ABSTRACT
Exact simulations of engineering practice are often difficult to accomplish with test methods of materials performance against hydrogen embrittlement (HE). Instead, it is proposed that assessment of the intrinsic susceptibility to HE be adaptable to diverse usage environments and loading modes. This notion is based on recent findings concerning the predominant role of strain-induced vacancies and their involvement in characteristic features of HE such as microstructural effects and dependence on strain rates and temperatures. The function of hydrogen in enhancing the generation of strain-induced vacancies operates throughout the entire process of fracture, and the density of vacancies is detectable using hydrogen as a tracer. A method is proposed here for using as the parameter the difference in the amounts of tracer-hydrogen between specimens given cyclic stressing with and without hydrogen.
Introduction
Proper selection and design of materials against hydrogen embrittlement (HE) have been key issues in recent developments pertinent to hydrogen energy technologies [Citation1–3]. HE of steels is manifested in diverse ways, such as a loss of ductility, reduction of load-bearing capacity and unexpected cracking, according to materials, loading modes and environments [Citation4]. The susceptibility to HE is evaluated in terms of various quantities pertinent to test methods employed. Materials for structural components must meet performance qualifications for engineering use. Fracture toughness under high-pressure gaseous hydrogen, for example, is evaluated in terms of the critical stress intensity factor, KIH, or J-integral, JIH, for the onset of fracture by simulating stress fields ahead of a crack tip predicted to occur under service conditions [Citation5–7]. Exact simulations, however, are often difficult. Delayed fracture under sustained loading is typical of HE in structural components of high-strength steel, but laboratory methods to assess the susceptibility of steels have not been established yet. The need to accelerate failure that actually takes place in a long-term service, often extending over years under atmospheric exposure [Citation8], is the main reason for the difficulties in laboratory tests, in addition to unspecified environments and stress states that materials have to tolerate in practice.
Embrittlement caused by hydrogen of even less than 1 mass ppm has characteristic features and the mechanism of HE has been widely discussed with respect to the role of hydrogen in both brittle and plasticity-related fracture models [Citation9–13]. Test methods for the susceptibility of materials to HE should be either empirically established or supported by the valid mechanism, but diverse manifestation of HE complicates a unified understanding of the mechanism involved. Conversely, test methods based on a presumed mechanism would correctly assess HE susceptibility if the postulated mechanism is valid. Application to materials selection and assessment will also be a necessary task for validation of a mechanism model. Recently, substantial advances in experimental techniques have unveiled the states of hydrogen in materials and microscopic details of the HE process [Citation14–18]. A consensus about the mechanism of HE has not been established yet, but recent findings suggest prospects of proper methods for the design of steels and assessment of their performance.
Test methods for the susceptibility to HE of materials can be classified roughly into two types: one is to evaluate directly the performance of materials under conditions close to practical service and the other is to assess the intrinsic susceptibility proper to each material under unspecified conditions. Here, the response of materials to applied stress under hydrogen environments leading to premature fracture is defined as the intrinsic susceptibility to HE. The former type of tests must be finally applied for qualification, but the latter is viable for the design of materials of wide usage. Examples of the latter are very few.
The crucial involvement of plasticity in HE has been well shown in fracture mechanics tests [Citation19], fracture morphologies [Citation20] and deformation microstructures beneath the fracture surface [Citation14]. The key issue in the mechanism of HE is the function of hydrogen in plasticity leading to premature fracture. The primary players in plasticity are dislocations and the role of dislocations is to be considered with respect to the process of premature fracture. Effects of hydrogen have been discussed mostly with respect to interactions with dislocations, but the movement of dislocations, resulting in their increased density, gives rise to not only displacement fields but also various point and planar defects by the mutual interactions of dislocations. It has been revealed experimentally and theoretically that interactions between hydrogen and vacancies decrease the formation energy [Citation21] and diffusivity [Citation22] of vacancies and stabilise a high density of strain-induced vacancies leading to the formation, growth and linking of vacancy clusters [Citation3,Citation17,Citation18]. The hydrogen-enhanced generation of strain-induced vacancies (HESIV) mechanism, a comprehensive presentation of which is in an overview paper [Citation18], shifts the essential player in the premature fracture in HE from hydrogen or dislocations to the promoted generation of damage. A view of the HESIV mechanism through the entire process of fracture suggests its application to the assessment of the susceptibility of steels under various environments and loading modes.
This paper is organised as follows: first, test methods of delayed fracture using the concept of the critical hydrogen concentration are critically reviewed. Recent findings concerning the predominant role of strain-induced vacancies and their involvement in characteristic features of HE are then summarised. After demonstrating the accumulation of vacancy-type defects in the entire process of fracture, a method to assess the intrinsic susceptibility to HE is proposed in terms of a parameter that represents the HESIV mechanism.
Limitations of the concept of critical hydrogen concentration
The concept of the critical hydrogen concentration, HC, may be an example of an expression of intrinsic susceptibility. It was applied to assess the susceptibility of steels to delayed fracture [Citation23,Citation24]. Considering that the hydrogen concentration is decisive in delayed fracture, HC was experimentally defined as the permissible content of hydrogen without causing failure for up to, say, 100 h at room temperature as schematically shown in Figure . The hydrogen content was measured by means of thermal desorption analysis (TDA) that detected hydrogen on a temperature ramp of specimen. TDA profiles in experiments by Suzuki et al. [Citation23] exhibited two desorption peaks and the amount of the lower temperature peak was employed as meaningful in terms of diffusible hydrogen. The peak higher than 550 K is likely background hydrogen desorbed from the apparatus. Hydrogen was introduced into notched specimens prior to sustained-loading fracture tests. In later papers, the observed hydrogen concentration was converted to the calculated local hydrogen concentration HC* at the site of maximum tensile stress or in the process zone ahead of the prenotch [Citation25,Citation26]. The magnitude of HC* of a steel is independent of the initial hydrogen content, but varies according to the specimen geometry and applied stress [Citation27]. A procedure to determine HC has been included in the ISO standards as a technique for evaluating the resistance of high-strength steels against HE [Citation28].
Figure 1. Schematic illustration of the method to determine the critical hydrogen concentration in sustained loading delayed fracture.
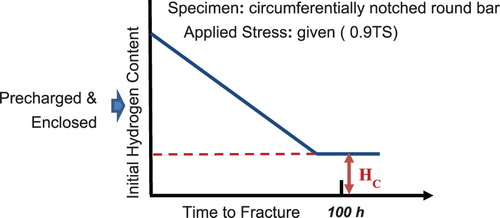
It should be noticed that the magnitude of HC or HC* does not by itself indicate superiority or inferiority of materials against HE. In service, hydrogen enters materials from the usage environment and the safety criterion is that hydrogen actually present in the test specimen should be less than Hc as expressed HC > HE or locally HC* > HE*, where HE is the amount of environmentally absorbed hydrogen. ISO standard 16573 [Citation28] disregards a comparison of HC with HE, which is crucial for the criterion of delayed fracture. Yamazaki et al. used HE experimentally obtained by a laboratory corrosion test [Citation24]. A good correlation was found between HE/HC and the failure ratios of steel fasteners exposed in an atmospheric environment for 12 months, but not for HC or (HC – HE), contrary to expectations. Another long-term atmospheric exposure test of fasteners showed a strong regional dependence of the fracture ratio [Citation8]. That finding indicates that HE must be adjusted according to the actual usage situation. In the study done by Yamazaki et al. [Citation24], the steels used were of different strengths and the order of the failure ratios among them was also coincident with that of their tensile strengths.
The concept of HC was originally proposed in a brittle fracture model of HE assuming a reduction of cohesive strength by hydrogen to a critical level [Citation29]. The state of hydrogen in the model was in solid solution. The model, however, required unrealistically high local stress and hydrogen concentration in order to fit the observed failure. The notion is convenient and intuitively tractable, and many studies have implicitly postulated it without a vital discussion about microscopic processes to operate in cracking. The concept might be useful as a measure of the susceptibility to HE in cases where the supply of hydrogen is rate-determining in the failure process. However, a steady increase in the hydrogen content corresponding to the occurrence of failure has never been observed in delayed fracture tests of steels under long-term atmospheric exposure [Citation30]. More definite evidence of the supplementary function of hydrogen in HE is a premature fracture in tensile tests even without the presence of hydrogen in the final stage [Citation31].
The method of determining HC has some crucial difficulties with regard to the function of hydrogen in HE. Hydrogen in materials occupies various sites of different binding energies and it functions differently in embrittlement depending on the site. Trapping behaviours of hydrogen change during service and precharged hydrogen is not always sufficient to follow the change. Phenomenologically determined HC or HC* under a given stress in short-term laboratory tests at room temperature might be utilised for quality control of a product, but would not be a direct guide for materials design or as a criterion for assessing performance in unspecified situations.
Density of strain-induced vacancies characterising HE
A parameter is requisite to express the intrinsic susceptibility to HE. In this section, it is shown that characteristics of HE of steels such as the dependence on materials and test methods are relevant to the strain-induced generation of vacancies. Effects of hydrogen in the generation of vacancies are expressed in terms of a parameter common to various materials and test methods.
Microstructural effects
HE of steels is influenced by compositions and microstructures, but independent control of each structural factor is usually difficult. If a common function of hydrogen operates in various microstructural effects on HE, parameters that represent such a function would be useful for the design of materials and assessment of their performance.
Tempering of martensite: internal strain
Tempered martensite is the most common microstructure of high-strength steels. Mechanical properties are controlled by tempering that stabilises substructures and releases internal strain. Figure (a) shows the critical stress intensity, KISCC, below which cracking does not occur in delayed fracture tests in a 3% NaCl aqueous solution of 0.35C–Cr–Mo steels additionally alloyed with (A) 0.04Nb, (B) 0.04Ti + 0.10V or (C) 0.10V + 0.04Nb (mass%) to the base compositions [Citation32]. The effects of the tempering temperature on KISCC are grouped by tensile strength levels. Lower tensile strengths obtained by higher tempering temperatures generally improve KISCC, i.e. reduce the susceptibility to HE. However, strength is not the sole outcome produced by tempering. The increase in KISCC by tempering at higher temperatures appears even at a constant strength level. Stabilisation of substructures by tempering reduces internal strain as observed by a linear decrease in the broadening of the X-ray diffraction line width [Citation32]. Figure (b) [Citation32] shows good correspondences between KISCC and internal strain estimated from the half-width of the X-ray diffraction line for the steels used in Figure (a).
Figure 2. Dependence of KISCC on (a) tempering temperature and (b) internal strain estimated from X-ray line broadening for medium carbon Cr–Mo martensitic steels. (A) 0.04Nb, (B) 0.04Ti + 0.10V or (C) 0.10V + 0.04Nb (mass%) added to the base compositions [Citation32]. (The numerical values in the abscissa of Figure (b) may not be correct.)
![Figure 2. Dependence of KISCC on (a) tempering temperature and (b) internal strain estimated from X-ray line broadening for medium carbon Cr–Mo martensitic steels. (A) 0.04Nb, (B) 0.04Ti + 0.10V or (C) 0.10V + 0.04Nb (mass%) added to the base compositions [Citation32]. (The numerical values in the abscissa of Figure 1(b) may not be correct.)](/cms/asset/45e9d917-b409-43d9-93fc-b89a90ec9c9a/ymst_a_1639009_f0002_ob.jpg)
The results strongly suggest that internal strain or associated dislocation configuration is a crucial controlling factor of the susceptibility of martensitic steels to HE. In that study, specimens were subjected to X-ray measurement before loading. Broadening of the X-ray diffraction line was also reported for intergranular (IG) fracture surfaces of hydrogen-charged martensitic steels [Citation33]. Doping of P enhanced the susceptibility to H of the steel that exhibited smoother IG surface with less traces of plasticity. However, the {110} X-ray line on the IG surface was broader than that from the rough surface of the P-undoped steel. The apparent contradiction implies that extreme strain localisation close to prior austenite grain boundaries causes intense accumulation of damage there leading to the premature fracture in very narrow areas along boundaries.
Grain size effect: strain-induced vacancies
Grain size refinement is a method that is effectively and commonly used to improve the fracture toughness of steels, and the origin of grain size effects is vital for understanding the mechanism of HE. The term ‘grain size’ for martensitic steels means here prior austenite grain size. Trapping of hydrogen can be utilised as a tool of detecting lattice defects. Instead of functioning as an embrittlement agent, hydrogen introduced for detecting defects [Citation34] is termed tracer-hydrogen.
Grain refinement of high-strength martensitic steels in the range of 25–4 μm by repeated induction-heating and quenching substantially reduced the susceptibility to HE evaluated in terms of fracture stress and fracture strain [Citation35]. The amount of tracer-hydrogen in as-heat-treated specimens increased associated with the increase in grain boundary areas, and plastic prestrain markedly enhanced the increase consistent with previous results [Citation34,Citation36]. However, the total amounts of tracer-hydrogen in strained specimens were rather reduced by grain refinement, and the increments by straining correlated well with the hydrogen degradation. Subsequent annealing of strained specimens at 523 K almost totally eliminated the strain-induced increase in tracer-hydrogen. The results indicate that the reduced susceptibility to HE by grain refinement stems from the reduction of strain-induced generation of vacancies. Another important implication of the experiment is that only a part of the total hydrogen is pertinent to degradation, consistent with previous results for 0.8% C steels of eutectoid and lower bainitic microstructures [Citation37]. Strongly trapped and non-diffusive hydrogen at room temperature was immune to degradation.
Compositional effect: hydrogen effects on strain-induced vacancies
Effects of steel compositions on HE result from deformation microstructures that develop in various ways. Tensile properties of 0.3% C martensitic steels were immune to the Mn contents when tested without hydrogen, but an increase in the Mn content from 0.5 to 1.5% substantially reduced fracture stress and strain under concurrent cathodic hydrogen-charging [Citation36]. Correspondingly, the amounts of tracer-hydrogen were initially immune to the Mn content but increased with increasing Mn content in specimens given plastic strain. Annealing of strained specimens at 523 K for 1 h after straining eliminated the appearance of the increase in tracer-hydrogen, indicating the vacancy-type nature of strain-induced defects. The generation of vacancies is very likely enhanced when hydrogen is present at the time of tensile testing, substantially reducing fracture strain.
The tensile-fracture surface of specimens under cathodic hydrogen-charging exhibited smoother IG fracture with reduced fine tear patterns for specimens of higher Mn content. The apparent contradiction between increased strain-induced defects as revealed by TDA and reduced plasticity on IG fracture surfaces is consistent with a previous observation for P-doped martensitic steel [Citation33]. The TDA results and fractographic features are consistent if strong strain localisation takes place in very narrow regions along prior austenite grain boundaries. Enhancement of IG fracture by Mn was noticed in temper embrittlement of martensitic steels [Citation38]. The microstructural nature of Mn was not definite, but it is feasible that constraint of slip extension across grain boundaries activates different slip systems and generates very locally high densities of vacancies by mutual interactions of dislocations.
Effects of test conditions: hydrogen-enhancement of strain-induced vacancies
A characteristic of HE of steels is strong dependence on the strain-rate and test-temperature [Citation39]. The traditional understanding has been the rate of buildup through diffusion of local hydrogen concentration to the critical level. However, in the context of the preceding sections, some factors common to microstructural effects are expected to be operating.
The binding energy with hydrogen differs by the type of lattice defects, and the TDA profile of tracer-hydrogen is useful in discriminating defects. TDA of hydrogen in cold-drawn eutectoid steels exhibits separate diffusive and non-diffusive peaks at around 373 K (Peak#1) and 620 K (Peak#2), respectively, and the latter hydrogen does not influence HE [Citation40]. The origin of the strain-rate dependence of HE in tensile tests was investigated for cold-drawn eutectoid steels prepared to contain diffusive or selectively only non-diffusive hydrogen [Citation41]. The latter specimens containing only Peak#2 hydrogen were prepared by heating them to 473 K after hydrogen charging to eliminate Peak#1. Figure (a) [Citation41] shows the strain-rate dependence of HE in terms of fracture strain for the two types of specimens. The strain-rate dependence did not appear in specimens that contained only Peak #2 hydrogen. Defects interacting with hydrogen were then detected using tracer-hydrogen introduced after straining. The difference in the amount of Peak#1 tracer-hydrogen between specimens strained to 0.08 with/without hydrogen was denoted as ΔCH as a measure of the HESIV mechanism. An increase in ΔCH with decreasing strain rate is shown in Figure (b) [Citation41]. The strain-rate dependence appeared in specimens containing Peak#1 hydrogen and higher degradation was associated with higher ΔCH.
Figure 3. Strain-rate dependencies of (a) relative reduction in area in tensile tests for specimens containing [Peak#1 + Peak#2] hydrogen and only [Peak#2] hydrogen, and (b) difference in Peak#1 hydrogen, ΔCH, between specimens strained to 0.08 with and without hydrogen for [Peak#1 + Peak#2] and [Peak#2] series [Citation41].
![Figure 3. Strain-rate dependencies of (a) relative reduction in area in tensile tests for specimens containing [Peak#1 + Peak#2] hydrogen and only [Peak#2] hydrogen, and (b) difference in Peak#1 hydrogen, ΔCH, between specimens strained to 0.08 with and without hydrogen for [Peak#1 + Peak#2] and [Peak#2] series [Citation41].](/cms/asset/fd7f222b-f6d4-466b-b128-10de0403652d/ymst_a_1639009_f0003_ob.jpg)
A similar correlation between hydrogen degradation and ΔCH was also found along with temperature dependence in specimens containing Peak#1 hydrogen as shown in Figure [Citation41]. Specimens containing only Peak#2 hydrogen were almost immune to both strain rate and temperature, and their ΔCH was practically zero. Similar correlations between strain-rate dependencies of fracture strain and ΔCH were observed for X 80 pipeline steel of ferrite and bainite microstructures [Citation42]. The fracture morphology observed in tensile tests in the presence of hydrogen was fine and shallow dimples.
Figure 4. Temperature dependencies of (a) relative reduction in area in tensile tests for specimens containing [Peak#1 + Peak#2] hydrogen and only [Peak#2] hydrogen, and (b) difference in Peak#1 hydrogen, ΔCH, between specimens strained to 0.08 with and without hydrogen for [Peak#1 + Peak#2] and [Peak#2] series [Citation41].
![Figure 4. Temperature dependencies of (a) relative reduction in area in tensile tests for specimens containing [Peak#1 + Peak#2] hydrogen and only [Peak#2] hydrogen, and (b) difference in Peak#1 hydrogen, ΔCH, between specimens strained to 0.08 with and without hydrogen for [Peak#1 + Peak#2] and [Peak#2] series [Citation41].](/cms/asset/07d7d339-1c6a-408b-94f1-bc93630297e4/ymst_a_1639009_f0004_ob.jpg)
Versatility of strain-induced vacancies in embrittlement: effects of stress and environmental histories
The intrinsic susceptibility to HE is to be assessed without using fracture tests. In this section, it is shown that stress and environmental histories affect HE of specimen. The HESIV mechanism operates throughout the entire process of fracture regardless of loading modes.
Structural components tolerate various stress and environmental changes during service. A crucial issue for the assessment of materials against HE is the carry-over of damage from stages preceding the final failure. This issue concerns the function of hydrogen throughout the entire process of fracture and the accumulation of damage induced by hydrogen prior to the final fracture. Carry-over of fatigue damage to subsequent sustained-loading delayed-fracture tests was exhibited as the reduction of time to failure for specimens given fatigue cycles close to the fatigue life [Citation43]. Degradation in the delayed fracture test almost disappeared when fatigued specimens were annealed at temperatures as low as 473 K for 1 h. This result indicates that damage generated by fatigue cycles plays a role in delayed fracture and that the nature of the damage is mostly vacancies reversible on annealing without any formation of flaws such as voids and cracks.
Another example is tensile tests of high-strength martensitic steel subjected to sustained loading under a corrosive environment prior to tensile tests [Citation44]. Sustained loading was conducted under stress of 80% of the tensile strength, macroscopically within the elastic range, in a 20% NH4SCN aqueous solution for 96 h at 323 K. Some specimens were annealed at 473 K for 2 h after sustained loading so as to annihilate strain-induced damage generated by sustained loading. Tensile tests were then conducted in air after removing hydrogen at 300 K. The experiment was intended to demonstrate the crucial role of accumulated damage in HE rather than that of hydrogen itself. Figure [Citation44] shows tensile curves of three treatments. The prior sustained loading substantially reduced fracture strain, but successive annealing at 473 K almost completely eliminated degradation. Sustained loading under a corrosive environment most likely introduces hydrogen that enhances the local generation of vacancies while the applied stress is apparently within the elastic range. Thus vacancies generated without forming irreversible flaws probably operate in successive tensile tests and reduce the level of strain to fracture. Promotion of failure without the presence of hydrogen in the final stage is consistent with previous results of tensile tests with interposed unloading and reloading for iron and Inconel 625 [Citation31].
Figure 5. Tensile curves of high-strength steels given sustained loading at 0.8 of the tensile strength for 96 h with hydrogen charging. Tensile tests were conducted after degassing hydrogen at 303 K for 168 h. Tensile curves of specimens annealed at 473 K for 2 h after [H + prestress + 200°C] or without [Non-prestressin + 200°C] prestressing are also shown for comparison [Citation44].
![Figure 5. Tensile curves of high-strength steels given sustained loading at 0.8 of the tensile strength for 96 h with hydrogen charging. Tensile tests were conducted after degassing hydrogen at 303 K for 168 h. Tensile curves of specimens annealed at 473 K for 2 h after [H + prestress + 200°C] or without [Non-prestressin + 200°C] prestressing are also shown for comparison [Citation44].](/cms/asset/0bbc6cb3-bd9e-498f-acd3-2d5266d685b0/ymst_a_1639009_f0005_ob.jpg)
Parameter as a measure of the intrinsic susceptibility to HE
The common function of hydrogen in different loading modes implies the possibility of a versatile parameter that represents the intrinsic susceptibility of materials to HE. A requisite for laboratory tests to assess the long-term performance of material is to accelerate failure without deteriorating essential functions of hydrogen. In previous studies, it was revealed that alternations of applied stress [Citation45,Citation46] and environment [Citation47] promoted failure in sustained-loading delayed fracture tests of high-strength steels. The mechanism is not yet definite, but induced alternations of dislocation configurations likely activate mutual interactions of dislocations as a source of vacancies.
The results, coupled with the ones described in preceding sections, put forward a method incorporating characteristics of HE. A model experiment worthy in this context was the application of cyclic stressing prior to the tensile test of a high-strength steel as schematically shown in Figure [Citation48]. Cyclic stressing was conducted with different strain rates, while the tensile test was performed at a constant strain rate. The tests were also conducted with and without hydrogen precharging. Hydrogen degradation of the final tensile properties was denoted as HE susceptibility, HES, defined as the ratio of the fracture strain of specimens with and without hydrogen, εfH/εf. Degradation in the final tensile test was more prominent with lower strain rates and increasing numbers of stress cycles.
Figure 6. Tensile test after cyclic prestressing. (a) Schematic illustration of cyclic prestressing of different strain rates with/without hydrogen charging. Tensile test was conducted at constant strain rate. (b) Degradation of fracture strain at the tensile test affected by cyclic prestressing under hydrogen precharging [Citation48].
![Figure 6. Tensile test after cyclic prestressing. (a) Schematic illustration of cyclic prestressing of different strain rates with/without hydrogen charging. Tensile test was conducted at constant strain rate. (b) Degradation of fracture strain at the tensile test affected by cyclic prestressing under hydrogen precharging [Citation48].](/cms/asset/3496fb77-2a7b-4b56-a229-92094f8b3f19/ymst_a_1639009_f0006_ob.jpg)
The nature of damage generated at the prestressing stage was examined using tracer-hydrogen introduced into cyclically stressed specimens after degassing the initial hydrogen. The effect of hydrogen was expressed in terms of the difference in the amounts of tracer-hydrogen, ΔCH, between specimens cyclically stressed with and without hydrogen. The magnitude of ΔCH increased with stress cycles more pronouncedly with lower strain rates as shown in Figure (a) [Citation48]. The increase in ΔCH in cyclic prestressing correlates well with the degradation in the tensile tests, HES, as shown in Figure (b) [Citation48]. The correlation is more explicit at lower strain rates that characterise HE. The fairly good correlation implies that detecting hydrogen enhancement of the generation of strain-induced defects, most probably vacancies, under well-controlled test conditions is useful in assessing the susceptibility of materials to HE.
Figure 7. (a) Hydrogen enhancement of the generation of defects on cyclic stressing with increasing stress cycles at different strain rates. (b) Relationship between HES and ΔCH at different strain rates at prestressing [Citation48].
![Figure 7. (a) Hydrogen enhancement of the generation of defects on cyclic stressing with increasing stress cycles at different strain rates. (b) Relationship between HES and ΔCH at different strain rates at prestressing [Citation48].](/cms/asset/ae52b5fc-43c8-4392-8dec-9b0bdb4299ea/ymst_a_1639009_f0007_ob.jpg)
ΔCH represents the effect of hydrogen on the evolution of damage caused by plastic straining, and the method here to define ΔCH incorporates the effects of cyclic stressing and strain rate that characterise HE of steels. The method is not a direct evaluation of the fracture performance of materials, but it is a method for quantifying the deterioration of materials caused by applied stress under hydrogen environments, i.e. the intrinsic susceptibility to HE. In this sense, the concept of ΔCH is adaptable to various environments and loading modes. ΔCH obtained under a proper condition may be functional for the relative ranking of steels against HE. However, information obtained by TDA is averaged over an entire specimen. Since the final fracture is a local event, ΔCH must be determined in situations where the local evolution of strain characterises the plastic deformation of materials. Microstructural effects on ΔCH are surely expected according to previous results [Citation35,Citation36], but extensive examinations must be made of the correspondence of ΔCH with the actual performance of steels.
Discussion and conclusions
Valid simulations of engineering practice are often difficult to accomplish in laboratory tests of HE of steels. Diverse manifestations of HE also make difficult evaluation of material performance in a single test. Accordingly, assessment of the intrinsic susceptibility of materials to HE will be functional for the design and qualification of materials against HE. A method in terms of the critical hydrogen concentration for delayed fracture might be an attempt of a test procedure, but it is not viable in actual practice.
Substantial evidence has been obtained concerning the operation of the HESIV mechanism and its involvement in characteristics of HE such as microstructural, strain-rate and temperature effects. Recent molecular dynamics simulations have depicted dislocation emission from nanovoids and associated void growth leading to the nanoscale multiple void coalescence process [Citation49–52]. The results are consistent with fractographic features of HE such as refined dimples, quasi-cleavage and decorated intergranular fracture surfaces. The simulations intimate that evolutions of high densities of vacancies and dislocation are cooperation or auto-catalytic in strain localisation. Distinction between hydrogen-enhanced localised plasticity (HELP) [Citation14,Citation15] and HESIV is often difficult, but the initiative will be attributable to the HESIV mechanism in the presence of hydrogen because of the higher binding energies of hydrogen with vacancies than dislocations [Citation53,Citation54]. TDA of tracer-hydrogen introduced under a low hydrogen fugacity to a ferritic steel given plastic strain also supports the notion [Citation34].
The HESIV mechanism operates over the entire process of fracture and the generated damage is carried over to later stages. Susceptibility to HE is detectable without conducting fracture tests. From the viewpoint that enhancement of damage is an essential factor of embrittlement, the difference in the amounts of tracer-hydrogen, ΔCH, between specimens cyclically stressed with/without hydrogen has been proposed as a parameter to represent the intrinsic susceptibility of steels to HE.
The proposed method incorporates characteristics features of HE and is likely adaptable to various loading modes and environments. It is also handy and excludes a large scatter of values often encountered in fracture tests. ΔCH is not a direct measure of material performance in service, but it will be of use for relative ranking of materials. However, it has not been extensively examined yet. It is hoped that the findings and notions presented in this paper stimulate studies to establish valid and practical test methods.
Disclosure statement
No potential conflict of interest was reported by the authors.
Correction Statement
This article has been republished with minor changes. These changes do not impact the academic content of the article.
References
- Briottet L, Moro I, Solin J, et al. Research on fatigue of Cr-Mo steel for hydrogen storage vessels. In: Somerday BP, Sofronis P, editors. Materials performance in hydrogen environments. New York (NY): ASME; 2017. p. 244–253.
- Saxena A, Prakash A, Nibur KA, et al. Considerations of the effects of H2 in the design of type II storage vessels built for fatigue resistance. In: Somerday BP, Sofronis P, editors. Materials performance in hydrogen environments. New York (NY): ASME; 2017. p. 382–389.
- Neeraj T. Hydrogen embrittlement and its impact on oil and gas industry. In: Somerday BP, Sofronis P, editors. Materials performance in hydrogen environments. New York (NY): ASME; 2017. p. 45–53.
- Nagumo M. Fundamentals of hydrogen embrittlement. Singapore: Springer Nature; 2016; Chapter 6, Manifestations of hydrogen embrittlement; p. 103–135.
- ASME Article KD-10. Special requirements for vessels in high pressure gaseous hydrogen transport and storage service, 2007.
- ANSI/CSA CHMC1-2014. Test methods for evaluating material compatibility in compressed hydrogen applications – metals, 2014.
- International Organization for Standardization (ISO). Transportable gas cylinders – compatibility of cylinder and valve materials with gas contents, part 4: test methods for selecting metallic materials resistant to hydrogen embrittlement. ISO; 2005. ISO11114-4.
- Matsuoka K, Uno N, Akiyama W, et al. Stochastic evaluation of hydrogen uptake affecting the delayed fracture of high strength Bolts. Steel Construct Eng. 2013;20:29–40. Japanese.
- Nagao A, Dadfarnia M, Sofronis P, et al. Hydrogen embrittlement: mechanism. In: Colás R, Totten GE, editors. Encyclopedia of iron, steel, and their alloy. New York (NY): Taylor & Francis; 2016. p. 1768–1784.
- Nagumo M. Hydrogen embrittlement: theories. In: Colás R, Totten GE, editors. Encyclopedia of iron, steel, and their alloy. New York (NY): Taylor & Francis; 2016. p. 1785–1800.
- Barrera S, Bombac D, Chen Y, et al. Understanding and mitigating hydrogen embrittlement of steels: a review of experimental, modelling and design progress from atomistic to continuum. J Mater Sci. 2018;53:6251–6290. doi: 10.1007/s10853-017-1978-5
- Pundt A, Kirchheim R. Hydrogen in metals: microstructural aspects. Annu Rev Mater Res. 2006;36:555–608. doi: 10.1146/annurev.matsci.36.090804.094451
- Lynch SP. Hydrogen embrittlement phenomena and mechanisms. Corros Rev. 2012;30:105–123.
- Robertson IM, Sofronis P, Nagao A, et al. Hydrogen embrittlement understood. Metall Mater Trans A. 2015;46A:2323–2341. doi: 10.1007/s11661-015-2836-1
- Martin ML, Dadfarnia M, Nagao A, et al. Enumeration of the hydrogen-enhanced localized plasticity mechanism for hydrogen embrittlement in structural materials. Acta Mater. 2019;165:734–750. doi: 10.1016/j.actamat.2018.12.014
- Neeraj T, Srinivasan R, Ju L. Hydrogen embrittlement of ferritic steels: observations on deformation microstructure, nanoscale dimples and failure by nanovoiding. Acta Mater. 2010;60:5160–5171. doi: 10.1016/j.actamat.2012.06.014
- Nagumo M. Hydrogen related failure of steels – a new aspect (overview). Mater Sci Tech. 2004;20:940–950. doi: 10.1179/026708304225019687
- Nagumo M, Takai K. The predominant role of strain-induced vacancies in hydrogen embrittlement of steels: overview. Acta Mater. 2019;165:722–733. doi: 10.1016/j.actamat.2018.12.013
- Hirth JP. The role of hydrogen in enhancing plastic instability and degrading fracture toughness in steels. In: Thompson AW, Moody NR, editors. Hydrogen effects in materials. Warrendale (PA): TMS; 1996. p. 507–522.
- Lynch SP. Environmentally assisted cracking: overview of evidence for an adsorption-induced localized-slip process. Acta Metall. 1988;36:2639–2661. doi: 10.1016/0001-6160(88)90113-7
- Kirchheim R. Reducing grain boundary, dislocation line and vacancy formation energies by solute segregation. Acta Mater. 2007;55:5129–5138. doi: 10.1016/j.actamat.2007.05.047
- Matsumoto R, Taketomi S. Atomistic study of hydrogen effects on stability and mobility of vacancy and vacancy-clusters. Proceedings of Hydrogenius and I2CNER Joint Research Symposium on Hydrogen-materials Interactions. Fukuoka: Kyushu University; 2018. p. 13–23.
- Suzuki N, Ishii N, Miyagawa T, et al. Estimation of delayed fracture properties of steels. Tetsu-to-Hagan. 1993;79:227–232. Japanese. doi: 10.2355/tetsutohagane1955.79.2_227
- Yamazaki S, Takahashi T. Evaluation method of delayed fracture properties of high strength steels. Tetsu-to-Hagané. 1997;83:454–459. Japanese. doi: 10.2355/tetsutohagane1955.83.7_454
- Takagi S, Inoue T, Hara T, et al. Parameters for the evaluation of hydrogen embrittlement of high strength steel. Tetsu-to-Hagané. 2000;86:689–695. Japanese. doi: 10.2355/tetsutohagane1955.86.10_689
- Takagi S, Inoue T, Tsuzaki K, et al. Evaluation of hydrogen embrittlement susceptibility of high strength steel by the Weibull stress. J Jpn Inst Metals. 2001;65:1073–1081. Japanese. doi: 10.2320/jinstmet1952.65.12_1073
- Wang E, Akiyama E, Tsuzaki K. Determination of the critical hydrogen concentration for delayed fracture of high strength steel by constant load test and numerical calculation. Corros Sci. 2006;48:2189–2202. doi: 10.1016/j.corsci.2005.07.010
- International Organization for Standardization (ISO). Measurement method for the evaluation of hydrogen embrittlement resistance of high strength steels. ISO; 2015. ISO 16573.
- Oriani RA, Josephic PH. Equilibrium aspects of hydrogen-induced cracking of steels. Acta Metall. 1974;22:1065–1074. doi: 10.1016/0001-6160(74)90061-3
- Kushida Y. Hydrogen entry into steel by atmospheric corrosion. ISIJ Int. 2001;43:470–474. doi: 10.2355/isijinternational.43.470
- Takai K, Shoda H, Suzuki H, et al. Lattice defects dominating hydrogen-related failure of metals. Acta Mater. 2008;56:5158–5167. doi: 10.1016/j.actamat.2008.06.031
- Owada N, Majima H, Eguchi T. Effects of alloying elements on delayed fracture. Advances in Delayed Fracture Solution – Report of Research Group. Tokyo: Iron Steel Inst. Jpn; 1997. p. 111–114. Japanese.
- Kameda J. Equilibrium and growth characteristics of hydrogen-induced intergranular cracking in phosphorus-doped and high purity steels. Acta Metall. 1986;34:1721–1735. doi: 10.1016/0001-6160(86)90119-7
- Nagumo M, Ohta K, Saitoh H. Deformation induced defects in iron revealed by thermal desorption spectroscopy of tritium. Scr Mater. 1999;40:313–319. doi: 10.1016/S1359-6462(98)00436-9
- Fuchigami H, Minami H, Nagumo M. Effect of grain size on the susceptibility of martensitic steel to hydrogen embrittlement. Philos Mag Lett. 2006;86:21–29. doi: 10.1080/09500830500482316
- Nagumo M, Matsuda H. Function of hydrogen in intergranular fracture of martensitic steels. Philos Mag A. 2002;82:3415–3425. doi: 10.1080/01418610208240452
- Takai K, Watanuki R. Hydrogen in trapping states innocuous to environmental degradation of high-strength steels. ISIJ Int. 2003;43:520–526. doi: 10.2355/isijinternational.43.520
- McMahon Jr CJ. Mechanism of intergranular fracture in alloy steels. Mater Charact. 1991;26:269–287. doi: 10.1016/1044-5803(91)90017-X
- Bernstein IM. The role of hydrogen: is the story any clearer? In: Thompson AW, Moody NR, editors. Hydrogen effects in materials. Warrendale (PA): TMS; 1996. p. 3–11.
- Takai H, Yamauchi G, Nakamura M, et al. Hydrogen trapping characteristics of cold-drawn pure iron and eutectoid steel evaluated by thermal desorption spectroscopy. J Jpn Inst Metals. 1998;62:267–275. Japanese. doi: 10.2320/jinstmet1952.62.3_267
- Doshida T, Takai K. Dependence of hydrogen-induced lattice defects and hydrogen embrittlement of cold-drawn pearlitic steels on hydrogen trap state, temperature, strain rate and hydrogen content. Acta Mater. 2014;79:93–107. doi: 10.1016/j.actamat.2014.07.008
- Hattori M, Suzuki H, Seko Y, et al. The role of hydrogen-enhanced strain-induced lattice defects on hydrogen embrittlement susceptibility of X80 pipeline steel. JOM. 2017;69:1376–1380. doi: 10.1007/s11837-017-2371-1
- Nagumo M, Sekiguchi S, Hayashi H, et al. Enhanced susceptibility to delayed fracture in pre-fatigued martensitic steel. Mater Sci Eng A. 2003;344:86–91. doi: 10.1016/S0921-5093(02)00403-3
- Doshida T, Suzuki H, Takai K, et al. Enhanced lattice defect formation associated with hydrogen and hydrogen embrittlement under elastic stress of a tempered martensitic steel. ISIJ Int. 2012;52:198–207. doi: 10.2355/isijinternational.52.198
- Kido M, Nakasa K, Takei H. Delayed fracture under repeating stress in various high strength steels. Tetsu-to-Hagané. 1979;65:535–541. Japanese. doi: 10.2355/tetsutohagane1955.65.5_535
- Izutsu K, Takai K, Nagumo M. Effect of cyclic stressing on delayed fracture of high strength steel. Tetsu-to-Hagané. 1997;83:371–376. Japanese. doi: 10.2355/tetsutohagane1955.83.6_371
- Nagumo M, Uyama H, Yoshizawa M. Accelerated failure in high strength steel by alternating hydrogen-charging potential. Scr Mater. 2001;44:947–952. doi: 10.1016/S1359-6462(00)00683-7
- Doshida M, Nakamura M, Saito H, et al. Hydrogen-enhanced lattice defect formation and hydrogen embrittlement of cyclically prestressed tempered martensitic steel. Acta Mater. 2013;61:7755–7766. doi: 10.1016/j.actamat.2013.09.015
- Traiviratana S, Bringa EM, Benson DJ, et al. Void growth in metals: atomistic calculations. Acta Mater. 2008;56:3874–3886. doi: 10.1016/j.actamat.2008.03.047
- Tang T, Kim S, Horstemeyer MF. Molecular dynamics simulations of void growth and coalescence in single crystal magnesium. Acta Mater. 2010;58:4742–4759. doi: 10.1016/j.actamat.2010.05.011
- Zhang Y, Jiang S. Investigation on dislocation-based mechanisms of void growth and coalescence in single crystal and nanotwinned nickels by molecular dynamics simulation. Philos Mag. 2017;97:2772–2794. doi: 10.1080/14786435.2017.1352108
- Chandra S, Samal MK, Chavan VM, et al. Void growth in single crystal copper-an atomistic modeling and statistical analysis study. Philos Mag. 2018;98:577–604. doi: 10.1080/14786435.2017.1412591
- Hirth JP. Effects of hydrogen on the properties of iron and steel. Metall Trans A. 1980;11A:861–890. doi: 10.1007/BF02654700
- Nagumo M. Fundamentals of hydrogen embrittlement. Singapore: Springer Nature; 2016; Chapter 3, Interactions of hydrogen with lattice defects; p. 35–63.