 5 nm gold and palladium combination (Au/Pd) using a rotating sputter device (208HR, Cressington Scientific Instruments Ltd, Watford, UK). Samples were then viewed using an accelerating voltage of 15.0 kV.
Cryogenic SEM was used to examine direct interactions between A. niger and RP particles. MEA medium blocks (∼6 × 4 mm) containing single RP particles (colonized by the fungus or not) were cut using a sterile scalpel and mounted on electron microscopy gold stubs. Samples were frozen in liquid nitrogen slush within the freezing chamber of a cryopreparation system (Alto 2500, Oxford instruments, Abingdon, UK). Samples were then transferred under vacuum to the cryopreparation stage attached to the electron microscope and fractured to reveal the internal structure. The vacuum chamber was warmed to −95 °C and held at this temperature for 5 min to remove surface water from the samples, and after sublimation, the chamber was re-cooled to −115 °C before coating with 30 nm Au/Pd inside the microscope. The electron beam voltage was 15 kV.
X-ray diffraction (XRD) and X-ray powder diffraction (XRPD)
For X-ray diffraction (XRD) analysis, mineral samples were individually mounted onto a steel sample holder with an Al203 fixative, and an X-ray beam was fired at the sample using an X-ray diffractometer (Hilton-Brooks, Cheshire, UK) with a monochromatic Cu-Kα radiation source and curved graphite, single-crystal chronometer (30 mA, 40 kV). Diffraction intensities were taken over the range of 3–60° 2θ, at a scan rate of 1°/min in 0.1° increments. For X-ray powder diffraction (XRPD), diffraction patterns were scanned and recorded from 3 to 60° 2θ using Ni-filtered Co-Kα radiation, scanning from 3–60° 2θ counting for 300 seconds per step on an X’Pert Pro Multipurpose Diffractometer (PANalytical, Cambridge, UK) using an X’Celerator detector (PANalytical, Cambridge, UK). Phases were identified with reference to patterns from the International Center for Diffraction Data (ICDD) Powder Diffraction File (PDF) database and the Inorganic Crystal Structure Database (ICSD).
Results
Interactions between fungi and RP particles
RP addition had no effect on fungal growth and average growth rates on media containing RP were 12, 11, and 17 mm/day for A. niger, S. himantioides and T. versicolor, respectively (). Under all treatments, RP particles incubated with test fungi became gradually surrounded by a white, cloudy precipitate (). Most precipitation was observed closest to the site of fungal inoculation. Rock phosphate particles from within these cloudy zones of precipitation were extracted and examined using SEM () and XRPD (). Scanning electron microscopy of the samples revealed that the surface structure of the RP was altered by each of the test fungi in a distinct manner ().
Figure 1. Fungal interaction with rock phosphate (RP) particles on MEA plates. Images show RP incubated with (a) no fungal inoculant, (b) Aspergillus niger, (c) Trametes versicolor, and (d) Serpula himantioides for 7 days at 25 °C in the dark, with examples of mineral precipitation or dissolution circled. Scale bars: 1 mm. Images shown are typical of several images.
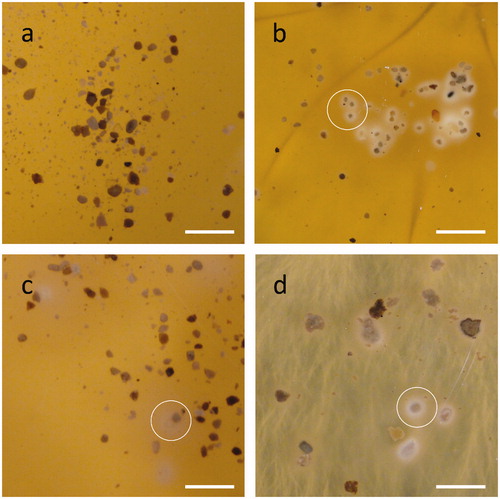
Figure 2. Alterations of rock phosphate (RP) surface structure caused by fungi. SEM images of RP incubated with (a, b) no fungi, (c, d) Aspergillus niger, (e, f) Trametes versicolor, (g, h) Serpula himantioides on MEA until fungal colonies had reached the edge of the Petri dishes. Higher magnification images in the right panel all relate to the respective panel on the left. Scale bars: (a) 100 µm, (b, d, h) 20 µm, (c, g) 200 µm, (e) 50 µm, (f) 5 µm. Images shown are typical of several images.
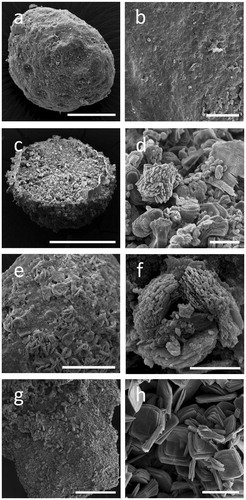
Figure 3. X-ray diffraction patterns of (a) untreated rock phosphate (RP) and RP incubated with (b) Aspergillus niger or with (c) oxalic, (d) gluconic or (e) citric acid at 0.5 mol/l. Standard patterns of (f) whewellite (ICSD 434201) and (g) fluorapatite (ICSD 9444) are also shown. All replicate samples from within treatments were pooled, and a subsample analyzed. Note the formation of whewellite when RP was incubated with A. niger and oxalic acid. Typical patterns are shown from one of several analyses.
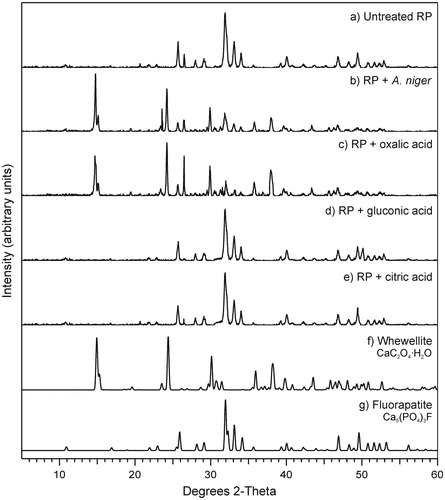
Table 1. Growth rates (mm/day ± standard error of mean) of test fungi on MEA plates with (+) or without (−) rock phosphate at 0.5% (w/v).
XRPD showed that control RP, not exposed to fungal metabolites, consisted mainly of apatite () and traces of quartz (not shown). Rock phosphate incubated with test fungi revealed the additional presence of whewellite (calcium oxalate monohydrate, CaC2O4·H2O). Overall, the intensity of peaks relating to apatite decreased with fungal incubation indicating the progressive conversion of apatite into whewellite. Since the XRPD patterns for all test fungi were similar, only data for A. niger are shown (). It is worth mentioning that there were no differences observed in RP particles that had or had not been subjected to the extraction method from solid media, meaning that any changes in mineral morphology were due to the test fungi and the metabolites they produce.
Role of organic acids in transformations of P-containing minerals
The formation of whewellite on the surface of RP particles provided strong evidence of the role of oxalic acid in the release of phosphate. To determine the significance of individual organic acids on mineral solubilization, abiotic tests with organic acids commonly associated with fungal P-mineral solubilization were conducted in agar plates containing 0.5% (w/v) Ca3(PO4)2 or RP. shows the effect of organic acids on Ca3(PO4)2 solubilization, with sterile Milli-Q water serving as a control. The halo zone surrounding the wells filled with oxalic acid was the most pronounced (∼9 mm diameter), followed by citric acid (∼5 mm diameter), with no halo zones surrounding the gluconic acid and control wells. In the plates containing RP, oxalic acid again produced the most distinct changes in mineral/plate appearance () and was the only organic acid that resulted in any noticeable changes. The light-colored precipitates observed in these experiments resembled those seen around RP particles incubated with various fungi in earlier experiments (), suggesting that oxalic acid is mainly responsible for the reaction seen.
Figure 4. Solubilization haloes from (a–d) Ca3(PO4)2 or (e–h) rock phosphate by addition of individual organic acids into wells bored in MEA plates amended with 0.5% P mineral. Agar wells were filled with 20 µl (a, e) ultrapure water, (b, f) citric, (c, g) oxalic or (d, h) gluconic acid. All organic acid concentrations were 0.5 mol/l. Images shown are typical examples from several determinations.
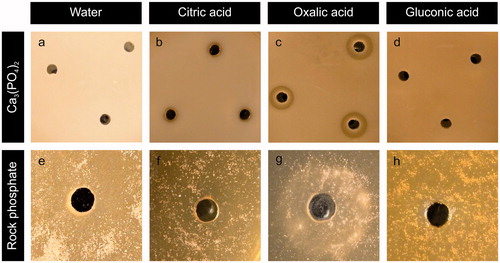
When RP particles were extracted from the agar surrounding the wells and examined using SEM, it was clear that the white precipitates observed when RP was exposed to oxalic acid corresponded to changes in surface morphology and structure of the RP particles (). Citric and gluconic acid created no detectable changes in the surface appearance of RP.
Figure 5. Alterations of RP surface structure when treated with organic acids in abiotic agar plates. SEM images showing RP exposed to (a, b) ultrapure water, (c, d) citric, (e, f) oxalic, or (g, h) gluconic acid. Scale bars: (a) 50 µm, (c, e, g) 100 µm, (b) 10 µm, (d, h) 25 µm, (f) 5 µm. Images shown are typical of several images.
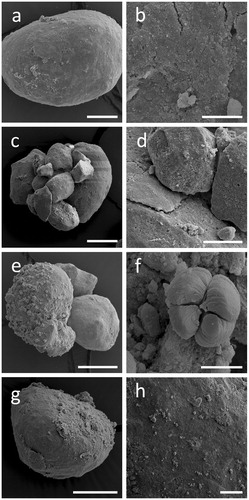
Rock phosphate particles extracted from these plates were analyzed for mineral composition using XRPD (). Significant mineralogical changes were only detected with oxalic acid, which again showed the presence of whewellite, also found when RP was incubated with test fungi ().
Physical mechanisms of RP bioweathering by A. niger
The effect of fungal metabolites, particularly oxalic acid, has therefore been demonstrated to play a key role in biochemical alteration of RP. However, the physical effects of fungal colonization cannot be overlooked. Therefore, RP particles were incubated in the presence or absence of A. niger, with no barrier between the particles and the fungus, thereby allowing direct colonization of the RP. Aspergillus niger was chosen for this aspect because it produced pronounced chemical changes in the RP particles and has a well-known ability to solubilize mineral phosphates (Mendes et al. Citation2014; Whitelaw Citation1999).
Extensive RP colonization by A. niger was clearly visible on the outer and internal surfaces of the particles (, respectively). Furthermore, hyphae were observed to show physical penetration and burrowing through the particles (), and possibly thigmotropism, that is, responding to cracks and fissures within the RP particle structure ().
Figure 6. Colonization of RP particles by Aspergillus niger. Left (a, c, e, g) and right (b, d, f, h) panels show cryogenic scanning electron micrographs of non-inoculated and inoculated RP particles, respectively. Note the complex aggregate structure (a, c) and the porosity (e, g) of RP particles. The images on the right show a RP particle with the middle section removed, allowing the interior of the particle to be studied. Note the ability of A. niger to effectively colonize RP particles (b), as well as thigmotropically (T) responding to cracks and fissures within the structure (d, f), physically burrowing (B) through RP particles (h) and depositing minerals or forming mineral nucleation sites on their hyphal surfaces (h). Scale bars: (a) 200 µm, (b) 1 mm, (c) 500 µm, (d–f) 50 µm, (g) 10 µm, and (h) 20 µm.
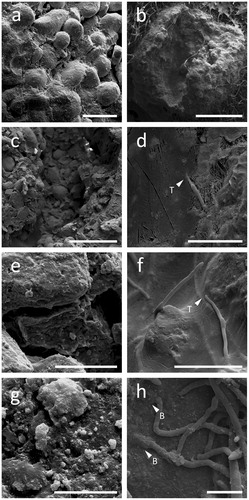
Discussion
This research has demonstrated direct fungal interactions with RP particles, highlighting the chemical and physical transformations mediated by the fungus during RP bioweathering. Our data have demonstrated that oxalic acid had a key role in the chemical transformation of apatite in RP, resulting in the formation of whewellite as a secondary biomineral and, hence, releasing soluble phosphate. Moreover, we present evidence of physical mechanisms of fungal bioweathering, including penetration, burrowing, and tunneling through the RP particles.
Whewellite was present in a variety of crystalline forms after incubation of RP with A. niger, S. himantioides, and T. versicolor. It has been found that RP solubilization using oxalic acid in an aqueous medium produces calcium oxalate minerals, with a predominance of whewellite over weddellite (Mendes et al., unpublished data). Whewellite (CaC2O4·H2O) is hydrated calcium oxalate, and as a monohydrate, it is more stable than its dihydrate form (weddellite) and one of the most common forms of oxalate encountered in the environment (Gadd Citation1999; Gadd et al. Citation2014). In terrestrial environments, calcium oxalate crystals are commonly found associated with fungi, and many species are capable of their formation due to secretion of oxalic acid: both weddellite and whewellite have been recorded (Fomina et al. Citation2010; Gadd Citation1999, Citation2017b; Gadd et al. Citation2014; Pylro et al. Citation2013). In our experiments, the source of the calcium was primarily from the apatite component of the RP, confirming that the whewellite product results from the interaction between the fungus and the apatite, following the equation:
(1)
(1)
The phosphate from the apatite is released into the external medium and can either remain free in the medium, be taken up by the fungi or other organisms (bacteria, plants) in the vicinity, or reprecipitate as a secondary phosphate mineral. Thus, it is clear that the excretion of oxalic acid was significant in affecting the solubilization of RP mineral components. This is also supported by previous research where oxalic acid was found to be the most effective organic acid for solubilizing RP in aqueous media (Kpomblekou and Tabatabai Citation1994, Citation2003; Mendes et al. 2014, 2020).
The role of oxalic acid in RP transformations after fungal inoculation was also confirmed in abiotic tests with the most common organic acids associated with fungal phosphate solubilization, namely oxalic, citric, and gluconic acid (Arcand and Schneider Citation2006; Mendes et al. Citation2014; Richardson et al. Citation2011; Schneider et al. Citation2010; Silva et al. Citation2014). Oxalic acid was the most effective organic acid at solubilizing Ca3(PO4)2 and RP, producing the widest halo zone on Ca3(PO4)2-containing media and the greatest extent of noticeable precipitation on the RP which was similar to that observed on RP when directly exposed to fungal exudates. Images of the RP particles taken from the area surrounding the wells revealed similar morphologies between RP particles treated abiotically with oxalic acid or with test fungi. In fact, oxalic acid was found to be the only organic acid that caused a change in the surface morphology and mineral composition of RP, resulting in whewellite production. Citric acid showed a much lower potential than oxalic acid for solubilizing the P sources. This is corroborated by other published works that report a lower RP solubilization efficiency of citric acid because oxalate can form a more stable complex with calcium (Kpomblekou and Tabatabai Citation1994, Citation2003; Mendes et al., Citation2020). There were no obvious effects of gluconic acid, which implied that gluconate was not involved in direct solubilization of RP under these conditions. However, gluconic acid is commonly detected in assays of bacterial and fungal solubilization of phosphates (Illmer and Schinner Citation1995; Mendes et al. Citation2013, Citation2014, Citation2014; Schneider et al. Citation2010; Whitelaw et al. Citation1999), and it is possible that gluconic acid plays a minor role in the solubilization of phosphate-containing minerals, for example, by reducing the pH. Furthermore, in the natural environment, each organic acid may not be produced exclusively, and they may work in synergy or compete with each other (Carlile et al. Citation2001).
Chemically-induced mineral phosphate solubilization has been widely studied and is considered to be the main mechanism of microbial phosphate release from sparingly soluble sources. This can be employed in bioprocesses based on microbially-produced organic acids as means to obtain soluble phosphate from RP (Goldstein et al. Citation1993; Mendes et al. Citation2013, Citation2015, Citation2017; Vassilev and Vassileva Citation2003), wastes, such as chars and ashes (Atienza-Martínez et al. Citation2014; Vassilev et al. Citation2009, 2013, Citation2013; Vassileva et al. Citation2012) and other insoluble phosphate minerals such as struvite (Suyamud et al. Citation2020). On the other hand, physical mechanisms of fungal phosphate bioweathering have been largely neglected, despite their potential importance in natural environments and in agroecosystems that rely on RP. In this regard, our results demonstrate active mineral colonization by fungi, including burrowing, tunneling and associated fragmentation and transformation, suggesting that physical interactions have an important role in RP bioweathering.
The physical force exerted by growing fungal hyphae has been well explored, but mainly focused on topics where fungi are pathogenic (Brand Citation2012; Money Citation2004; Money and Howard Citation1996), or where they cause damage to human-made structures (Gadd Citation2017c; Gadd et al. Citation2014; Sterflinger Citation2010). However, physical mechanisms of mineral biodeterioration have been demonstrated to be important in black slate bioweathering, along with biochemical mechanisms, by the fungus Schizophyllum commune (Kirtzel et al. Citation2020). Direct mineral interactions were also found to be necessary for more efficient bioweathering of cobalt-containing laterites and pyritic ores (Yang et al. Citation2019) and manganese oxides (Ferrier et al. Citation2019). Examples of fungal tunneling (Fomina et al. Citation2010; Hoffland et al. Citation2003; Jongmans et al. Citation1997; Pinzari et al. Citation2013) and ‘footprints’ (the marks left by fungal interactions) (Jongmans et al. Citation1997; Kirtzel et al. Citation2020; Pinzari et al. Citation2013; Wei et al. Citation2012) have been widely demonstrated as components of bioweathering, though importantly, not necessarily isolated from organic acids and their impact. We have evidence for similar mechanisms of fungal penetration of RP particles. This confirms that A. niger has a turgor pressure suitable for probing RP or other minerals, and that physical interactions are also important in the biodeterioration of RP. In the silicate mineral feldspar, fungal tunneling contributed to approximately 2% of the total weathering (Smits et al. Citation2005). Interestingly, the frequency of tunneling in mineral samples was found to increase with nutrient limitation (Hoffland et al. Citation2004), so it can be hypothesized that fungal tunneling of RP would also be more evident at limiting P, allowing for a more significant contribution of physical interactions to bioweathering. Indeed, it was demonstrated that mesh bags filled with apatite stimulated mycelial growth of mycorrhizal fungi around them, with a higher stimulus in P-deficient soil than in soil with adequate P levels (Hagerberg et al. Citation2003). Such mechanisms of phosphate solubilization in a free-living saprotrophic fungus such as A. niger supports the possibility of applying phosphate-solubilizing fungi as a soil inoculant in agriculture (Vassilev et al. Citation2019; Vassilev and Mendes Citation2018), especially in tropical areas where P-deficient soils predominate (Roy et al. Citation2016) and the use of RP as fertilizer is widespread. In the soil, where the production and diffusion of organic acids may be limited, direct hyphal interactions with RP particles are very likely to be an important contributory mechanism for phosphate solubilization.
In summary, this research reveals chemically- and physically-induced fungal transformation of RP particles, highlighting the important role of fungi in P cycling, as well as other associated elements (Gadd et al. Citation2014). Oxalic acid was shown to be a key factor in this process. Beyond participating in P mineral bioweathering in natural environments, these fungi present a potential to enhance phosphate uptake by plants in agronomic systems. In contrast to other studies using liquid media, our data demonstrate that A. niger can interact and transform RP in a solid matrix, which supports the idea of using fungal inoculants combined with RP to promote phosphate release in agricultural soil. The mechanisms exhibited by free-living saprophytic fungi may be critical in releasing phosphate to other organisms in colonizing ecosystems influenced by the geological, geomorphic, and agricultural change.
Conclusions
Fungi can cause chemical and physical alterations to rock phosphate particles, using mechanisms that actively participate in environmental P cycling. Oxalic acid has a key role in such transformation of RP and related phosphate minerals. Furthermore, the results demonstrate that the production of oxalic acid is responsible for the formation of the secondary biomineral whewellite as a result of apatite solubilization. Moreover, our data provide clear evidence of fungal penetration and tunneling through RP particles, showing that physical interactions are also important for RP solubilization and bioweathering.
Disclosure statement
No potential conflict of interest was reported by the author(s).
Additional information
Funding
References
- Adeyemi AO, Gadd GM. 2005. Fungal degradation of calcium-, lead- and silicon-bearing minerals. Biometals 18(3):269–281.
- Alori ET, Glick BR, Babalola OO. 2017. Microbial phosphorus solubilization and its potential for use in sustainable agriculture. Front Microbiol 8:971.
- Arcand MM, Schneider KD. 2006. Plant- and microbial-based mechanisms to improve the agronomic effectiveness of phosphate rock: a review. An Acad Bras Cienc 78(4):791–807.
- Atienza-Martínez M, Gea G, Arauzo J, Kersten SRA, Kootstra AMJ. 2014. Phosphorus recovery from sewage sludge char ash. Biomass Bioenergy 65:42–50.
- Brand A. 2012. Hyphal growth in human fungal pathogens and its role in virulence. Int J Microbiol 2012:517529.
- Burford EP, Fomina M, Gadd GM. 2003. Fungal involvement in bioweathering and biotransformation of rocks and minerals. Mineral Mag 67(6):1127–1155.
- Burgstaller W, Schinner F. 1993. Leaching of metals with fungi. J Biotechnol 27(2):91–116.
- Carlile MJ, Watkinson SC, Gooday GW. 2001. Fungi and biotechnology. In: The Fungi, 2nd ed. London (UK): Academic Press, p461–542.
- Ceci A, Kierans M, Hillier S, Persiani AM, Gadd GM. 2015. Fungal bioweathering of mimetite and a general geomycological model for lead apatite mineral biotransformations. Appl Environ Microbiol 81(15):4955–4964.
- Cordell D, Drangert J-O, White S. 2009. The story of phosphorus: global food security and food for thought. Glob Environ Chang 19(2):292–305.
- Cordell D, Neset T-SS. 2014. Phosphorus vulnerability: a qualitative framework for assessing the vulnerability of national and regional food systems to the multi-dimensional stressors of phosphorus scarcity. Glob Environ Chang 24(1):108–122.
- Duponnois R, Colombet A, Hien V, Thioulouse J. 2005. The mycorrhizal fungus Glomus intraradices and rock phosphate amendment influence plant growth and microbial activity in the rhizosphere of Acacia holosericea. Soil Biol Biochem 37(8):1460–1468.
- Ferrier J, Yang Y, Csetenyi L, Gadd GM. 2019. Colonization, penetration and transformation of manganese oxide nodules by Aspergillus niger. Environ Microbiol 21(5):1821–1832.
- Fomina M, Alexander IJ, Hillier S, Gadd GM. 2004. Zinc phosphate and pyromorphite solubilization by soil plant-symbiotic fungi. Geomicrobiol J 21(5):351–366.
- Fomina M, Burford EP, Hillier S, Kierans M, Gadd GM. 2010. Rock-building fungi. Geomicrobiol J 27(6–7):624–629.
- Fomina M, Charnock JM, Hillier S, Alvarez R, Gadd GM. 2007. Fungal transformations of uranium oxides. Environ Microbiol 9(7):1696–1710.
- Fomina M, Gadd GM. 2008. Metal and mineral transformations: a mycoremediation perspective. In: Robson GD, van West P, Gadd Geoffrey M., editors. Exploitation of Fungi. Cambridge (UK): Cambridge University Press, p236–254.
- Fomina M, Hillier S, Charnock JM, Melville K, Alexander IJ, Gadd GM. 2005. Role of oxalic acid overexcretion in transformations of toxic metal minerals by Beauveria caledonica. Appl Environ Microbiol 71(1):371–381.
- Gadd GM. 1999. Fungal production of citric and oxalic acid: importance in metal speciation, physiology and biogeochemical processes. Adv Microb Physiol 41:47–92.
- Gadd GM. 2007. Geomycology: biogeochemical transformations of rocks, minerals, metals and radionuclides by fungi, bioweathering and bioremediation. Mycol Res 111(1):3–49.
- Gadd GM. 2016. Geomycology. In: Purchase D, editor. Fungal Applications in Sustainable Environmental Biotechnology. Cham (Switzerland): Springer International Publishing, p371–401.
- Gadd GM. 2017a. The geomycology of elemental cycling and transformations in the environment. Microbiol Spectr 5(1):1–16.
- Gadd GM. 2017b. Fungi, rocks, and minerals. Elements 13(3):171–176.
- Gadd GM. 2017c. Geomicrobiology of the built environment. Nat Microbiol 2(4):16275.
- Gadd GM, Bahri-Esfahani J, Li Q, Rhee YJ, Wei Z, Fomina M, Liang X. 2014. Oxalate production by fungi: significance in geomycology, biodeterioration and bioremediation. Fungal Biol Rev 28(2–3):36–55.
- Gharieb MM, Sayer JA, Gadd GM. 1998. Solubilization of natural gypsum (CaSO4·2H2O) and the formation of calcium oxalate by Aspergillus niger and Serpula himantioides. Mycol Res 102(7):825–830.
- Gleeson DB, Melville K, McDermott F, Clipson N, Gadd GM. 2010. Molecular characterization of fungal communities in sandstone. Geomicrobiol J 27(6–7):559–571.
- Goldstein AH, Rogers RD, Mead G. 1993. Mining by microbe. Nat Biotechnol 11(11):1250–1254.
- Gorbushina AA. 2007. Life on the rocks. Environ Microbiol 9(7):1613–1631.
- Gosling P, Mead A, Proctor M, Hammond JP, Bending GD. 2013. Contrasting arbuscular mycorrhizal communities colonizing different host plants show a similar response to a soil phosphorus concentration gradient. New Phytol 198(2):546–566.
- Hagerberg D, Thelin G, Wallander H. 2003. The production of ectomycorrhizal mycelium in forests: relation between forest nutrient status and local mineral sources. Plant Soil 252(2):279–290.
- Hoffland E, Giesler R, Breemen N, van Jongmans AG. 2003. Feldspar tunneling by fungi along natural productivity gradients. Ecosystems 6(8):739–746.
- Hoffland E, Kuyper TW, Wallander H, Plassard C, Gorbushina AA, Haselwandter K, Holmström S, Landeweert R, Lundström US, Rosling A, et al. 2004. The role of fungi in weathering. Front Ecol Environ 2(5):258–264.
- Illmer P, Barbato A, Schinner F. 1995. Solubilization of hardly-soluble AlPO4 with P-solubilizing microorganisms. Soil Biol Biochem 27(3):265–270.
- Illmer P, Schinner F. 1995. Solubilization of inorganic calcium phosphates – solubilization mechanisms. Soil Biol Biochem 27(3):257–263.
- Jacobs H, Boswell GP, Harper FA, Ritz K, Davidson FA, Gadd GM. 2002. Solubilization of metal phosphates by Rhizoctonia solani. Mycol Res 106(12):1468–1479.
- Jones DL. 1998. Organic acids in the rhizosphere – a critical review. Plant Soil 205(1):25–44.
- Jones DL, Dennis PG, Owen AG, van Hees PAW. 2003. Organic acid behaviour in soils – misconceptions and knowledge gaps. Plant Soil 248(1–2):31–41.
- Jongmans AG, van Breemen N, Lundström U, van Hees PAW, Finlay RD, Srinivasan M, Unestam T, Giesler R, Melkerud P-A, Olsson M. 1997. Rock-eating fungi. Nature 389(6652):682–683.
- Kang X, Csetenyi L, Gadd GM. 2019. Biotransformation of lanthanum by Aspergillus niger. Appl Microbiol Biotechnol 103(2):981–993.
- Kang X, Csetenyi L, Gadd GM. 2020. Monazite transformation into Ce- and La-containing oxalates by Aspergillus niger. Environ Microbiol 22(4):1635–1648.
- Kirtzel J, Ueberschaar N, Deckert-Gaudig T, Krause K, Deckert V, Gadd GM, Kothe E. 2020. Organic acids, siderophores, enzymes and mechanical pressure for black slate bioweathering with the basidiomycete Schizophyllum commune. Environ Microbiol 22(4):1535–1546.
- Kpomblekou AK, Tabatabai MA. 1994. Effect of organic acids on release of phosphorus from phosphate rocks. Soil Sci 158(6):442–453.
- Kpomblekou AK, Tabatabai MA. 2003. Effect of low-molecular weight organic acids on phosphorus release and phytoavailabilty of phosphorus in phosphate rocks added to soils. Agric Ecosyst Environ 100(2–3):275–284.
- Kucey RMN, Janzen HH, Leggett ME. 1989. Microbially mediated increases in plant-available phosphorus. Adv Agron 42:199–228.
- Landeweert R, Hoffland E, Finlay RD, Kuyper TW, van Breemen N. 2001. Linking plants to rocks: ectomycorrhizal fungi mobilize nutrients from minerals. Trends Ecol Evol 16(5):248–254.
- Liang X, Gadd GM. 2017. Metal and metalloid biorecovery using fungi. Microb Biotechnol 10(5):1199–1205.
- Mendes GO, Murta HM, Valadares RV, Silveira WB, Silva IR, Costa MD. 2020. Oxalic acid is more efficient than sulfuric acid for rock phosphate solubilization. Miner. Eng. 155:106458.doi:10.1016/j.mineng.2020.106458.
- Mendes GO, da Silva NMRM, Anastácio TC, Vassilev NB, Ribeiro JI, da Silva IR, Costa MD. 2015. Optimization of Aspergillus niger rock phosphate solubilization in solid-state fermentation and use of the resulting product as a P fertilizer. Microb Biotechnol 8(6):930–939.
- Mendes GO, Dias CS, Silva IR, Júnior JIR, Pereira OL, Costa MD. 2013. Fungal rock phosphate solubilization using sugarcane bagasse. World J Microbiol Biotechnol 29(1):43–50.
- Mendes GO, Freitas ALM, Pereira OL, Silva IR, Vassilev NB, Costa MD. 2014. Mechanisms of phosphate solubilization by fungal isolates when exposed to different P sources. Ann Microbiol 64(1):239–249.
- Mendes GO, Galvez A, Vassileva M, Vassilev N. 2017. Fermentation liquid containing microbially solubilized P significantly improved plant growth and P uptake in both soil and soilless experiments. Appl Soil Ecol 117–118:208–211.
- Mendes GO, Vassilev NB, Bonduki VHA, Silva IR, Ribeiro JI, Costa MD. 2013. Inhibition of Aspergillus niger phosphate solubilization by fluoride released from rock phosphate. Appl Environ Microbiol 79(16):4906–4913.
- Mendes GO, Zafra DL, Vassilev NB, da Silva IR, Ribeiro JI, Costa MD. 2014. Biochar enhances Aspergillus niger rock phosphate solubilization by increasing organic acid production and alleviating fluoride toxicity. Appl Environ Microbiol 80(10):3081–3085.
- Money NP. 2004. The fungal dining habit: a biomechanical perspective. Mycologist 18(2):71–76.
- Money NP, Howard RJ. 1996. Confirmation of a link between fungal pigmentation, turgor pressure, and pathogenicity using a new method of turgor measurement. Fungal Genet Biol 20(3):217–227.
- Nautiyal CS. 1999. An efficient microbiological growth medium for screening phosphate solubilizing microorganisms. FEMS Microbiol Lett 170(1):265–270.
- Nelson PN, Su N. 2010. Soil pH buffering capacity: a descriptive function and its application to some acidic tropical soils. Soil Res 48(3):201.
- Oelkers EH, Valsami-Jones E. 2008. Phosphate mineral activity and sustainability. Elements 4(2):83–87.
- Pinzari F, Tate J, Bicchieri M, Rhee YJ, Gadd GM. 2013. Biodegradation of ivory (natural apatite): possible involvement of fungal activity in biodeterioration of the Lewis Chessmen. Environ Microbiol 15(4):1050–1062.
- Pollard W. 2018. Periglacial processes in glacial environments. In: Menzies J, van der Meer J, editors. Past Glacial Environments, 2nd ed. Amsterdsm (The Netherlands): Elsevier Inc., p537–564.
- Pylro VS, Freitas Ad, Otoni WC, Silva Id, Borges AC, Costa MD. 2013. Calcium oxalate crystals in eucalypt ectomycorrhizae: morphochemical characterization. PLoS One 8(7):e67685.
- Rajan SSS, Watkinson JH, Sinclair AG. 1996. Phosphate rocks for direct application to soils. Adv Agron 57:77–159.
- Richardson A, Lynch J, Ryan P, Delhaize E, Smith FA, Smith S, Harvey P, Ryan M, Veneklaas E, Lambers H, et al. 2011. Plant and microbial strategies to improve the phosphorus efficiency of agriculture. Plant Soil 349(1–2):121–156.
- Richardson AE, Simpson RJ. 2011. Soil microorganisms mediating phosphorus availability update on microbial phosphorus. Plant Physiol 156(3):989–996.
- Rockström J, Steffen W, Noone K, Persson A, Chapin FS, Lambin EF, Lenton TM, Scheffer M, Folke C, Schellnhuber HJ, et al. 2009. A safe operating space for humanity. Nature 461(7263):472–475.
- Roy ED, Richards PD, Martinelli LA, Della CL, Lins SRM, Vazquez FF, Willig E, Spera SA, VanWey LK, Porder S. 2016. The phosphorus cost of agricultural intensification in the tropics. Nat Plants 2(5):16043.
- Sammauria R, Kumawat S, Kumawat P, Singh J, Jatwa TK. 2020. Microbial inoculants: potential tool for sustainability of agricultural production systems. Arch Microbiol 202(4):677–617.
- Sayer JA, Cotter-Howells JD, Watson C, Hillier S, Gadd GM. 1999. Lead mineral transformation by fungi. Curr Biol 9(13):691–694.
- Schneider KD, van Straaten P, de Orduna RM, Glasauer S, Trevors J, Fallow D, Smith PS. 2010. Comparing phosphorus mobilization strategies using Aspergillus niger for the mineral dissolution of three phosphate rocks. J Appl Microbiol 108(1):366–374.
- Silva UdC, Mendes GdO, Silva NMRM, Duarte JL, Silva IR, Tótola MR, Costa MDMD. 2014. Fluoride-tolerant mutants of Aspergillus niger show enhanced phosphate solubilization capacity. PLoS One 9(10):e110246.
- Smits MM, Hoffland E, Jongmans AG, van Breemen N. 2005. Contribution of mineral tunneling to total feldspar weathering. Geoderma 125(1–2):59–69.
- Sterflinger K. 2010. Fungi: their role in deterioration of cultural heritage. Fungal Biol Rev 24(1–2):47–55.
- Sullivan TS, Gadd GM. 2019. Metal bioavailability and the soil microbiome. Adv Agron 155:79–120.
- Suyamud B, Ferrier J, Csetenyi L, Inthorn D, Gadd GM. 2020. Biotransformation of struvite by Aspergillus niger: phosphate release and magnesium biomineralization as glushinskite. Environ Microbiol 22(4):1588–1602.
- Vassilev N, Malusa E, Requena AR, Martos V, López A, Maksimovic I, Vassileva M. 2017. Potential application of glycerol in the production of plant beneficial microorganisms. J Ind Microbiol Biotechnol 44(4–5):735–743.
- Vassilev N, Martos E, Mendes G, Martos V, Vassileva M. 2013. Biochar of animal origin: a sustainable solution to the global problem of high-grade rock phosphate scarcity? J Sci Food Agric 93(8):1799–1804.
- Vassilev N, Medina A, Mendes G, Galvez A, Martos V, Vassileva M. 2013. Solubilization of animal bonechar by a filamentous fungus employed in solid state fermentation. Ecol Eng 58(0):165–169.
- Vassilev N, Mendes GO. 2018. Solid-state fermentation and plant-beneficial microorganisms. In: Pandey A, Larroche C, Soccol CR, editors. Current Developments in Biotechnology and Bioengineering. Amsterdam (The Netherlands): Elsevier, p435–450.
- Vassilev N, Mendes G, Costa M, Vassileva M. 2014. Biotechnological tools for enhancing microbial solubilization of insoluble inorganic phosphates. Geomicrobiol J 31(9):751–763.
- Vassilev N, Serrano M, Jurado E, Bravo V, Vassileva I, Vassileva M. 2009. Effect of microbially treated agro-wastes and simultaneously solubilized animal bone char on Zn and P uptake and growth of white clover in Zn-contaminated soil. N Biotechnol 25:S312.
- Vassilev N, Vassileva M. 2003. Biotechnological solubilization of rock phosphate on media containing agro-industrial wastes. Appl Microbiol Biotechnol 61(5–6):435–440.
- Vassilev N, Vassileva M, Martos V, Galvez A, Flor-Peregrin E, Garcia del Moral LF. 2019. Phosphate sources, microorganisms, and P plant nutrition: challenges and future trends. Arch Crop Sci 3(1):61–63.
- Vassileva M, Eichler-Lobermann B, Reyes A, Vassilev N, Vassileva M, Eichler-Lobermann B, Reyes A, Vassilev N. 2012. Animal bones char solubilization by gel-entrapped Yarrowia lipolytica on glycerol-based media. Sci World J 2012:907143.
- Vassileva M, Serrano M, Bravo V, Jurado E, Nikolaeva I, Martos V, Vassilev N. 2010. Multifunctional properties of phosphate-solubilizing microorganisms grown on agro-industrial wastes in fermentation and soil conditions. Appl Microbiol Biotechnol 85(5):1287–1299.
- Wei Z, Kierans M, Gadd GM. 2012. A model sheet mineral system to study fungal bioweathering of mica. Geomicrobiol J 29(4):323–331.
- Whitelaw MA. 1999. Growth promotion of plants inoculated with phosphate-solubilizing fungi. Adv Agron 69:99–151.
- Whitelaw MA, Harden TJ, Helyar KR. 1999. Phosphate solubilisation in solution culture by the soil fungus Penicillium radicum. Soil Biol Biochem 31(5):655–665.
- Withers PJA, Elser JJ, Hilton J, Ohtake H, Schipper WJ, Van Dijk KC. 2015. Greening the global phosphorus cycle: how green chemistry can help achieve planetary P sustainability. Green Chem 17(4):2087–2099.
- Yang Y, Ferrier J, Csetenyi L, Gadd GM. 2019. Direct and indirect bioleaching of cobalt from low grade laterite and pyritic ores by Aspergillus niger. Geomicrobiol J 36(10):940–949.
- Zapata F, Roy RN, editors. 2004. Use of Phosphate Rocks for Sustainable Agriculture. Rome (Italy): FAO Land and Water Development Division and the International Atomic Energy Agency.