Abstract
The present study examines the heating efficiency of a combination of manganese or cobalt ferrites in a binary (Co- or Mn-) ferrite nanoparticle form with magnetite, covered with citric acid to improve biocompatibility. The nanoparticle synthesis is based on the aqueous co-precipitation of proper salts, a facile, low-cost, environmentally friendly and high yield synthetic approach. By detailed structural and magnetic characterisation, the direct influence of structural and magnetic features on magnetic hyperthermia concludes to optimum heating efficiency. At a second stage, best performing magnetic nanoparticles undergo in vitro testing in three cell lines: one cancer cell line and two reference healthy cell lines. Both binary ferrite (MnFe2O4/Fe3O4 and CoFe2O4/Fe3O4) appear to be internalised and well tolerated by the cells while a versatile hyperthermia protocol is attempted in an effort to further improve their in vitro performance. Within this protocol, hyperthermia sequences are split in two runs with an intermediate 48 h time interval cell incubation stage while in each run a variable field mode (single or multiple pulses) is applied. Single-pulse field mode represents a typical hyperthermia application scheme where cells undergo the thermal shock continuously. On the other hand multiple-pulses mode refers to multiple, much shorter in duration AC field changes (field ON/OFFs), at each hyperthermia run, resulting eventually in high heating rate and much more harmful cell treatment. Consequently, we propose a novel series of improved performance heat mediators based on ferrite structures which show maximum efficiency at cancer cells when combined with a versatile multiple-pulse hyperthermia module.
Introduction
Magnetic nanoparticles (MNPs) dispersed in diverse media are currently the subject of basic and applied research, due to their properties that can lead to a wide variety of applications in technological and biomedical fields [Citation1], such as genetic engineering [Citation2], bound biomolecules [Citation3], cell separation [Citation4], cell signalling [Citation5], gene delivery and gene therapy [Citation6], contrast enhancement in magnetic resonance imaging (MRI) [Citation7,Citation8], site-specific drug delivery [Citation9–11], and Magnetic Particle Hyperthermia (MPH) [Citation12,Citation13].
Hyperthermia incorporating magnetic materials as heat moderators was developed in the 1950s [Citation14] and is still under development with MNPs as heating mediators, in an effort to successfully deal with current applicability constraints [Citation15–17]. With the development of precise methods for synthesising functionalised nanoparticles [Citation18,Citation19], MNPs with high tumour tissue specificity have been developed as heating elements for magnetic hyperthermia [Citation20]. The current development of MPH is heavily focussed on two aspects, namely, the composition of nanoparticles (where reproducibility and scalability are consistently found hard to achieve), and the instrumentation needed for applying external fields to generate the MPH and for measuring the resulting heat deposition at cellular level. Regarding the instrumentation, the vast majority of devices are purpose-built designs intended for in vitro testing, or pre-clinical in vivo testing; clinical scale appliances are very much the exception to date [Citation21,Citation22].
Herein, we report a strategy to enhance the in vitro efficiency of MPH by a two-fold approach. Firstly, we propose novel materials as heat mediators with much enhanced heating efficiency, while, secondly, we maximise their in vitro performance by applying versatile hyperthermia protocols, i.e., by multiplying the AC magnetic field pulses. Due to large differences in the physiology of cells depending on their tissue origin and proliferation rate (cancer or healthy cells), we decided to include different cell types in this study to address such aspects and demonstrate the selective efficiency of the proposed methodology on neoplastic cells. In particular, this work deals with osteosarcoma (SaOS-2), which represents the most common histological form of primary bone cancer and also ranks high as a common malignant bone tumour in children and adolescents. Recent advances in multimodality treatments consisting of aggressive adjuvant chemotherapy and wide local excision have markedly improved the prognosis of patients with osteosarcoma [Citation23]. However, metastasis occurs in approximately 40 to 50% of the patients with osteosarcoma increasing mortality rate [Citation24]. Osteoblastic properties of the human osteosarcoma cell line SaOS-2, which was isolated from an 11-year old Caucasian female in 1975, were well characterised by Rodan and colleagues [Citation25]. 3T3-L1 fibroblast-like preadipocytes and C2-C12 skeletal myoblasts were used as reference healthy cell lines. 3T3-L1 differentiation is an economical and convenient pathway to generate adipocyte-like cells for experiments [Citation26]. C2-C12 mouse skeletal myoblasts are a well-established cell line frequently used to study the differentiation of myoblasts and osteoblasts, to express various proteins, and also to explore mechanistic pathways [Citation27].
Materials and methods
Materials & properties
In this work, binary MnFe2O4/Fe3O4 and CoFe2O4/Fe3O4 magnetic nanoparticles were synthesised in a two-stage high-yield green procedure by aqueous co-precipitation route of Mn + 2, Co + 2 and Fe + 3 precursors. In particular, iron (II) sulphate heptahydrate and citric acid monohydrate were purchased from Riedel-de Haen. Cobalt (II) sulphate heptahydrate was purchased from Merck. Absolute ethanol and n-hexane were purchased from Analar Normapur (VWR). Mn(II) sulphate monohydrate (98%), iron (III) sulphate hydrate (97%), iron (III) acetylacetonate (97%), iron (II) acetate (97%), cobalt (II) acetate tetrahydrate (reagent grade), manganese (II) acetate tetrahydrate (99%), oleylamine (70%), oleic acid (90%), octadecene (90%) and sodium citrate dihydrate (99%) were purchased from Sigma Aldrich. All the chemicals were used as received, without further purification. Initially, high-crystalline ferrite nanoparticles either MnFe2O4 or CoFe2O4 were synthesised and served as nuclei-seeds at the second synthetic stage where Fe3O4 synthesis was attempted. The magnetically soft magnetite presumably grows as a surrounding shell on a magnetically hard ferrite core, as witnessed by the increased diameter size. Such an approach is followed in order to combine two magnetically different phases via exchange-coupling in a hard/soft interface to enhance magnetic performance. An overview of structural and magnetic features may be found in SI while the details of the proposed synthetic procedure together with correlation between structural and magnetic features appear in [Citation28]. Thus, MNPs’ magnetic profile may be eventually tuned and lead to superior magnetic hyperthermia response [Citation29–31]. At the same time, magnetite serves as a biocompatible “blanket” together with outer coverage of sodium citrate, also incorporated at the final synthetic stage, to successfully preclude the usual toxicity issues in ferrite biomedical applicability schemes [Citation32]. In the following, the two binary ferrite samples, MnFe2O4/Fe3O4 and CoFe2O4/Fe3O4, will be referred to as Mn + Fe and Co + Fe, respectively.
Magnetic heating
Magnetic nanoparticles of several concentrations were dispersed in 1 mL volume of distilled water (aqueous solutions) and in 1 mL volume of DMEM (Dulbecco’s Modified Eagle Medium). Magnetic heating was performed using an induction heating system (SPG-10: Ultrahigh Frequency Induction Heating Machine, Shuangping Corporation) by placing the sample at the centre of a water cooled, two-turn coil that generated an AC magnetic induction with amplitude of 30 mT and frequency of 765 kHz. In all cases, magnetic heating and cooling sequences were recorded for approximately 10 min for each sequence with an optical fibre thermometer. During the in vitro process, two individual experiments were conducted (single or multiple pulses) each one comprising of two separate stages (1st, 2nd hyperthermia run as denoted in ). Special care was taken, by tuning the magnetic induction amplitude between 25 and 30 mT, to maintain the sample’s temperature within the hyperthermia region (41–45 °C).
Figure 1. Schematics of the experimental sequences followed for the in vitro manipulation of a cell line culture with versatile magnetic particle hyperthermia protocols. Indexes denote in vitro assays performed at each stage: 1: Detached with trypsin, 2: Cytotoxicity with Trypan Blue, 3: Caspase 3/7 activity.
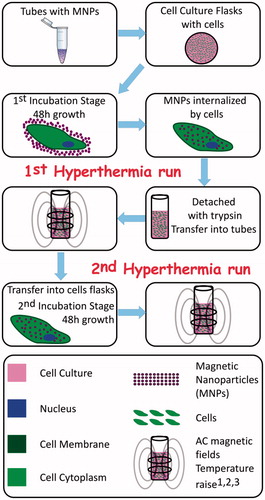
Thermal dosimetry
For hyperthermia, the goal is to heat the tumour to a defined temperature range for a set period of time while its therapeutic benefit is dependent upon the temperature achieved and duration of heating. Thermal dosimetry is critical to the optimisation of hyperthermia treatment as well as to the minimisation of potential side-effects of heat-related toxicity in surrounding healthy tissues [Citation33]. The urge to use descriptors for temperature variations in dosimetry arises from the fact that thermal gradients exist within the treated region during hyperthermia application with a most common method the CEM43 dosimetry. CEM43 dosimetry is a powerful tool in the establishment of regulatory guidelines (i.e., safety thresholds) for exposure to RF and microwave fields. Sapareto and Dewey introduced the concept of the thermal isoeffect dose, to quantify thermal dose as “equivalent heating minutes” at 43 °C [Citation34,Citation35] in an effort to compare the heating impact in diverse target locations and clinical circumstances. Using this method any time–temperature history is converted to an equivalent number of minutes of heating at 43 °C, using the following formula:
(1)
where CEM43 °C is the cumulative number of equivalent minutes at 43 °C, ti is the i-th time interval, R is related to the temperature dependence of the rate of cell death (R(T< 43 °C) = 1/4, R(T > 43 °C) = 1/2) and Ti is the average temperature during time interval ti. The resulting CEM43°C value represents the effect of the entire history of heat exposure on cell death [Citation36,Citation37]. CEM43 dosimetry has provided thresholds for establishing regulatory guidelines for exposure to RF fields and microwave radiation. The amount of damage is related to the temperature achieved and the duration of heating with thermal convection and diffusion affecting them the temperatures achieved for any set amount of power delivered to a tissue [Citation38]. Two warm water bath experimental runs were performed, at 45 °C and 43 °C, to have an equivalent thermal dose with the calculated heating dose (CEM43°C) of the single- and multiple-pulses MPH experiments, respectively.
Cell cultures
SaOS-2 human osteosarcoma cells, C2-C12 mouse adherent myoblasts and 3T3-L1 cells were cultured in DMEM (Dulbecco's Modified Eagle Medium) nutrient mixture F-12 Ham, supplemented with 1% L-glutamine, 10% of FBS (fetal bovine serum) and 1% penicillin/streptomycin at 37 °C and 5% CO2. The cells were seeded in 6 well plates until 70% confluency and then incubated with the magnetic fluid for 48 h. After washing with PBS under gentle shaking, cells were detached with 5% trypsin-EDTA (3–10 min incubation) and transferred to a Neubauer plate.
In vitro treatments – cell assays
The separate steps and stages followed to reach the final goal of the in vitro MPH application are illustrated in .
To proceed with in vitro studies, all three cell lines were incubated with binary ferrite samples, MnFe2O4/Fe3O4 and CoFe2O4/Fe3O4, with optimum structural and magnetic features to comparatively evaluate their in vitro hyperthermia performance. Initial MNPs’ concentration was 1 mg/mL while final MNPs’ concentration was re-measured in in vitro samples after all assays were completed in order to have the final MNPs’ quantity adsorbed by the cancer cells and found to be nearly ∼0.5 mg/mL for both MNPs indicating some material loss after the cell washing procedures. Wherever error bars appear in in vitro assay graphs, these correspond to the statistical result of four similar sample packs measured.
To clarify if nanoparticles are internalised by cells, Prussian blue staining was performed. After 48 h incubation with nanoparticles cells were washed with PBS and fixed with 4% formaldehyde for 15 min at room temperature. Following the washing with distilled water, cells were incubated with a mix of 10% potassium ferrocyanide in water and 20% hydrochloride solution, in 1:1 ratio, for 20 min at room temperature. After washing with distilled water and counterstaining with Nuclear Fast Red staining, optical microscopy was performed.
For the cytotoxicity assessment, the Trypan Blue exclusion test was performed just before and immediately after each sample’s hyperthermia sequence (1st and 2nd run respectively). Two control samples served as reference: (a) cells without MNPs but with magnetic field exposure, and (b) cells with MNPs and without magnetic field exposure. Since, our goal was to initially evaluate if our combinatory protocol was of any effectiveness on cell viability, it was necessary to look over a routine and convenient assay for determination of magnetism-induced cell viability. Moreover, in vitro assays should be carried out with care since cells in culture do not experience the phase of pathogenic effects observed in vivo while MNPs may also interact with the assay components, interfere with the readout and sometimes contribute to erroneous results [Citation39]. Thus, Trypan Blue was selected against MMT and clonogenic assays as an easy and rapid method for checking cell viability effects while adjusting protocol’s dosage and cell density [Citation40].
To investigate if the observed hyperthermia-induced cell viability decrease is caused by caspase-dependent apoptosis, a Caspase-Glo®3/7 assay (Promega, Madison, WI) was performed according to manufacturer's instructions. In particular, 20000 hyperthermia-treated or control, SaOS-2 osteoblasts, C2-C12 mouse adherent myoblasts or 3T3-L1 fibroblast-like preadipocytes, in 50 μL culture medium, were seeded in the appropriate well of a white-walled 96-well plate. Fifty microliters of Caspase-Glo®3/7 reagent were added to each well and, after a 30 s gentle mixing, a one hour incubation at room temperature was carried out. Luminescence of each sample was measured in the GloMax® 96 Microplate Luminometer (Promega Corporation, Madison, WI) and results were expressed in relative luminescence units (RLU). Background luminescence of blank wells without cells was subtracted from all samples.
Results and discussion
Nanoparticle internalisation
Optical microscope images presented in were taken after two days of cells’ incubation with MNPs using a control sample (cells without MNPs) as a reference (). Cells appearing stained with blue or partially blue colour () indicate the presence of nanoparticles inside them due to Prussian blue staining on the corresponding MNPs. Furthermore, an initial approximation of particles’ cytotoxic effect can be considered by visual comparison of cell morphologies in these three images. Both nanoparticle samples incubated with cells exhibit non-toxic behaviour, even after two days incubation.
Figure 2. Optical microscopy imaging 17 × of control SaOS-2 sample (a). Prussian Blue stained SaOS-2 after 48 h incubation with MnFe2O4/Fe3O4 (Mn + Fe) (b) and Prussian Blue stained SaOS-2 after 48 h incubation with CoFe2O4/Fe3O4 (Co + Fe) (c) The cells’ colour became blue or partially blue (b and c), indicating the MNPs internalisation by the cancer cells.
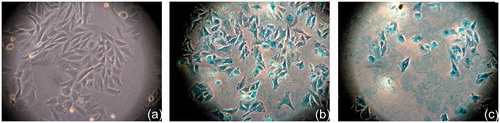
The impact of binary MNPs
In our previous study [Citation41] SaOS-2 osteoblasts as well as two healthy cell lines (primary bone marrow-derived osteoblasts, 3T3-L1 fibroblast-like preadipocytes) were incubated with uncovered manganese ferrite nanoparticles to comparatively evaluate their in vitro hyperthermia performance. An analogous two-run hyperthermia treatment was applied resulting to a reduction of SaOS-2 viability, while healthy cell lines were able to survive. To go further, in the current work, manganese and cobalt ferrites covered initially by magnetite and eventually by sodium citrate, exhibiting greater magnetic features due to evolving binary structures, were chosen to be the heating agents. presents hyperthermia curves for the single- pulse case for both 1st and 2nd runs, which were separated by a 48 h incubation interval, together with the control sample (no MNPs present in cells) depicting the temperature rise due to magnetic field exposure.
Figure 3. In vitro single-pulse hyperthermia on SaOS-2. Sequences performed in two runs with a 48 h interval in-between: Graph 3a corresponds to first hyperthermia run while Graph 3b corresponds to second hyperthermia run. Blue/red curves refer to cell samples incubated with MnFe2O4/Fe3O4 (Mn + Fe) and CoFe2O4/Fe3O4 (Co + Fe) MNPs respectively while green curve corresponds to heating response of control sample (cells without particles). Samples (cells with particles under field) in both cases safely enter the hyperthermia region (41–45 °C) for ambient time.
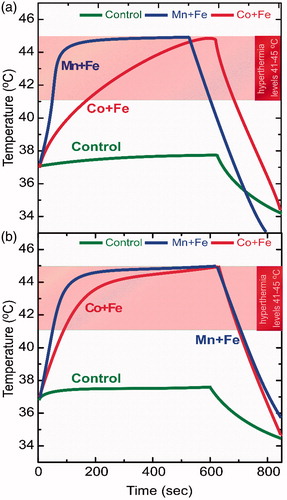
The binary ferrite combination seems to enhance magnetic particle hyperthermia efficiency due to adequate magnetic mixing and matching between two distinct magnetic phases at the nanoscale level, as we have recently discussed [Citation30,Citation31,Citation28]. It should be noted here that, during the in vitro experiments, it is crucial not to overcome an upper hyperthermia boundary of 45 °C in order not to reach thermoablative temperatures or change the biological mode of interaction. Therefore, the AC field application sequence ended whenever approaching this temperature limit. The resulting heating duration and the corresponding temperature range (41–45 °C) were kept the same in all subsequent experiments for comparison reasons. As shown in , in both hyperthermia runs, samples are easily entering the required temperature region and remain there for ambient time (up to 600 s), therefore a temperature shock is naturally expected in the corresponding cell life cycle.
The role of apoptosis
presents the viability results of the two-run MPH treatment for the single-pulse protocol performed in SaOS-2 osteoblasts (shown in ). In agreement with our previous work again on SaOS-2 osteoblasts [Citation41] the cytotoxicity effect is found pronounced when an additional (2nd) hyperthermia run is following the initial (1st) AC field application. Cell death may be eventually induced by apoptotic mechanisms, which may be more intense due to the longer AC magnetic field application together with the fact that the AC field application facilitates the cell uptake of MNPs [Citation42]. The occurrence of a caspase 3/7-dependent apoptotic mechanism is depicted in (1st and 2nd hyperthermia run respectively) appearing more intense for the case of Mn + Fe MNPs.
Figure 4. (a) Viability results of single-pulse hyperthermia for SaOS-2 osteoblasts incubated with MnFe2O4/Fe3O4 (Mn + Fe) and CoFe2O4/Fe3O4 (Co + Fe) for two hyperthermia cycles with respect to control sample (cells + MNPs without magnetic field exposure). (b) and (c): Caspase 3/7 activity for human osteosarcoma cell line SaOS-2 for 1st and 2nd hyperthermia run respectively: SaOS-2 osteoblasts incubated with MNPs together with the (reference) control sample of SaOS-2 osteoblasts incubated without MNPs, where “H” denotes the hyperthermia treated samples (only magnetic field exposure in case of control sample).
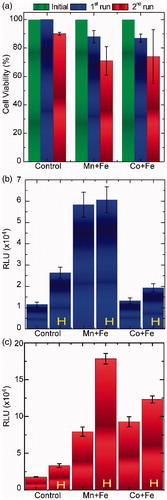
In each case, two samples appear, one is the control sample with MNPs but without exposure to the AC field and the other, denoted with H, is the actual sample under study. Control samples without MNPs, appear also as reference, where the sole role of the AC field is evaluated. It is evident that the incubation of cells with MNPs and the subsequent AC field application have a dramatic effect on cell viability, as expressed by a statistically significant increased caspase 3/7 activity. Moreover, the non-induced apoptosis in control samples (leftmost blue and red columns) in , respectively, seems to be in accordance with the zero-level or very low percentages of cell viability decrease depicted in (leftmost control sample columns).
Consequently, caspase 3/7-dependent apoptosis appears to be induced and tuned (via the two-run protocol) only in MPH treated SaOS-2 osteoblasts, while MNPs or AC field exposure alone do not significantly affect cell viability rates.
Continuous vs. pulsing magnetic field exposure
In this section we report the use of a new protocol for MPH that uses pulsing instead of continuous exposure of cells to the magnetic field. This protocol results in multiple-pulses hyperthermia, again in two runs, as depicted in , left and right panel, respectively. Here, the plotted area is zoomed in the temperature region of 41–45 °C to directly illustrate if the prerequisite hyperthermia conditions are satisfied for SaOS-2 osteoblasts (top row), C2-C12 (middle row) and 3T3-L1 (bottom row).
Figure 5. In vitro multiple-pulse hyperthermia protocol on SaOS-2 (top), C2-C12 (middle), 3T3-L1 (bottom). Multiple-pulses hyperthermia sequences performed in two runs with a 48 h interval in-between: Left column graphs (5a, 5b, 5c) correspond to 1st hyperthermia run while right column (5d, 5e, 5f) correspond to 2nd hyperthermia run. Blue and red curves refer to cell samples incubated with MnFe2O4/Fe3O4 (Mn + Fe) and CoFe2O4/Fe3O4 (Co + Fe) MNPs, respectively.
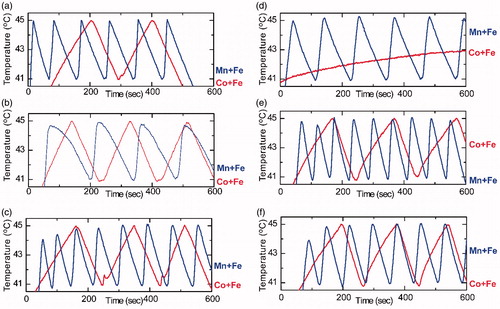
In order to characterise the various treatment modalities shown in and , the thermal isoeffect dose in terms of CEM43°C was calculated (). It is clear from that CEM43°C maintained quite high values (15–27 min) in single-pulse runs when compared with the much lower thermal dose of multiple-pulses ones (5–15 min). Such a difference, between the single- and multiple-pulses protocols, was actually expected, since samples were exposed for longer time at elevated temperatures (>43 °C) when the single-pulse mode was applied.
Table 1. Equivalent thermal dose (CEM43) for each individual hyperthermia section of single and multiple pulses together with total number of pulses and the Initial Heating Rate (IHR) at 41 °C.
In an effort to replicate the thermal conditions present, during single- and multiple-pulses MPH experiments, SaOS-2 cells were subjected to two independent warm bath experiments. One experiment where the thermal dose was kept similar to the continuous MPH one, denoted “warm bath” hereafter, and one with similar multiple-pulses conditions as in MPH experiments, denoted as “pulses” hereafter. The former, refers to a single dip of the sample in a constant temperature warm water bath of 43 °C for a specific time period, according to the first column of (CEM43°C values) to deliver to the sample an identical thermal dose as in the single-pulse MPH experiment. The latter, incorporated multiple sample dips in a warm water bath of 52 °C, where sample was allowed to heat from 41 to 45 °C as many times as multiple-pulses MPH dictated (“Number of Pulses” column in ).
depicts a comparative overview of heating impact on cell viability between magnetic (MPH) and non-magnetic (warm water bath) origins. Cell viability results of single () and multiple () pulses of MPH measurements are presented in dark blue and dark red colour for 1st and 2nd run, respectively. It is clear that viability rates are not related with the thermal dose. If thermal dose was the dominant cause of cell viability reduction, light blue bars (water bath cell viabilities) should have been equally reduced compared with deep blue bars (cell viabilities after 1st magnetic hyperthermia run) in both approaches (single- and multiple- pulses).
Figure 6. Cell viability comparison of two-run (a) single and (b) multiple-pulse hyperthermia sequences on SaOS-2 osteoblasts in comparison with an equivalent thermal dose (CEM43) warm bath (single: one dip or pulses: dipping in and out). (c) Comparative viability results of multiple-pulses hyperthermia for reference cell line 3T3-L1 incubated with MnFe2O4/Fe3O4 (Mn + Fe) and CoFe2O4/Fe3O4 (Co + Fe) for two hyperthermia runs with respect to control sample (cells + MNPs without treatment).
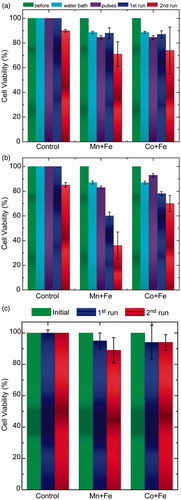
Additionally, the second warm bath experiment, replicating the multiple-pulse conditions (appearing as purple bars in and denoted as “pulses”), seems to be well tolerated by SaOS-2 cells. On the contrary, a similar heating of SaOS-2 with a steep rate, as induced by MPH (magnetic nanoparticles internalised by cells), had a substantially harmful effect on cell viability. It should be mentioned that, in warm bath hyperthermia, heat has to be first absorbed by the culture tube and then diffuse into the cells. The glass bottle apparently acts as a huge heat sink. It absorbed heat energy and transferred the energy gradually to the media; whereas in AMF hyperthermia, heat was produced directly from the cellular solution.
In order to investigate whether the role of heating rate together with pulse repetition would be able to compensate the corresponding CEM43°C decrease from continuous hyperthermia, the in vitro cytotoxic effect was examined after employing 4–9 pulses for the Mn + Fe and 1–3 pulses for the Co + Fe system in two hyperthermia runs as illustrated in . Depending on the MNPs heating efficiency, several pulses of varying rise time (initial heating rate, IHR, in ) were applied in each individual experimental hyperthermia session. The differences between Mn + Fe and Co + Fe samples with respect to cell killing may be directly associated with the superior heating efficiency (rate) of Mn + Fe MNPs (). Heating efficiency contrast between the two samples can be supported by the structural and magnetic properties as well as by the magnetic hyperthermia’s efficacy in aqueous solution of the binary systems, as it is described in SI (Figure S1). This effect becomes more pronounced, in the 2nd multiple-pulses MPH run, when the Mn + Fe steep heating slope of 230 × 10 − 3 oC/s is applied 6 times against the Co + Fe gradual heating rate of 6 × 10 − 3 oC/s applied only once, resulting in a dramatic difference of final cell viability. Heating rate is an important determinant of time-dependent thermal killing and should be also considered when evaluating cell toxicity results [Citation43–45]. Tang and McGoron [Citation46] have shown in vitro that rapidly increasing temperature from 37 °C to 43 °C leads to greater cell membrane damages compared with the gradual induction of a similar heat shock. Alvarez-Berríos et al. have also provided in their study the convincing evidence that cell membrane permeability induced by AMF hyperthermia is significantly greater than induced by hot water hyperthermia under similar temperature conditions [Citation47]. Moreover, as recently reported, nanoparticles deliver much more efficiently their heat-cargo, if positioned at the cell membrane acting as thermal nanoseeds [Citation48].
A point worth noting here is that MPH did not have a cytotoxic effect of the same intensity on healthy cells ( and Figure S2). Such a cell-specific effect of MPH may be attributed to the differences of membrane composition and fluidity between cells [Citation49] or the location of MNPs in the cell: As recently discussed, the extent and timeline of the cell death over a population may be controlled not only by varying the level of the administered thermal load, but also with membrane ruptures via moderate thermal loads, where intracellular effects do not depend on heating effects in the local environment [Citation50].
Conclusion
Manganese and cobalt ferrites combined with magnetite and covered by citric acid, synthesised by a high-yield “green” approach, possess remarkable structural and magnetic properties and display a great potential as MPH agents. Their low inherent toxicity, ensured by magnetite and citric acid, allows them to be internalised and deliver their cargo (heat) inside target cells. In the present study such MNPs were used in combination with a versatile in vitro hyperthermia protocol consisting of two hyperthermia runs separated by a 48 h incubation stage with the latter run resulting in a maximised cytotoxicity effect on SaOS-2 cells. Additional variation of in vitro hyperthermia protocol by altering AC single-pulses (continuous hyperthermia) with multiple pulses (intermittent MNPs exposure to magnetic field) led to further enhancement of the cytotoxic effect specifically on SaOS-2 cells. On the contrary, two reference healthy cell lines (C2-C12 and 3T3-L1 fibroblast-like preadipocytes) managed to survive the treatment fairly well.
Water bath experiments ruled out the thermal isoeffect dose of CEM43°C as the ultimate cause for the highest effect of multiple pulses. The experimental results support the conclusion that heating rate and location of heating may be the dominant factors in this case. Consequently, a pulsed magnetic field combined with the enhanced heating efficiency of binary MNPs can lead to a promising tumour-selective MPH protocol.
Supplemental Files
Download Zip (765.4 KB)Disclosure statement
The authors report no conflicts of interest. The authors alone are responsible for the content and writing of this article.
References
- Wu W, Wu Z, Yu T, et al. (2015). Recent progress on magnetic iron oxide nanoparticles: synthesis, surface functional strategies and biomedical applications. Sci Technol Adv Mater 16. doi:10.1088/1468-6996/16/2/023501.
- Sakamoto JH, Van De Ven AL, Godin B, et al. (2010). Enabling individualized therapy through nanotechnology. Pharmacol Res 62:57–89.
- Tseng P, Judy JW, Di Carlo D. (2012). Magnetic nanoparticle-mediated massively parallel mechanical modulation of single-cell behavior. Nat Methods 9:1113–19.
- Plouffe BD, Murthy SK, Lewis LH. (2014). Fundamentals and application of magnetic particles in cell isolation and enrichment: a review. Rep Prog Phys 78:016601. doi:10.1088/0034-4885/78/1/016601.
- Bonnemay L, Hoffmann C, Gueroui Z. (2014). Remote control of signaling pathways using magnetic nanoparticles. Wiley Interdiscip Rev Nanomed Nanobiotechnol 3:342–54.
- Dobson J. (2006). Gene therapy progress and prospects: magnetic nanoparticle-based gene delivery. Gene Ther 13:283–7.
- Corot C, Robert P, Idée JM, Port M. (2006). Recent advances in iron oxide nanocrystal technology for medical imaging. Adv Drug Deliv Rev 58:1471–504.
- Yang F, Li Y, Chen Z, et al. (2009). Superparamagnetic iron oxide nanoparticle-embedded encapsulated microbubbles as dual contrast agents of magnetic resonance and ultrasound imaging. Biomaterials 30:3882–90.
- Yang L, Cao Z, Sajja HK, et al. (2008). Development of receptor targeted magnetic iron oxide nanoparticles for efficient drug delivery and tumor imaging. J Biomed Nanotechnol 4:439–49.
- Estelrich J, Escribano E, Queralt J, Busquets MA. (2015). Iron oxide nanoparticles for magnetically-guided and magnetically-responsive drug delivery. Int J Mol Sci 16:8070–101.
- Liu Y, Gao Y, Xu C. (2013). Using magnetic nanoparticles to manipulate biological objects. Chin Phys B 22:097503.
- Périgo EA, Hemery G, Sandre O, et al. (2015). Fundamentals and advances in magnetic hyperthermia. Appl Phys Rev 2:041302.
- Dutz S, Hergt R. (2014). Magnetic particle hyperthermia-a promising tumour therapy? Nanotechnology 25:452001.
- Gilchrist RK, Medal R, Shorey WD, et al. (1957). Selective inductive heating of lymph nodes. Ann Surg 146:596–606.
- Jordan A, Scholz R, Maier-Hauff K, et al. (2001). Presentation of a new magnetic field therapy system for the treatment of human solid tumors with magnetic fluid hyperthermia. J Magn Magn Mater 225:118–26.
- Kozissnik B, Bohorquez AC, Dobson J, Rinaldi C. (2013). Magnetic fluid hyperthermia: advances, challenges, and opportunity. Int J Hyperthermia 29:706–14.
- Hilger I. (2013). In vivo applications of magnetic nanoparticle hyperthermia. Int J Hyperthermia 29:828–34.
- Grüttner C, Müller K, Teller J, Westphal F. (2013). Synthesis and functionalisation of magnetic nanoparticles for hyperthermia applications. Int J Hyperthermia 29:777–89.
- Ito A, Shinkai M, Honda H, Kobayashi T. (2005). Medical application of functionalized magnetic nanoparticles. J Biosci Bioeng 100: 1–11.
- Balivada S, Rachakatla RS, Wang H, et al. (2010). A/C magnetic hyperthermia of melanoma mediated by iron(0)/iron oxide core/shell magnetic nanoparticles: a mouse study. BMC Cancer 10:119–27.
- Gneveckow U, Jordan A, Scholz R, et al. (2004). Description and characterization of the novel hyperthermia- and thermoablation-system MFH 300F for clinical magnetic fluid hyperthermia. Med Phys 31:1444–51.
- Johannsen M, Thiesen B, Wust P, Jordan A. (2010). Magnetic nanoparticle hyperthermia for prostate cancer. Int J Hyperthermia 26:790–5.
- Kansara M, Teng MW, Smyth MJ, Thomas DM. (2014). Translational biology of osteosarcoma. Nat Rev Cancer 14:722–35.
- Hogendoorn PC, Athanasou N, Bielack S, et al. ESMO/EUROBONET Working Group. (2010). Bone sarcomas: ESMO clinical practice guidelines for diagnosis, treatment and follow-up. Ann Oncol 21:204–13.
- Rodan SB, Imai Y, Thiede MA, et al. (1987). Characterization of a human osteosarcoma cell line (SaOS-2) with osteoblastic properties. Cancer Res 47:4961–6.
- Zebisch K, Voigt V, Wabitsch M, Brandsch M. (2012). Protocol for effective differentiation of 3T3-L1 cells to adipocytes. Anal Biochem 425:88–90.
- Blau HM, Pavlath GK, Hardeman EC, et al. (1985). Plasticity of the differentiated state. Science 230:758–66.
- Simeonidis K, Liébana Viñas S, Wiedwald U. (2016). A versatile large-scale and green process for synthesizing magnetic nanoparticles with tunable magnetic hyperthermia features. RSC Adv 6: 53107–17.
- Lee JH, Jang JT, Choi JS, et al. (2011). Exchange-coupled magnetic nanoparticles for efficient heat induction. Nat Nanotechnol 6:418–22.
- Angelakeris M, Li ZA, Hilgendorff M, et al. (2015). Enhanced biomedical heat-triggered carriers via nanomagnetism tuning in ferrite-based nanoparticles. J Magn Magn Mater 381:179–87.
- Liébana Viñas S, Simeonidis K, Li Z-A, et al. (2016). Tuning the magnetism of ferrite nanoparticles. J Magn Magn Mater 415:20–3.
- Sharifi I, Shokrollahi H, Amiri S. (2012). Ferrite-based magnetic nanofluids used in hyperthermia applications. J Magn Magn Mater 324:903–15.
- Bellizzi G, Bucci OM, Chirico G. (2016). Numerical assessment of a criterion for the optimal choice of the operative conditions in magnetic nanoparticle hyperthermia on a realistic model of the human head. Int J Hyperthermia 7. doi:10.3109/02656736.2016.1167258.
- Sapareto SA. (1987). Thermal isoeffect dose: addressing the problem of thermotolerance. Int J Hyperthermia 3:297–305.
- Oleson JR, Samulski TV, Leopold KA, et al. (1993). Sensitivity of hyperthermia trial outcomes to temperature and time: Implications for thermal goals of treatment. Int J Radiat Oncol Biol Phys 25: 289–97.
- Yarmolenko PS, Moon EJ, Landon C, et al. (2011). Thresholds for thermal damage to normal tissues: an update. Int J Hyperthermia 26:1–26.
- Van Rhoon GC, Samaras T, Yarmolenko PS, et al. (2013). CEM43 °C thermal dose thresholds: A potential guide for magnetic resonance radiofrequency exposure levels? Eur Radiol 23:2215–27.
- Dewhirst MW, Abrahamx J, Viglianti B. (2015). Evolution of thermal dosimetry for application of hyperthermia to treat cancer. Chapter 6. Adv Heat Transfer 47:397–421.
- Lei L, Ling-Ling J, Yun Z, Gang L. (2013). Toxicity of superparamagnetic iron oxide nanoparticles: research strategies and implications for nanomedicine. Chin Phys B 22:1–10.
- Chung DM, Kim JH, Kim JK. (2015). Evaluation of MTT and trypan blue assays for radiation-induced cell viability test in HepG2 cells. Int J Radiat Res 13:331–5.
- Makridis A, Topouridou K, Tziomaki M, et al. (2014). In vitro application of Mn-ferrite nanoparticles as novel magnetic hyperthermia agents. J Mater Chem B Mater Biol Med 2:8390–8.
- Bogart LK, Taylor A, Cesbron Y, et al. (2012). Photothermal microscopy of the core of dextran-coated iron oxide nanoparticles during cell uptake. ACS Nano 6:5961–71.
- Herman TS, Gerner EW, Magun BE, et al. (1981). Rate of heating as a determinant of hyperthermic cytotoxicity. Cancer Res 41:3519–23.
- Gerner EW. (1987). Thermal dose and time-temperature factors for biological responses to heat shock. Int J Hyperthermia 3: 319–27.
- Mallory M, Gogineni E, Jones GC, et al. (2016). Therapeutic hyperthermia: The old, the new, and the upcoming. Crit Rev Oncol Hematol 97:56–64.
- Tang Y, McGoron AJ. (2013). Increasing the rate of heating: a potential therapeutic approach for achieving synergistic tumour killing in combined hyperthermia and chemotherapy. Int J Hyperthermia 29:145–55.
- Alvarez-Berríos MP, Castillo A, Mendéz J, et al. (2013). Hyperthermic potentiation of cisplatin by magnetic nanoparticle heaters is correlated with an increase in cell membrane fluidity. Int J Nanomed 8:1003.
- Urban AS, Fedoruk M, Horton MR, et al. (2009). Controlled nanometric phase transitions of phospholipid membranes by plasmonic heating of single gold nanoparticles. Nano Lett 9:2903–8.
- Sundararajan R, Jason H, Funian X, et al. (2014). Electroporation-based therapies for cancer. Cambridge, UK: Elsevier.
- Blanco-Andujar C, Ortega D, Southern P, et al. (2016). Real-time tracking of delayed-onset cellular apoptosis induced by intracellular magnetic hyperthermia. Nanomedicine (Lond) 11:121–36.