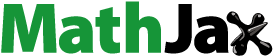
ABSTRACT
In this account, bent-core molecules involving the polar cyano-group are reviewed with a focus on the intensely investigated class of 4-cyanoresorcinol bisbenzoates. Cybotaxis and phase biaxiality of their nematic phases, the transition from nematic to smectic phases and the development of tilt and polar order in their smectic phases are discussed. Of special interest are heliconical (twist–bend) smectic intermediate phases occurring at the transition from paraelectric to antiferroelectric polar smectic phases if coinciding with the transition between anticlinic and synclinic tilt correlation. For some compounds, a reentrant polar SmA phase (SmALTPF) is observed below SmC and a silylated compound forms a new leaning-type phase. The formation of these achiral phases instead of the polar SmC phases involving layer chirality is discussed under the aspect of enantiophobic vs. enantiophilic organization. The effects of core structure and chain branching and, in addition, the effects of the intrinsic transient molecular, superstructural, and permanent molecular chirality on LC self-assembly are discussed.
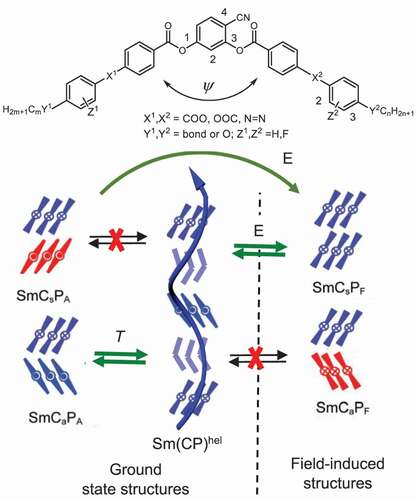
1. Preamble
Prof. B. K. Sadashiva is well known to me from his papers, several conferences and some visits at the Raman Research Institute (RRI) in Bangalore where he kindly invited and hosted me. Moreover, two of his former students Dr R. A. Reddy and Dr H. N. S. Murthy worked in Halle as Postdocs with me and Prof. Weissflog, respectively. Especially, I remember a post-conference excursion after the Keystone conference to Arches National Park with Prof. B. K. Sadashiva, Prof. W. Weissflog and Prof. R. Stannarius () where we got to know him more personally. Like me, BKS was a synthetic organic chemist focusing upon structure–property relationships to find new properties and phases in liquid crystalline (LC) materials. Besides being well known for his co-authorship in the fundamental paper on discotic LCs together with S. Chandrasekhar and K. A. Suresh [Citation1], he is also well recognized for his work on re-entrant nematic phases [Citation2] and on metallomesogens [Citation3,Citation4], with a focus on the search for phase biaxiality. Since the beginning of this century his work was focused on bent-core liquid crystals (BCLCs), especially fluorine substituted [Citation5,Citation6,Citation7] and those forming non-tilted biaxial and polar smectic phases, where he made significant contributions [Citation8,Citation9–11]. The research interests and topics of ours and B.K. Sadashivas were occasionally closely related, and in fact, the reports on ferroelectric switching in achiral bent-core LCs appeared almost simultaneously in the same year 2002 from both of our groups. We reported it for chain-silylated BCLCs [Citation12], whereas his compounds were based on core-fluorinated BCLCs [Citation13]. The same happened when the first SmAb phases were found in low molecular weight systems. He and his colleagues from the Raman Research Institute (RRI) reported them for mixtures of rod-like and bent-core molecules [Citation14], whereas our approach was based on sanidic metallomesogens [Citation15].
Figure 1. (Colour online) Left side: Prof. R. Stannarius, Prof. W. Weissflog, Prof. B.K. Sadashiva, (left to right in the inset) and I (the photographer) hiking in the Arches National Park after the Keystone ILCC in 2006; right-hand side pictures were used with courtesy of W. Weissflog.
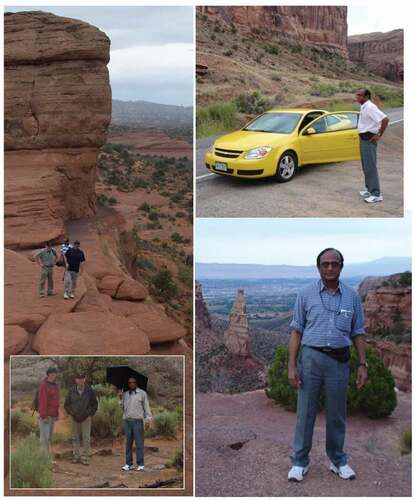
Scheme 1. Examples of bent molecules involving CN substituted aromatic cores and their typical LC phases; compounds CN1–CN4 have been reported by B.K. Sadashiva et al.
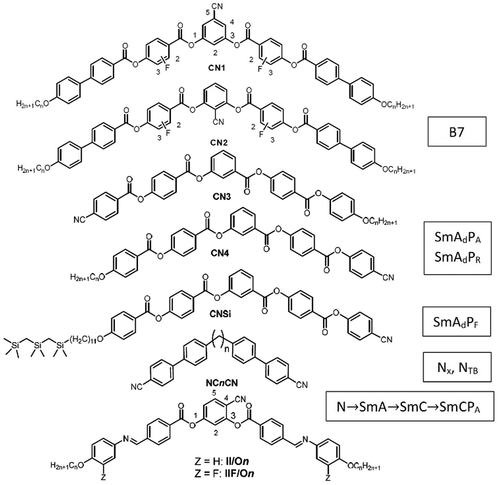
BKS also provided first reports on BCLC involving cyano groups. After its introduction by G. Gray in 1973 [Citation16], the CN group became one of the most influential groups in LC science. BKS has shown in his work that replacing one terminal alkyl chain at the bent core by a CN end group leads to antiparallel packing of the aromatic cores (CN3, CN4, ,
Figure 2. The intercalated double layer and ‘single layer’ polar SmA phases of BCLCs [A, R, and F stand for antipolar, randomized and synpolar (ferroelectric) order between adjacent layers].
![Figure 2. The intercalated double layer and ‘single layer’ polar SmA phases of BCLCs [A, R, and F stand for antipolar, randomized and synpolar (ferroelectric) order between adjacent layers].](/cms/asset/875143de-9843-4e67-938a-adf17f30c2b2/tlct_a_2010142_f0002_b.gif)
Later, bent molecules with CN groups at both ends (the bent dimesogens NCnCN in ) lead to the first compounds showing the twist–bend nematic (Nx, NTB) phase, theoretically predicted by R. B. Meyer [Citation25] and I. Dozov [Citation26] and experimentally observed by V.P. Panov et al. [Citation27] and others [Citation28–35].
During his research, BKS also introduced the CN group into other positions at the bent polyaromatic core. Thus, he reported first examples of BCLCs having CN groups attached to the central bent unit, either in 2- or in 5-position, (CN2 and CN1, ) [Citation36–38]. Similar to the related NO2 substituted compounds, this polar substituent provides polar and modulated smectic phases (B7 phases) showing fascinating textures involving helical filaments [Citation39,Citation40]. The only remaining substitution pattern being unexplored at that time was the 4-CN substitution. This class of compounds was found to provide new phase structures and a variety of insights into the principles of development of chirality, polarity and tilt in soft self-assembly as will be shown in this review.
2. Introduction
4-Cyanoresorcinol based LCs (compounds II/On, IIF/On, BB/On and TT/O8,O12, ) have been first synthesized and investigated by W. Weissflog et al. in Halle and lead to phase sequences like N – SmA – SmC – B2, on cooling [Citation41,Citation42,Citation43], involving LC phases being typical for rod-like as well as for BCLCs [Citation44,Citation45,Citation46]. The main focus of his work was on the Schiff base derived compounds II/On and IIF/On (). For a peripheral core-fluorinated compound of type IIF/On, a non-tilted single layer biaxial SmAPA phase was for the first time postulated based on apparent X-ray evidence [Citation46]. Further work was focused on the transition between the uniaxial and biaxial smectic phases in thin films [Citation47] and in bulk [Citation48]. NMR studies revealed an opening angle ψ between the two Schiff base wings () being substantially larger than 120°, increasing from 136° at 77°C to 143° at 167°C [Citation121]. This widening of the opening angle is due to a combination of dipole compensation between C=O and CN, steric and stereoelectronic interactions around the COO group in 3-position, and it leads to molecules with an effective average shape at the cross-over between classical rod-like (ψ = 180°) and bent-core mesogens (αψ ~ 120°), showing transitions from non-polar to polar LC phases [Citation44]. The large opening angle ψ of the core and the presence of the CN group also promote nematic phases [Citation44,Citation50], which are rare among the typical bent-core mesogens with 120° bend [Citation22,Citation51,Citation52,Citation53].
Our interest in these bent compounds arose from their capability of forming weakly tilted and orthogonal smectic as well as nematic phases. The initial target was to use them as building blocks for the design of LCs forming the orthorhombic biaxial nematic phase [Citation53]. Though this phase was never achieved, the research on these compounds was very fruitful and let to new knowledges and LC phase types. The focus of this review is on the 4-cyanoresorcinol bisbenzoates collated in . Most of these compounds involve COO groups with different directions and in some cases also azo groups (N=N) as linking units X in their rod-like wings. Compounds with identical wings (AA, BB, PP, TT) as well as those combining different wings (BT, TB, AB, AT …) and compounds being fluorinated at the periphery (Z = F) or having branched chains were also investigated. These investigations have been spread over numerous scientific papers and the models proposed for their organization have changed during the course of the ongoing investigations. The purpose of this contribution is to summarize the major conclusions of this work and to provide a bird’s eye view over the present state of understanding of their LC self-assembly, though not all details are fully understood yet.
Scheme 2. Major compounds under discussion and the used abbreviations. The initial letters indicate the type of rod-like wing groups attached to the 4-cyanoresorcinol unit; I = imine (Schiff base), B = phenylbenzoate, T = phenylterephthalate, A = azobenzene and P = biphenyl; combinations indicate two different wing groups, the first letter indicating that one at the 1-position and the second that at the 3 position of the 4-cyanoresorcinol core unit. The connecting unit Y (single bond or O) and chain length m, n is given after the slash, only one number n indicates two identical chains (m = n). For compounds being fluorinated at the outer ring (Z = F) F is added before the slash.
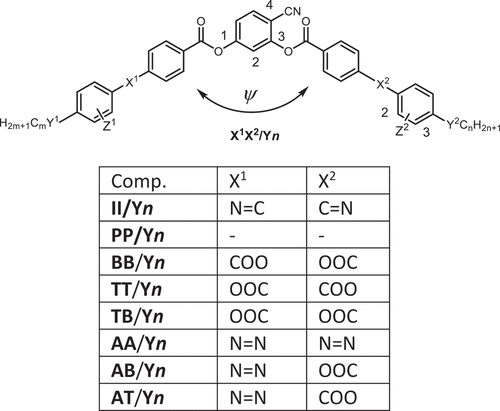
3. 4-Cyanoresorcinols as molecules at the rod-banana cross-over
3.1. Synthesis, purification and chemical stability
Scheme 3. Synthesis of the 4-cyanoresorcinol derived bent-core mesogens. Reagents and conditions: i: (1) H2NOH, (2) Ac2O; ii: NaOH/H2O; iii: (1) 1/n or 1/m, SOCl2, 80°C, 2 hrs, (2) removal of excess SOCl2, (3) 2/n or 3 (0.5 equ), DCM, pyridine, 50°C, 2 hrs.; iv: H2/Pd/C; for the structure and abbreviations of the target compounds (see .
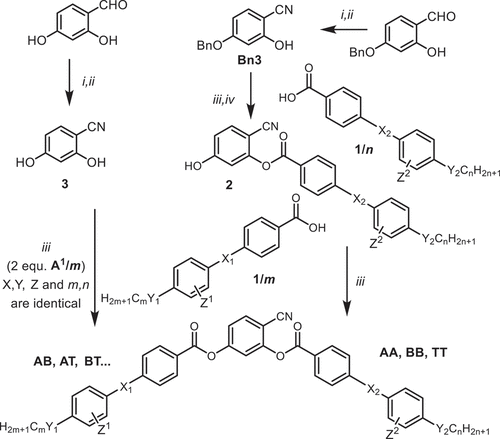
Though the synthesis of the 4-cyanoresorcinol based BCLCs is straightforward, starting from commercially available 2,4-dihydroxybenzaldehyde and using well established procedures (), it requires some experience to obtain sufficiently pure and long-time stable products. Especially, it must be considered that the CN group increases the reactivity of the adjacent COO groups (especially in position 3) against nucleophilic attack. Such ‘active esters’ are prone to acyl group transfer, scrambling the COO groups (transesterification) in the molecules not only during synthesis, but also during purification (chromatography and recrystallization), storage and investigation in the temperature ranges of the LC phases. Therefore, the use of common acylation methods with dicyclohexylcarbodiimide (DCC) as a condensation reagent and related reactions, especially those requiring 4-dimethylaminopyridine (DMAP) as acylation catalyst [Citation54], cannot be recommended. Tiny traces of these reagents catalyze the acyl group transfer reactions. This problem is especially serious for molecules involving more than only one type of COO linkage (non-symmetric molecules and multiple COO groups, as compounds AA, AB and BB, see ) and involving electron deficit benzoic acids (terephthalates), which additionally enhances the reactivity. Much better is the classical acylation by first activation of the benzoic acids with SOCl2 or oxalyl chloride to give the benzoyl chloride, careful removal of the excess reagents, followed by reaction with the appropriate phenols with pyridine as base in a second step, which usually leads to pure products being long term stable. Symmetric compounds were simply obtained by acylation of 4-cyanoresorcinol (3), whereas non-symmetric compounds require the use of (benzyl)protected 4-cyanoresorcinol (Bn3) and a second acylation step of 2/n after deprotection (). shows typical LC phase sequences observed for the selected representative examples from each series with Z = H and m = n = 12.
Table 1. LC phases and transition temperatures of selected 4-cyanoresorcinol based BCLCs with either two alkyl (n) or alkoxy chains (On).a
3.2. Cybotaxis and local biaxiality in the nematic phases
3.2.1. Cybotaxis
The nematic phases of BCLCs arose significant interest, especially with respect to their potential biaxiality and polar order [Citation53,Citation55–58]. A typical feature of these N phases is cybotaxis (local smectic cluster formation), which was for a long time assumed to represent exclusively a pre-transitional phenomenon [Citation59], but work on bent-core mesogens, especially the 4-cyanoresorcinols [Citation49], 4-halogenated resorcinols [Citation60,Citation61] and oxadiazoles [Citation62–64], has shown that for the nematic phases of these molecules, cybotaxis represents a general feature. The correlation length of these cybotactic clusters increases with growing chain length and decreasing temperature, as confirmed by XRD and dielectric spectroscopy (see ) [Citation49,Citation65,Citation66]. The usually observed phase type is the skewed cybotactic nematic phase composed of small SmCs clusters (NCybC), which is characterized by the typical dumbbell shaped diffuse SAXS scattering of magnetically aligned samples [Citation49,Citation62]. The non-tilted SmA type (NCybA) [Citation53,Citation67], showing only one diffuse scattering maximum on the meridian, is only rarely observed. There is still some controversy if the cybotactic clusters represent discrete domains in a continuum of the nematic phase [Citation66], as suggested by cryo-transmission electron microscopy (cryo-TEM) imaging [Citation68,Citation69], or uniform and completely dynamic fluctuating structures with smectic clusters popping in and out of existence, thus combining local smectic with long-range nematic order. We prefer the second view and assume that only after cryo-treatment, discrete clusters become fixed and then can be observed on a mesoscopic level.
Figure 3. (Colour online) (a) 2D SAXS patterns of magnetically aligned samples in the NCybC phases of compounds BB/n depending on chain length and on temperature, (b) their SAXS plots with (c) model of the molecular organization in the NCybC phases and (d) explanation of the alignment of the molecules under the applied magnetic field. Reprinted from ref. [Citation49], copyright 2010, RSC.
![Figure 3. (Colour online) (a) 2D SAXS patterns of magnetically aligned samples in the NCybC phases of compounds BB/n depending on chain length and on temperature, (b) their SAXS plots with (c) model of the molecular organization in the NCybC phases and (d) explanation of the alignment of the molecules under the applied magnetic field. Reprinted from ref. [Citation49], copyright 2010, RSC.](/cms/asset/f9b034a1-f3a4-49c7-8449-e0400b808297/tlct_a_2010142_f0003_oc.jpg)
These dynamic clusters give rise to a change of macroscopic properties, such as an increased viscosity of these nematic phases [Citation70] and special elastic and flexoelectric properties [Citation71,Citation72]. For example, for BB/O12, the bend elastic constant k33 was found to be much smaller than the splay elastic constant k11 in the whole temperature range [Citation73] due to the twisted and bend molecular shape [Citation74]. This allows the preparation of mixtures with typical NTB forming materials such as NCnCN () retaining the NTB phase in these mixtures up to relatively high concentrations of the 4-cyanoresorcinol based compound [Citation75]. Under an applied electric field the formation of striped electroconvection patterns [Citation55,Citation76,Citation77] is a common feature of the bent-core nematic phases. Sometimes, the distance between the convection roles becomes so small that it is in the range of visible wave length, and then, the texture appears like a fan-texture of a planar aligned smectic phase [Citation55]. In some cases, an inversion of the dielectric anisotropy (Δε) depending on chain length and temperature is observed [Citation65,Citation70,Citation78]. For example, for the compounds BB/n (), Δε is positive at high T and negative at low T for the short chain compounds BB/4 – BB/9 and always negative for BB/10 with long chains. The negative Δε is due to the hindrance of the rotation of the individual molecules around their long axes emerging from the local packing in the cybotactic clusters and is observed after exceeding a certain cluster size. This hindered rotation can also support the development of a local polarization within the clusters [Citation55,Citation66] as well as the partial synchronization of molecular conformers [Citation79–81], as will be discussed in Section 4. However, on a macroscopic scale, these effects are canceled out due to the randomization of the domain orientation, leaving only the nematic long-range order [Citation82].
It is noted that the main reason for cybotaxis is not the bend molecular shape, but the relatively large size of the rigid molecular fragments used as building blocks of BCLCs (usually ≥5 benzene rings), thus providing a significant source of nano-scale segregation from the flexible alkyl end-chains (rigid-flexible and chemical incompatibilities), which increases with growing chain length [Citation83]. Therefore, it is not surprising that the cybotaxis in nematic phases is a quite general phenomenon which can be observed for any mesogen involving either an extended aromatic core or having any other kind of amphiphilicity, combined with a competing frustration force inhibiting the formation of infinite layers, as for example caused by lateral substituents [Citation84]. In the case of BCLCs, the layer distorting effect is mainly due to the molecular bend und twist, as well as by the developing local polar order which tends to be canceled out by emerging twist, splay or any other kind of layer modulation.
3.2.2. Phase biaxiality
There was a long-lasting debate concerning the potential biaxiality of the nematic phases of BCLCs (
Figure 4. Nematic phases of bent-core molecules: (a) uniaxial nematic phase of molecules rotationally disordered around their long axis (Nu), (b) restricted rotation around n, but without polar order, leads to the orthorhombic D2h symmetry (Nbo phase), (c) biaxial nematic phase with monoclinic C2h symmetry (Nbm phase), and (d,e) clusters where the view is along direction z of the reference system; (d) rotationally disordered biaxial clusters, leading to uniaxiality; (e) phase biaxiality due to alignment of the biaxial clusters. Reprinted from ref. [Citation53], copyright 2010, RSC.
![Figure 4. Nematic phases of bent-core molecules: (a) uniaxial nematic phase of molecules rotationally disordered around their long axis (Nu), (b) restricted rotation around n, but without polar order, leads to the orthorhombic D2h symmetry (Nbo phase), (c) biaxial nematic phase with monoclinic C2h symmetry (Nbm phase), and (d,e) clusters where the view is along direction z of the reference system; (d) rotationally disordered biaxial clusters, leading to uniaxiality; (e) phase biaxiality due to alignment of the biaxial clusters. Reprinted from ref. [Citation53], copyright 2010, RSC.](/cms/asset/626fa4d8-4bd4-402e-838b-5265ddd0fbe1/tlct_a_2010142_f0004_oc.jpg)
Though it was tried hard, there is presently no indication of the orthorhombic type of biaxial nematic phase or state (Nbo) derived from the NcybA phase ()) [Citation53]. One reason is that non-tilted LC phases are rare for bent molecules [Citation53,Citation67]. Moreover, the SmA phases of bent-core mesogens can be considered as de Vries-like SmA phases formed by SmCs clusters being too small to assume a long-range uniform alignment of the tilt direction [Citation47,Citation90–92]. In line with the de Vries character, a typical feature of these SmA phases of bent-core molecules (which in fact represent SmCs phases with short range tilt correlation) is the absence of a change of the d-spacing at the SmA-SmCs transitions. Due to the smaller tilt and cluster size, the randomization of the secondary director is much easier than in the NCybC phase.
3. 3. Nematic to smectic transitions
The NCybA-SmA and NCybC-SmCs transitions on cooling are more complicated than the N-Sm transition known for classical rod-like mesogens as the clusters are so dominant and continuously or stepwise grow on cooling. Moreover, the clusters can assume different shapes (oblate, prolate and ribbon-like) and develop local polar order [Citation49]. Therefore, often additional intermediate phases emerge via continuous phase transitions or transitions with very small enthalpies. Among them, cluster phases that were tentatively designated as CybC/CybA and cannot be unambiguously assigned to either nematic or smectic phases (e.g. by the SAXS line width) are considered to be composed of giant clusters [Citation49,Citation93]. Sometimes the low temperature ranges of the NCybC phases mimic textures of biaxial nematic phases [Citation94] and NTB phases [Citation32,Citation95]. However, in order to identify any NTB-like twisted intermediate phases, confirmation of the helical structure by at least one appropriate method (TEM [Citation69] or resonant soft X-ray scattering, RSoXS [Citation96,Citation97]) would be required.
3. 4. Development of tilt and polar order in the smectic phases of azobenzenes AA/On and AAF/On
shows the typical chain length and temperature dependence of the phase transitions of 4-cyanoresorcinol based BCLCs for two series of compounds containing two azobenzene wings as examples. Compounds AA/On have relatively electron rich wing groups with a strong driving force for uniform tilt (~30 °) and forming exclusively synclinic tilted NCybC and SmCs phases (besides Colrec, )) [Citation98,Citation99]. The series of fluorinated compounds AAF/On, ()) having reduced electron density in the wings due to the electron withdrawing F atoms at the periphery, shows a reduced tilt (~15–20°) [Citation100,Citation101]. This enables the formation of NCybA and SmA phases for the short chain compounds; the SmA phase even persists for long alkyl chains in certain T-ranges, and there is an additional transition from synclinic to anticlinic tilt in the polar antiferroelectric SmCPA phases at lowest temperature. In both homologous series, the synclinic tilt becomes more dominating with growing chain length, though the tilt angle itself does not change significantly. For the effects of other fluorination patterns, see ref. [Citation100].
Figure 5. (Colour online) Effect of chain length and temperature on the phase types and phase sequences of (a) compounds AA/On with two identical azobenzene wings and (b) the related peripheral wing-fluorinated compounds AAF/On [Citation98,Citation100]. Reprinted from ref. [Citation100], copyright 2016, Wiley-VCH.
![Figure 5. (Colour online) Effect of chain length and temperature on the phase types and phase sequences of (a) compounds AA/On with two identical azobenzene wings and (b) the related peripheral wing-fluorinated compounds AAF/On [Citation98,Citation100]. Reprinted from ref. [Citation100], copyright 2016, Wiley-VCH.](/cms/asset/21353c87-52c3-4472-9232-08407ab047cc/tlct_a_2010142_f0005_oc.jpg)
Similar phase sequences were found for the series AB, BB, AT, BT, TB and TT involving only one azo group or exclusively COO linking units (). The compounds having one or both azobenzenes of compounds AA replaced by the electron rich phenyl benzoate wing (AB, BB) show even lower phase stability and exclusively strongly tilted phases [Citation49,Citation102], whereas those involving at least one electron deficit phenyl terephthalate unit (TT, AT, BT, TB) show much higher isotropization temperatures, a smaller tilt and have a SmA high temperature phase [Citation92,Citation102,Citation103]. Details of these series are described in the references given in ; the terepthalates TT will be discussed in the following sections in more detail.
3. 5. Development of polar order in the smectic phases of terephthalates TT/n and TT/On
The development of polar order was in detail studied for compound TT/O6, having two electron deficit terephthalate based wings (
Figure 6. (Colour online) Field-dependence of the SHG signal (left) and polarization current curves under a triangular wave voltage (200 Vpp, 10 Hz, 6 μm ITO cell, right) of compound TT/O6 depending on temperature (a,d) SmAPR range (c is most likely a conductivity peak); (b,e) SmAPAR range of the SmA phase (in (e) there is still a small residual peak from SmAPR) and (c,f) SmCaPA phase, the sharp double peaks in (f) and the discontinuous field-dependence in (c) demonstrate the AF character of the switching. Reprinted from ref. [Citation108], copyright 2017, Wiley-VCH.
![Figure 6. (Colour online) Field-dependence of the SHG signal (left) and polarization current curves under a triangular wave voltage (200 Vpp, 10 Hz, 6 μm ITO cell, right) of compound TT/O6 depending on temperature (a,d) SmAPR range (c is most likely a conductivity peak); (b,e) SmAPAR range of the SmA phase (in (e) there is still a small residual peak from SmAPR) and (c,f) SmCaPA phase, the sharp double peaks in (f) and the discontinuous field-dependence in (c) demonstrate the AF character of the switching. Reprinted from ref. [Citation108], copyright 2017, Wiley-VCH.](/cms/asset/c953f0a1-a032-47cf-81b0-3d642acac70a/tlct_a_2010142_f0006_oc.jpg)
3. 6. The general phase sequence and intermediate phases
The sequence SmAPR-SmAPAR-SmCaPA is observed for all other 4-cyanoresorcinol based BCLCs with at least one electron deficit wing (TT/n, BT/n and AAF/On) and relatively short alkyl chains. For homologues with longer chains, biaxiality develops first and the transition from apolar via paraelectric to polar smectic phases takes place within the synclinic SmCs range (see )). This leads to SmCsPR and SmCsPAR ranges [Citation98], representing new biaxial analogues of the previously known uniaxial SmAPR and SmAPAR ranges of the SmA phase [Citation105–107]. In addition, the anticlinic SmCaPA phase is fully or partly replaced by the synclinic SmCsPA phase.
Considering the de Vries character of the SmA phases of most BCLCs, it is likely that local tilt is already present in the uniaxial paraelectric SmAP(A)R ranges and the domain size exceeds the critical length for long-range tilt correlation in the biaxial SmCsP(A)R phases. It is noted that the SmCsPAR range of the paraelectric SmCs phase was first thought to be a ferroelectric SmCsPF phase, because in freely suspended films, the switching was experimentally found to be bistable (ferroelectric) [Citation98]. However, it appears that the growing polar domains tend to assume an antipolar modulation which is suppressed in the freely suspended films (SmCsPF) and supported by the strong surface anchoring on glass substrates (SmCsPAR). This antipolar modulation can obviously also lead to the emergence of new modulated phases (M1/SmsPA, ) emerging at the SmCsPAR
SmCsPA transition of compounds with relatively strong tilt. The development of layer modulation can be deduced from a change of the texture from fan-like to mosaic-like, associated with a substantial increase of viscosity (
Figure 7. (Colour online) Texture showing the coexistence of the M1-phase of compound TT/O14 with (a) the SmCsPR phase at T = 133°C and (b) with the uniaxial heliconical Sm(CP)hel phase at T = 130.5°C as observed on cooling in a homeotropic 8 μm cell. Reprinted from ref. [Citation110], copyright 2014, RSC.
![Figure 7. (Colour online) Texture showing the coexistence of the M1-phase of compound TT/O14 with (a) the SmCsPR phase at T = 133°C and (b) with the uniaxial heliconical Sm(CP)hel phase at T = 130.5°C as observed on cooling in a homeotropic 8 μm cell. Reprinted from ref. [Citation110], copyright 2014, RSC.](/cms/asset/5f5203f4-b73a-483d-aa40-5e6df9d529c4/tlct_a_2010142_f0007_oc.jpg)
Figure 8. (Colour online) Development of tilt, tilt correlation and polar order (P) in the LC phases of BCLCs at the cross-over between linear and bent molecular shapes, depending on temperature (T), molecular opening angle (ψ) and molecular structure; dark gray arrows indicate phase transition, light gray arrows refer to changing polar order in the same phase; non-tilted phases are indicated in blue, synclinic in red and anticlinic in green. At the bottom, the phase sequences are associated to typical series of 4-cyanoresorcinol based BCLCs (see ).
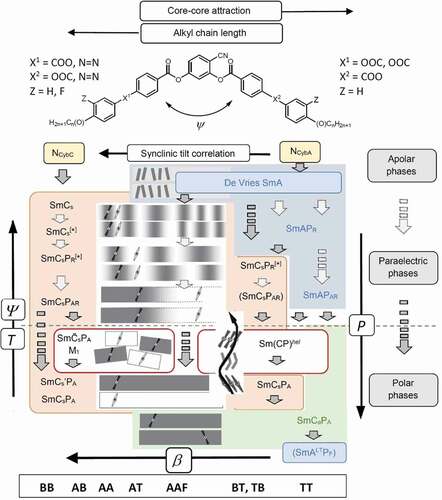
The phase sequences observed for most investigated 4-cyanoresorcinol derived BCLCs depending on tilt correlation and temperature are summarized in [Citation98–103,Citation108–111]. It is recognized that the modulated phases occur only for molecules forming strongly tilted SmCs phases (left hand side of ), whereas compounds with smaller tilt and involving an SmA phase (blue) at higher temperature and an anticlinic SmCsPA phase (green) at low T (right hand side of ) form heliconical intermediate phases (Sm(CP)hel) instead; this will be discussed in Section 4.1.
3.7. Synclinic vs. anticlinic tilt
In the SmC phases of the weakly bent 4-cyanresorcinols the electric field induced switching typically takes place by rotation around the long axis (
Figure 9. (Colour online) Effects of the opening angle and the molecular rotation on (a) the switching process and (b) the out-of-layer fluctuations (green arrows); (c) the structure of tilted smectic phases formed by molecules with a shape at the transition between linear and bent.

Figure 10. (Colour online) Bar diagrams of compounds (a) TT/n and (b) BT/m-n, showing the development of the LC phases on cooling and depending on chain length; for the long chain compounds of both series the phase sequence changes after application of a few cycles of an AC field after removing the field; the columns with E show the transitions of these pre-treated samples; abbreviations: SmCaPA(hel) = SmCaPA phase with short-range heliconical structure (though the short range heliconical structure appears to be involved in all SmCaPA phases of the series TT/n, it is only indicated for TT/14 where it was proven by RSoXS [Citation91]); SmCXPR; polarization randomized smectic phase with heliconical structure, SmALTPF = non-tilted polar low temperature phase; Cr’ = crystalline phase with SmA-like texture; for SmCaPA(F) (TT/14) AF [Citation92] as well as FE switching [Citation91] was reported, possibly there is a strong dependence on the experimental conditions for this compound at the borderline between AF (SmCaPA) and FE (SmALTPF) phases (see for other abbreviations) [Citation92,Citation103]. a) is adapted from Ref. [Citation92]; an expanded version is shown in Fig. S4 in the SI.
![Figure 10. (Colour online) Bar diagrams of compounds (a) TT/n and (b) BT/m-n, showing the development of the LC phases on cooling and depending on chain length; for the long chain compounds of both series the phase sequence changes after application of a few cycles of an AC field after removing the field; the columns with E show the transitions of these pre-treated samples; abbreviations: SmCaPA(hel) = SmCaPA phase with short-range heliconical structure (though the short range heliconical structure appears to be involved in all SmCaPA phases of the series TT/n, it is only indicated for TT/14 where it was proven by RSoXS [Citation91]); SmCXPR; polarization randomized smectic phase with heliconical structure, SmALTPF = non-tilted polar low temperature phase; Cr’ = crystalline phase with SmA-like texture; for SmCaPA(F) (TT/14) AF [Citation92] as well as FE switching [Citation91] was reported, possibly there is a strong dependence on the experimental conditions for this compound at the borderline between AF (SmCaPA) and FE (SmALTPF) phases (see Table 1 for other abbreviations) [Citation92,Citation103]. a) is adapted from Ref. [Citation92]; an expanded version is shown in Fig. S4 in the SI.](/cms/asset/b13ffdad-808c-43c5-afc5-92358bf0660e/tlct_a_2010142_f0010_oc.jpg)
3.8. The SmCaPA phase of low tilt compounds
The relations between chain length and LC phase type is shown in ) for the series of compounds TT/n. All compounds with n ≥ 6 have a transition from SmAPR via SmAPAR to polar smectic phases, being either anticlinic tilted (SmCaPA) for compounds with n up to n = 16, or synclinic tilted (SmCsPA) for those with longer chains (n ≥ 18). The emergence of tilt at the SmAP(A)R→SmCaPA transition is difficult to detect by optical or XRD investigation, because the tilt is relatively small and anticlinic, the switching in planar cells takes place by rotation around the long axis (no tilt or rotation of the extinction crosses is observed) and the layer spacing almost continuously increases on cooling without any discontinuity across the SmAP(A)R→SmCaPA transition (
Figure 11. (Colour online) (a-d) Textures of compound TT/4, (c, d) in the SmA phase at T = 118°C and (a, b) in the SmCaPA phase at T = 80°C. (a, c) show the homeotropic textures and (b, d) the planar textures; (e) dependence of the layer distance d of compounds TT/n on the chain length n and temperature; the grey bar indicates the temperature range of the paraelectric-(anti)ferroelectric transitions. Reprinted from ref. [Citation92], copyright 2020, Wiley-VCH.
![Figure 11. (Colour online) (a-d) Textures of compound TT/4, (c, d) in the SmA phase at T = 118°C and (a, b) in the SmCaPA phase at T = 80°C. (a, c) show the homeotropic textures and (b, d) the planar textures; (e) dependence of the layer distance d of compounds TT/n on the chain length n and temperature; the grey bar indicates the temperature range of the paraelectric-(anti)ferroelectric transitions. Reprinted from ref. [Citation92], copyright 2020, Wiley-VCH.](/cms/asset/3040bcb4-d23b-47d4-9378-747cb09fa718/tlct_a_2010142_f0011_oc.jpg)
Figure 12. (Colour online) The Sm(CP)hel phase. (a) SAXS and RSoXS data in the LC phases of TT/14 depending on temperature [Citation91]; (b) shows models [Citation128]; (d,e) Textures of compound BT12/6; the insets show the corresponding homeotropic textures; (f) polarization current curve of this compound as recorded under a triangular wave voltage at 200 Vpp, in a 6 μm PI-coated ITO cell. (a, b) were reprinted with permission from refs. [Citation91] and [Citation128], respectively, copyright (2019) by the American Physical Society (https://doi.org/10.1103/PhysRevLett.122.107801; https://doi.org/ 10.1103/PhysRevMaterials.3.045603).
![Figure 12. (Colour online) The Sm(CP)hel phase. (a) SAXS and RSoXS data in the LC phases of TT/14 depending on temperature [Citation91]; (b) shows models [Citation128]; (d,e) Textures of compound BT12/6; the insets show the corresponding homeotropic textures; (f) polarization current curve of this compound as recorded under a triangular wave voltage at 200 Vpp, in a 6 μm PI-coated ITO cell. (a, b) were reprinted with permission from refs. [Citation91] and [Citation128], respectively, copyright (2019) by the American Physical Society (https://doi.org/10.1103/PhysRevLett.122.107801; https://doi.org/ 10.1103/PhysRevMaterials.3.045603).](/cms/asset/4d71e5e7-47c6-45d1-a870-df5f786d2056/tlct_a_2010142_f0012_c.jpg)
4. Superstructural, supramolecular and transient molecular chirality
4.1. Heliconical smectic phase (Sm(CP)hel)
The most interesting feature of the series TT/n is the formation of a short pitch heliconical smectic phase (Sm(CP)hel), occurring for compounds with n > 10. It is obviously associated with the anticlinic→synclinic transition in smectic phases with small tilt and growing synpolar coherence length occurring just as polar order becomes long-range and before it becomes strictly antipolar [Citation92,Citation125,Citation127,Citation128]. The helical pitch P in terms of a number of smectic layers is incommensurate and slightly smaller than 3 times the thickness of a layer, as indicated by the RSoXS experiments ()) [Citation91]. This heliconical smectic phase has first been assumed to be an orthogonal SmAPα phase where the polar direction changes by a uniform angle unequal to 0° or 180° between the polar layers [Citation129]. Later, it was recognized that there is a small tilt which sets in together with the polar order (SmCPα) [Citation110]. The present designation is either SmCsPFhel [Citation127], Sm(CP)α [Citation91] or Sm(CP)hel, the latter is use herein [Citation92]. A typical feature associated with the Sm(CP)hel phase, indicating the tilted organization, is the formation of a field-induced so-called tiger stripe texture (
Figure 13. (Colour online) Optical investigation of the switching process of compound TT/12 in planar alignment (6 μm PI coated ITO cell) under an applied DC field in the Sm(CP)hel range at T = 105°C with models of the distinct field-induced states. Reprinted from ref. [Citation92], copyright 2020, Wiley-VCH.
![Figure 13. (Colour online) Optical investigation of the switching process of compound TT/12 in planar alignment (6 μm PI coated ITO cell) under an applied DC field in the Sm(CP)hel range at T = 105°C with models of the distinct field-induced states. Reprinted from ref. [Citation92], copyright 2020, Wiley-VCH.](/cms/asset/c4574cdf-f71c-47cd-9fd2-a9c41695b659/tlct_a_2010142_f0013_c.jpg)
Without applied field the texture of the Sm(CP)hel phase appears with the typical features of a SmA phase, indicated by a smooth fan texture and appearing optically isotropic under homeotropic alignment due to the presence of the tight helix parallel to the layer normal ()). The transition from a speckled to a plain fan texture is a good optical indication of the SmCaPA-Sm(CP)hel transition ()). As shown in
Figure 14. (Colour online) (a) Polarization values (Ps) of BT/12-6 depending on temperature (Cr 69 Sm(CP)hel 81 SmCaPA 83 SmAPR/SmA 131°C Iso) and (b) temperature dependence of the δε and relaxation frequency; (c) shows the temperature dependence of the SHG response I2ω of compound BT/18-6 (Cr (SmCaPA 69 Sm(CP)hel 82 SmCaPA 84 SmAPR/SmA 138°C Iso); the measurements were made on cooling in an AC electric field (Epp = 25 V/µm) in a 10 µm thick IPS cell with ITO electrodes. Reprinted from ref. [Citation103], copyright 2020, RSC.
![Figure 14. (Colour online) (a) Polarization values (Ps) of BT/12-6 depending on temperature (Cr 69 Sm(CP)hel 81 SmCaPA 83 SmAPR/SmA 131°C Iso) and (b) temperature dependence of the δε and relaxation frequency; (c) shows the temperature dependence of the SHG response I2ω of compound BT/18-6 (Cr (SmCaPA 69 Sm(CP)hel 82 SmCaPA 84 SmAPR/SmA 138°C Iso); the measurements were made on cooling in an AC electric field (Epp = 25 V/µm) in a 10 µm thick IPS cell with ITO electrodes. Reprinted from ref. [Citation103], copyright 2020, RSC.](/cms/asset/b09b1d49-69ea-46f9-9a5d-3b919dbcca78/tlct_a_2010142_f0014_c.jpg)
As shown in ), the Sm(CP)hel phase appears below a polarization randomized high permittivity SmAPR, SmAPAR, SmCsPAR or SmCsPR[*] phase and replaces the anticlinic SmCaPA phase partly or completely in a certain temperature range slightly below or at the paraelectric-(anti)ferroelectric transition. As the synclinic tilt correlation becomes stronger by chain elongation, the anticlinic SmCaPA phase is replaced by the synclinic SmCsPA phase for n = 16,18. This phase is achiral and as a result incompatible with helix formation, and as a consequence, the Sm(CP)hel phase is removed (see )). However, the Sm(CP)hel phase can still be induced under a pulsed E-field which switches the achiral SmCsPA phase to the chiral SmCsPF state by rotation around the long axis (
Figure 15. (Colour online) (a-d) Textures of TT/18 as observed in a planar cell at 100°C, showing the field induced transition of (a) SmCsPA to (b,c) SmCsPF, followed by relaxation to (d) Sm(CP)hel and (b-d) reversible switching between the two SmCsPF states and Sm(CP)hel at 0 V; (a-d) were used under CC BY 4.0 from ref. [Citation127]. (e) Summary of the field-induced transformations in the polar smectic phases of compounds TT/n and BT/m-n.
![Figure 15. (Colour online) (a-d) Textures of TT/18 as observed in a planar cell at 100°C, showing the field induced transition of (a) SmCsPA to (b,c) SmCsPF, followed by relaxation to (d) Sm(CP)hel and (b-d) reversible switching between the two SmCsPF states and Sm(CP)hel at 0 V; (a-d) were used under CC BY 4.0 from ref. [Citation127]. (e) Summary of the field-induced transformations in the polar smectic phases of compounds TT/n and BT/m-n.](/cms/asset/d36c817d-2b2e-40a1-881e-e7dddc0c29c9/tlct_a_2010142_f0015_c.jpg)
This barrier is smaller for the transition from Sm(CP)hel to SmCaPA by collective precession on a cone, retaining the layer chirality. Therefore, once the Sm(CP)hel phase is formed, the mode of switching changes from the originally preferred chirality inverting rotation around the long axis to a chirality preserving precession on a cone ()) [Citation22,Citation52,Citation133]. The interesting point is, that in this case it is not the degree of molecular bend or a layer modulation which decides about the switching mechanism, but it is the heliconical organization of the molecules, favoring the collective precession on a cone ‘kinetically’. Moreover, this process, retaining the layer chirality, retains the lowest energy diastereomeric pair of layer chirality and heliconical twist sense, favoring this process also ‘thermodynamically’. The switching process is also voltage dependent and changes to a rotation around the long axis above a threshold voltage required to remove the helix [Citation128]. For intermediate cases more complicated behavior can be found, as for example at the SmCsPR-Sm(CP)hel transition described in ref. [Citation125].
The Sm(CP)hel phase is also observed in the series of compounds BT/n, combining an electron deficit terephthalate with an electron rich phenylbenzoate wing [Citation103]. As shown in ), in this series of compounds BT/m-n, having different chain length at the ends, the Sm(CP)hel phase is stable for the shorter homologues whereas at a certain chain length it is replaced by SmCsPA from which the Sm(CP)hel phase is obtained as a field-induced phase in the whole SmCsPA ranges. It appears that for compounds BT/m-n there is a slightly higher tendency to assume a synclinic tilt as indicated by the complete removal of the anticlinic tilted phase in comparison to the same chain length range in the series TT/n (see , dashed lines). A similar slight shift towards synclinic tilt is also found by replacing one or both alkyl chains by alkoxy chains (BT/On) and by flipping the direction of the CN group (TB/n, TB/On = exchange of the positions of phenyl benzoate and terephthalate wings, see for examples and ref. [Citation103] for more details).
The switching of the Sm(CP)hel phase is of practical interest for application in electro-optical devices, either using the very fast V-shaped grey scale switching by helix deformation in planar alignment (DHF mode) [Citation123,Citation127,Citation128,Citation134] or the in-plane switching of the secondary optical axis in homeotropic alignment [Citation122,Citation124]. It is remarkable that the switching of the secondary optical axis in homeotropic samples is not only found in the Sm(CP)hel phase itself, but for all compounds TT/n in the SmCaPA ranges [Citation116,Citation118,Citation120]. As shown by RSoXS for TT/14 [Citation91] (see )) a residual short-range helix persists even in the SmCaPA phase (SmCaPA(hel)) adjacent to Sm(CP)hel. This is also responsible for the relatively low birefringence of the homeotropic sample (, texture at the left) [Citation92]. As an in-plane electric field with increasing voltage is applied to a homeoptropic cell, the birefringence increases first, then it rapidly goes through zero and then rises again (
Figure 16. (Colour online) Switching of the secondary director in the SmCaPA phases of compounds TT/n. (a) Electric-field dependence of the birefringence δn of TT/12 in a homeotropic cell under an applied in-plane electric field at different temperatures; reproduced with permission from ref. [Citation118], copyright 2012, Taylor & Francis (www.tandfonline.com). (b) shows models of a proposed molecular reorganization under the applied electric field, where the solid arrows indicate the directions of the secondary director in two adjacent layers (dashed arrows represent the next two layers) in a view parallel to the layer normal.
![Figure 16. (Colour online) Switching of the secondary director in the SmCaPA phases of compounds TT/n. (a) Electric-field dependence of the birefringence δn of TT/12 in a homeotropic cell under an applied in-plane electric field at different temperatures; reproduced with permission from ref. [Citation118], copyright 2012, Taylor & Francis (www.tandfonline.com). (b) shows models of a proposed molecular reorganization under the applied electric field, where the solid arrows indicate the directions of the secondary director in two adjacent layers (dashed arrows represent the next two layers) in a view parallel to the layer normal.](/cms/asset/2d42d104-135a-47bc-9257-605ae2480447/tlct_a_2010142_f0016_oc.jpg)
It is also emphasized that in planar samples, with the field applied between the top and bottom surfaces, the switching in the SmCaPA range takes place by rotation around the long axis, probably, because the local heliconical twist is suppressed in this alignment [Citation125] (however, in the Sm(CP)hel range the heliconical structure is retained in planar alignment, see ). This observation also suggests that the typical speckled ground state texture of the planar aligned SmCaPA phase (fan texture) might be interpreted as an indication of a residual driving force for twist between the layers, leading to an alternation of domains with an anchoring of either the polar axis or the bow plane parallel to the surfaces (90° jumps of the alignment of the secondary director in adjacent domains, see inset in )).
4.2. Comparison with related heliconical smectic and other mirror symmetry broken smectic and nematic phases
4.2.1. Relations to chiral LC phases of rod-like molecules
Commensurate heliconical phases with 3- or 4-layer pitch were previously known for permanently chiral rod-like molecules in the SmCFI1*, SmCFI2* phases, respectively, occurring at the synclinic-anticlinic (= FE-AF = SmCs*-SmCa*) transition of highly chiral mesogens [Citation131,Citation132]. Likewise, the incommensurate short pitch SmCα phase was found at the onset of uniform tilt at the SmA*-SmC* transition of these rod-like molecules [Citation136–138]. In this case the permanent molecular chirality, coupling with and biasing the helical molecular conformations is the main driving force of helix formation and the helix sense is fixed by the permanent molecular chirality [Citation139]. For the achiral, but transiently chiral BCLCs very similar heliconical phases appear at the FE-AF transition coinciding with the onset of uniform tilt and the synclinic-anticlinic transition. However, in the Sm(CP)hel phase the chirality appears as a broken symmetry. i.e. the developing helix sense is stochastic, assuming either helix sense with the same probability and thus leading to conglomerates (ambidextrous mirror symmetry breaking). Here the transient molecular conformational chirality as well as the escape from a developing polar order support the spontaneous helix formation if neither the synclinic nor the anticlinic structure can dominate.
4.2.2. Relations to the SmCTB and NTB phases of bent dimesogens
A non-polar analog of the heliconical Sm(CP)hel phase has been theoretically predicted [Citation140,Citation141] and recently found for bent mesogenic dimers below the NTB phase phase [Citation142]. This SmCTB phase represents a commensurate heliconical phase with 4-layer (SmCFI2*-like) structure with an overlaid larger twist [Citation143]. In other bent dimesogens a biaxial smectic phase is observed below the NTB phase, in which the individual mesogenic cores of the bent dimers are anticlinic tilted [Citation135,Citation143–145]. This phase is related to the SmCaPA phase occurring besides the Sm(CP)hel phase of the 4-cyanoresorcinol based BCLCs (see Fig. S2). The main difference is the polar order in SmCaPA, whereas there is obviously no polar order for the anticlinic smectic phases of the dimesogens.
The NTB phase involves only orientational order and heliconical self-assembly, as indicated by NMR spectroscopy [Citation146,Citation147], circular dichroism (CD) [Citation148] and RSoXS [Citation31,Citation149,Citation150]. There is theoretical work showing that the development of the helical twist is entropy driven and only requires a bent, but not any helical molecular conformation (axial chirality) [Citation140]. In line with this, the enantiomeric excess of the molecular conformations within the chiral domains of the conglomerate appears to be relatively small in the NTB phases [Citation151] (though it can become larger in other spontaneously chiral phases, as for example shown for the B4 phase [Citation152]). Nevertheless, there are diastereomeric relations between the transient conformational molecular and the helix chirality, leading to different energies for the diastereomeric pairs. Even if molecular chirality itself would not be required for emergence of chirality, it is likely to supports chirality synchronization and helical self-assembly of the molecules. This is in line with results obtained for heliconical phases by mean field theory [Citation141,Citation153]. Overall, chirality synchronization requires a certain coherence length of molecular order and intermolecular interactions that the free energy gain can exceed the critical value of ~2kT, as required for chirality synchronization in the liquid state [Citation79,Citation154,Citation155]. Therefore, in the nematic phase cybotaxis-like aggregation with increasing coherence length at reduced temperature might support chiral segregation by increasing the collective coupling of the individual molecules.
4.2.3. Relations to helical network phases
Helix formation and chirality synchronization were also found in LC network phases, as for example in the optically isotropic bicontinuous cubic (I23, previously known as ‘Im3m’ [Citation156]) and the birefringent 3D tetragonal (SmQ) LC phases [Citation157,Citation158], and it even leads to the mirror symmetry broken isotropic liquids of polycatenar molecules (Iso1[*]) [Citation155]. In the liquid conglomerates the branching of helical columns provides a long-range transmission of chirality by retaining short-range positional order in the Iso1[*] phase and after emergence of a long-range 3D lattice in the cubic (Cub[*]/I23) and non-cubic (SmQ) phases with 3D lattice, occurring at lower temperature [Citation79,Citation80,Citation159]. It is noted that already about 50 years ago, Ya. B. Zeldovich proposed the existence of a spontaneously chiral isotropic liquid representing the ‘minimal LC’ with minimal symmetry breaking (only space inversion) [Citation160,Citation161]. This chiral isotropic liquid phase [Citation79,Citation80] completes the sequence of spontaneously mirror symmetry broken LC phases,
Iso1[*] – NTB – SmTB – Sm(CP)hel,
occurring with decreasing phase symmetry by the stepwise emergence of orientational, translational and polar order. In all helical mesophases there is a preference for the side-by-side packing of the molecular/supramolecular helices with the same handedness. The dominance of this enantiophobic mode of chiral self-assembly is required for stochastic mirror symmetry breaking leading to the formation of conglomerates by ambidextrous mirror symmetry breaking [Citation79,Citation80,Citation162].
4.2.4. Enantiophilic vs. enantiophobic self-assembly
Interestingly, in crystalline assemblies the enantiophilic self-assembly with favourable interaction between opposite enantiomers and leading to racemates represent the dominating mode of self-assembly for mixtures of permanently or transiently chiral molecules, observed for >90 % of the cases. In contrast, the enantiophobic self-assembly with favourable interaction between like enantiomers and leading to conglomerates is rare [Citation163,Citation164]. Helical morphologies are typical for pure enantiomers and conglomerates, whereas the racemates usually form non-helical, often sheet-like structures in the solid state [Citation165]. In LC systems of transient chiral molecules or helical aggregates only the conglomerates are easily recognized by their chiral domain formation or helix formation and therefore dominate the discussion, whereas racemate formation is usually not recognized and their effect on LC self-assembly is therefore underestimated. An example is found in the bicontinuous cubic phases of polycatenar molecules, where enantiophilic packing of two enantiomorphous chiral gyroid networks leads to the achiral (racemic) double gyroid with Ia3d space group, whereas enantiophobic self-assembly gives rise to the uniformly chiral I23 cubic lattice. The transition between Ia3d and I23 takes place by modification of the alkyl chain length as well as depending on temperature [Citation79,Citation158].
4.3. Ferroelectric re-entrant SmA phase (SmALTPF)
An interesting feature of the long chain compounds of the series TT/n is that the layer spacing of the polar smectic phases increases on cooling more strongly than for those with shorter chains ()). This is in line with the experimentally observed decrease of the tilt angle with lowering temperature in the polar smectic phases of the compounds TT/n with n = 16–22 [Citation92]. Even a transition to a non-tilted smectic phase (SmALTPF) is found for these compounds [Citation126], leading to the unusual SmA-SmCs-SmALT phase sequence with a reentrance of the SmA phase on cooling. Formation of an SmA phase below SmCs has rarely been observed. The few known cases include permanently chiral rod-like molecules and bent mesogenic dimers [Citation166,Citation167]. For compounds TT/n the re-entrant SmA phase is polar. Because only one polarization current peak is observed under a triangular wave voltage, a uniformly polar nature of this SmALT phase is suggested (SmALTPF) [Citation92]. In the series of compounds TT/n formation of SmALTPF is associated with the anticlinic-synclinic transition upon growing chain length and the SmALTPF phase accompanies the heliconical Sm(CP)hel phase as a low temperature phase. There must be a specific reason for the very unusual decrease of the tilt upon chain elongation and lowering temperature. Here, it is postulated that in the Sm(CP)hel phase the enantiophobic packing, supporting chiral superstructures and conglomerate formation is favored, whereas in the SmALTPF range the enantiophilic packing becomes dominating, disfavouring helical and favouring achiral assemblies. This means that chiral phases, either heliconical smectic phases or tilted polar phases with intrinsic layer chirality become disfavoured. For compounds TT/n it leads to the removal of the tilt and a re-entrance of a SmA phase which is non-tilted and achiral. The transition from the chiral SmCsPF to an achiral leaning phase, described in Section 4.7, can be explained in a similar way, too. The change from enantiophobic to entantiophilic self-assembly could even play a role in the development of either the chiral twist–bend nematic (NTB) [Citation25,Citation25,Citation30–32] or achiral nematic or smectic phases, as for example the achiral splay (NS) and polar nematic phase (NF) [Citation168,Citation169,Citation170–172].
4.4. Surface supported mirror symmetry breaking by conglomerate formation in nematic phases (N[*])
Mirror symmetry breaking by conglomerate formation was occasionally observed in the nematic phases of BCLCs (N[*]) [Citation173,Citation174]. This was also found for the NCybC phases of some 4-cyanoresorcinol based BCLCs [Citation175,Citation176] and shown to be due to twisted states developing between the cell surfaces [Citation64,Citation175]. Though this surface supported mode of mirror symmetry breaking would not require any source of chirality, it is assumed to be supported by the pronounced transient helicity of bent-core molecules. Therefore, it can develop without application of any helical twist between the surfaces and even after linear shearing. The confinement of the molecules in the cybotactic clusters and the chiral SmCsPF structure of these clusters can couple with the transient molecular chirality, thus reinforcing its small effect in a cooperative way. The pronounced helicity of BCLC is also known to lead to an increased twisting power induced by chiral dopants, compared with standard rod-like nematic hosts [Citation162]. It is noted that there are alternative explanations only considering the elastic constants. A small twist elastic constants k22 [Citation177] or a small or negative bend elastic constant k33 are considered as origin of helix formation [Citation26,Citation30], especially if it takes place under confined conditions. In another model a chiral tetrahedral nematic phase with D2 symmetry was suggested [Citation178,Citation179] which might play a role for nematic phases of short chain BCLCs with low aggregation number or for clusters formed by pairs of bent mesogens [Citation176].
4.5. Surface supported mirror symmetry breaking by conglomerate formation in SmCs phases (SmCs[*] and SmCsPR[*])
A related conglomerate formation by surface supported mirror symmetry breaking is also found in the paraelectric SmCs phases designated as SmCs[*] and SmCsPR[*]. Thin cells with homeotropic alignment show only a very small birefringence, in line with a helix developing between the two surfaces and perpendicular to the layer planes. Under slightly uncrossed polarizers dark and bright domains can be distinguished which invert their brightness by inverting the direction of the analyzer, whereas rotation of the sample between the polarizers does not change the texture, confirmng a chiral conglomerate () [Citation98–101]. The conglomerate texture is lost at the transition to the SmCsPAR or SmCsPA phase with antipolar correlation, which are racemic. That these conglomerates cannot be observed in thick samples with reduced surface effects and in freely suspended films shows that this mirror symmetry breaking is surface supported, similar to that observed in the NCybC[*] phases. However, its formation is more easy reproduced than in the N[*] phases. This is presumably a result of the enhanced cooperativity provided by the quasi infinite layers in comparison to the cybotactic clusters. Moreover, there is a relation between the appearance of a broad single peak in the current response curves and conglomerate formation. Whereas conglomerates are typically observed in the SmCsPR[*] phases showing a single broad current peak under the triangular wave electric field, they are only rarely found for paraelectric SmCs[*] phases without detectable current peak. This means that also a growing coherence length of polar order has a stabilizing effect on conglomerate formation. This is in line with the suggested coupling between the emerging layer chirality and conformational molecular chirality [Citation79,Citation80].
Figure 17. (Colour online) Textures of the SmCS[*] phase of compound AAF/O20 at T = 130°C: (a,c) between slightly uncrossed polarizers, showing dark and bright domains, indicating the presence of areas with opposite chirality sense: (b) between crossed polarizers and (d, e) texture between crossed polarizers after rotation of the sample by 15° either clockwise or anticlockwise; the birefringence does not change which confirms chirality as origin of the effects seen in (a-c). Reprinted from ref. [Citation101], copyright 2016, Wiley-VCH.
![Figure 17. (Colour online) Textures of the SmCS[*] phase of compound AAF/O20 at T = 130°C: (a,c) between slightly uncrossed polarizers, showing dark and bright domains, indicating the presence of areas with opposite chirality sense: (b) between crossed polarizers and (d, e) texture between crossed polarizers after rotation of the sample by 15° either clockwise or anticlockwise; the birefringence does not change which confirms chirality as origin of the effects seen in (a-c). Reprinted from ref. [Citation101], copyright 2016, Wiley-VCH.](/cms/asset/06bb5a99-6d99-4704-9244-55e9210b0786/tlct_a_2010142_f0017_oc.jpg)
4.6. Dark conglomerate formation
Notably, mirror symmetry broken isotropic dark conglomerate (DC) type LC sponge phases and related crystalline helical nanofilament phases (HNF, B4) [Citation180,Citation181] as known for highly bent BCLCs, weakly bent oxadiazoles [Citation174,Citation182] and some oligomesogens [Citation183] are apparently missing in the series of 4-cyanoresorcinol based mesogens, even for compounds with silylated end-chains (see next Section) [Citation19,Citation184]. On the other hand, soft crystalline dark conglomerates (helical nano-crystallite phases, HNC) emerge and become dominating if the linear 4-CN group is replaced by bulky and more spherical 4-Br, 4-I or 4-CH3 substituents in the azobenzene based BCLCs [Citation185,Citation186,Citation187–190]. From DFT calculations it appears that the 4-CN group reduces the molecular bend (larger opening angle ψ), but induces a relatively large twist α between the π-planes of the wings (α = 86°, ) [Citation188]. This obviously retains flat layers and supports the development of a heliconical twist between the layers by the twist between the alkyl end-chain orientations, thus supporting the formation of the heliconical phases, namely the short pitch Sm(CP)hel phase and the surface stabilized long pitch heliconical states in the N[*] and SmCs(PR)[*] phases. However, this twist α obviously does not produce a sufficient transversal twist of the layers themselves, required for the formation of isotropic DC-type mesophases. This transversal twist obviously requires a stronger molecular bend, i.e. an opening angle ψ close to 120°. In addition, the bulkiness of the halogens and CH3 provide significant layer distortion, destabilizing the layers and allowing an easier layer deformation.
Figure 18. (Colour online) Energy minimum conformations obtained by DFT calculations for (a) the 4-bromo- and (b) 4-cyanoresorcinol based BCLCs with azobenzene wings; α = (90-α1)+(90-α2). Reprinted from ref. [Citation188], copyright 2014, RSC.
![Figure 18. (Colour online) Energy minimum conformations obtained by DFT calculations for (a) the 4-bromo- and (b) 4-cyanoresorcinol based BCLCs with azobenzene wings; α = (90-α1)+(90-α2). Reprinted from ref. [Citation188], copyright 2014, RSC.](/cms/asset/2f17fbf2-f8fa-4b30-bd65-4783330352eb/tlct_a_2010142_f0018_oc.jpg)
4.7. The leaning phase and ferroelectricity by terminal chain silylation
Silylation of bent-core mesogens by using oligosiloxane or carbosilane groups at one alkyl chain end is known as the most efficient tool to stabilize ferroelectric switching smectic phases in achiral BCLCs [Citation12, Citation19, Citation21–24, Citation191]. However, in most cases the developing SmCsPF phases escape from macroscopic polarization by layer modulation (B1 and B7 phases) [Citation40] or formation of sponge-like dark conglomerate (DC) phases, and only after surface stabilization between glass surfaces ferroelectric states can be found in some cases [Citation22]. However, for the terephthalate TT/Si () the birefringent SmCsPF phase is long time stable even as bulk material in the ground state and there is no relaxation to an antiferroelectric or DC phase, though the development of some layer modulation with short coherence length or long wave length (both being invisible in XRD) cannot fully be excluded. The formation of the SmCsPAR and SmCsPA phases with antipolar order is in this case inhibited by the nano-segregated layers of the carbosilanes. These layers inhibit the fluctuations of the molecules between the layers and thus remove the entropic advantage of the antipolar packing (), right) [Citation19,Citation21,Citation192].
Figure 19. (Colour online) Formula and phase transitions (T/°C) of the silylated compounds TT/Si and BT/Si and the structures of the different types of tilted phases of bent-core molecules [Citation184].
![Figure 19. (Colour online) Formula and phase transitions (T/°C) of the silylated compounds TT/Si and BT/Si and the structures of the different types of tilted phases of bent-core molecules [Citation184].](/cms/asset/399df5e0-459c-4b7f-8b89-cb90ebb607a2/tlct_a_2010142_f0019_oc.jpg)
In contrast, the isomeric compound BT/Si differing only in the direction of one of the COO groups and combining only one terephthalate unit with a phenylbenzoate unit shows a weaker segregation, and the silyl group mainly acts as a bulky substituent reducing the coherence length of polar order. Therefore, the polar domain size in the SmA and SmCs phases is only small, not showing a visible polarization peak, though there is mirror symmetry breaking by conglomerate formation in the SmCs[*] phase. BT/Si also tends to assume synpolar order in the layers, but surprisingly, the tilt direction coincides with the polar direction in this ferroelectric smectic phase, providing the first case of a so-called leaning phase SmCLsPF ()) [Citation184,Citation193]. This is a new type of polar smectic C phase complementing the previously known SmCsPF phase with orthogonal directions of tilt and polar order and the SmCGPA/F phases [Citation194–196] with inclined directions of tilt/polar directions by the new syn-leaning SmCLsPF phase with coincidence of tilt and polar directions ().
Due to the coincidence of polar and tilt directions, the leaning phases are achiral [Citation197]. It is remarkable that achiral phases, SmALTPF for compounds TT/n and SmCLsPF for BT/Si, are formed at relatively low temperature though the packing becomes denser and synchronization of molecular conformations is likely to become more important for self-assembly. A possible explanation for the transition from the usually observed polar SmC phases of bent-core molecules, with chiral layers (due to the orthogonal combination of polar direction and tilt direction) to the achiral SmALTPF and SmCLsPF phases could be that the enantiophilic self-assembly (racemate formation) becomes advantageous over the enantiophobic (conglomerate formation), as explained in Section 4.3. Due to the bulky silyl groups BT/Si has a stronger tendency to retain tilt than the non-silylated compounds of series BT/n and TT/n. Their tilt cannot be removed, and therefore removal of the inclination between tilt and polar direction by formation of the leaning SmCLsPF phase becomes the preferred alternative.
4.8. Effects of branched chains
Branched chains reduce the packing density of the bent aromatic rods, i. e. phase transitions as well as SmCsPF domain formation and their growth and fusion to polar layers are shifted to lower temperatures. It also appears, that due to the reduced packing density even the polar smectic phases do not represent classical B2 phases with quasi infinite polar order in the layers, but the layers still retain a significant polar domain structure which is indicated by the apostrophe in the phase abbreviations (SmCs’PA). ) shows the temperature dependent development of the layer spacing in the smectic phases of compound rac-TT/Ocit with two branched 3,7-dimethyloctyloxy end-chains derived from rac-citronellol (black squares) [Citation198]. The decrease of the layer spacing d in the uniaxial SmA range between 110 and 95°C can be attributed to the emergence and growth of a random tilt due to the growth of SmCsPF domains and therefore at least this range of the uniaxial SmA phase can be considered as a de Vries like tilted smectic phase. The polar domain structure is retained in the biaxial SmC phase. That the phase is biaxial though no optical tilt can be identified in the absence of an applied E-field (), red) means that the synpolar and synclinic alignment of the SmCsPF domains becomes on average antipolar and anticlinic and in this respect this phase can be considered as a de Vries like SmAb phase [Citation198]. Application of a small E-field induces a significant tilt due to the alignment of the SmCsPF domains. This field induced tilt by domain alignment can be considered as a non-classical electroclinic effect which is also observed in the de Vries SmA phase range, where it requires larger E-fields (), black).
Figure 20. (Colour online) (a) Temperature dependence of the d-value of the small angle (black) and the maxima of the diffuse wide-angle scattering (red) in the paraelectric mesophases of rac-TT/Ocit (under these conditions crystallization takes place before the transition to the polar phase); (b) field strength dependence of the optical tilt angle β of rac-TT/Ocit in the paraelectric smectic phases. Reprinted from ref. [Citation198], copyright 2016, RSC.
![Figure 20. (Colour online) (a) Temperature dependence of the d-value of the small angle (black) and the maxima of the diffuse wide-angle scattering (red) in the paraelectric mesophases of rac-TT/Ocit (under these conditions crystallization takes place before the transition to the polar phase); (b) field strength dependence of the optical tilt angle β of rac-TT/Ocit in the paraelectric smectic phases. Reprinted from ref. [Citation198], copyright 2016, RSC.](/cms/asset/4ec7ca57-42b7-435a-8450-175181e79f38/tlct_a_2010142_f0020_oc.jpg)
It is noted that, in addition, the branching of the molecules contributes to the stabilization of the ferreoelectric order due to the layer decoupling effect of the bulkier and more disordered branched chains [Citation199,Citation200]. Moreover, chain branching can create stereogenic centers leading to permanent molecular chirality which can be uniform or racemic.
5. Effects of permanent molecular chirality
5.1. Non-classical electroclinic effect and switching modes
Permanent molecular chirality is known to reduce the phase symmetry of tilted smectic phases and thus was found to induce ferroelectric switching in SmCs and antiferroelectric switching in SmCa phases of rod-like molecules [Citation130,Citation131]. Alternatively, the reduction of phase symmetry is achieved by the polar order developing in the layers of the smectic phases of bent-core molecules, which thus become chiral and show ferroelectric or antiferroelectric switching, in this case independent on the mode of tilt correlation. The effects of the permanent molecular chirality on the mesophases of weakly bent mesogens was studied by comparing the enantiomeric pure compound (S)-TT/Ocit with its racemate rac-TT/Ocit [Citation198]. In the de Vries SmA* range of (S)-TT/Ocit () there is a significant electroclinic effect already at low field strength ()), meaning that the chiral SmCsPF domains with one specific sense of chirality are stabilized by the diastereomeric coupling with the permanent molecular chirality, thus supporting uniform polar order ()). As a result of this diastereomeric coupling the double current peak in the SmCsPAR range of the racemate is replaced by a single peak in the SmCsPR* range of the enantiomer. Moreover, it changes the switching from a superparaelectric-like switching in the SmCs’PA range of the racemate to ferroelectric in the corresponding SmCs’PF* phase range of the enantiomer ()) [Citation198]. This means, that for weakly bent BCLC the synpolar order is supported by the molecular chirality, whereas for more strongly bent molecules the spatially induced polar order (polar packing of the bend directions) becomes dominating, which usually prefers antiferroelectric phases even in the presence of molecular chirality and if the tilt is synclinic [Citation198].
Figure 21. (Colour online) (a) Field strength dependence of the optical tilt of (S)-TT/Ocit in the distinct paraelectric smectic phases and; b,c) switching current response curves in the polar smectic phases of racemate and enantiomer at the same temperature (75°C); the (anti)ferroelectric character of switching in (b) is additionally confirmed by electrooptical investigations. The slope of the β = f(E) curve in the SmC* phase of (S)-TT/Ocit is smaller than in the SmC phase of the racemate rac-TT/Ocit (compare )) due to helix unwinding. Reprinted from ref. [Citation198], copyright 2016, RSC.
![Figure 21. (Colour online) (a) Field strength dependence of the optical tilt of (S)-TT/Ocit in the distinct paraelectric smectic phases and; b,c) switching current response curves in the polar smectic phases of racemate and enantiomer at the same temperature (75°C); the (anti)ferroelectric character of switching in (b) is additionally confirmed by electrooptical investigations. The slope of the β = f(E) curve in the SmC* phase of (S)-TT/Ocit is smaller than in the SmC phase of the racemate rac-TT/Ocit (compare Figures 20(a) and 21(a)) due to helix unwinding. Reprinted from ref. [Citation198], copyright 2016, RSC.](/cms/asset/2cb15d4d-d6c5-401a-9842-3c01f855ebfb/tlct_a_2010142_f0021_oc.jpg)
Table 2. Mesophases and phase transition temperatures of the branched chiral compounds with (S)-configuration and their racemic mixtures (rac) [Citation90,Citation198]. a
Interestingly, between 73 and 81°C, in the achiral SmCs’PA phase range of rac-TT/Ocit (), the switching takes place by collective rotation around the long axis as typical for the non-helical LC phases of the 4-cyanoresorcinols, whereas the corresponding chiral SmCs’PF* phase of the (S)-enantiomer switches by collective precession on a cone which retains the layer chirality [Citation198]. Thus, the effect of permanent molecular chirality is the same as that of the spontaneously broken symmetry in the Sm(CP)hel phase of the related achiral compounds TT/n with linear alkyl chains (see section 4.1). This indicates a significant diastereomeric coupling between molecular and helical chirality on the one hand, and the layer chirality on the other hand, i.e. the energetically favored couple between molecular/helical and layer chirality has to be retained during the switching process. However, below 73°C, after transition to the anticlinic phase, the switching takes place by precession on a cone for both, the racemate (SmCa’PA) and the (S)-enantiomer (SmCa’PA*), meaning that the bent molecular shape becomes dominating and molecular chirality is no more required for inhibition of the rotation around the long axis. Thus, in the SmCa’PA(*) phase of the compounds with branched chains the switching takes place by precession on a cone, whereas in the SmCaPA phase of the related achiral compounds TT/n with linear alkyl chains the molecules rotate collectively around the long axis. This is remarkable, because the opposite would be expected, i.e. the branched chains are expected to lead to a reduced packing density of the cores and therefore should support the rotation around the long axis (planar alignment in both cases). A possible explanation could be that a local segregation of the enantiomers takes place, which leads to a kind of nanoscale conglomerate and this local chirality provides an energetic penalty for the transition to an achiral phase structure, thus disfavouring the rotation around the long axis.
5.2. Diastereomerism between superstructural and permanent molecular chirality
In contrast, in the SmCsPA phase of the biphenyl compound PP/Ocit with a shorter bent core unit the switching takes place by collective rotation around the long axis for the racemate as well as for the (S)-enantiomer (
Figure 22. (Colour online) Textures as observed for the SmCsPA* phase of compound (S)-PP/Ocit under an applied DC field at T = 43°C; in a 6 μm polyimide coated ITO cell with planar alignment; (c) and (h) were taken immediately after field reversal, whereas (d-f) and (i, j) were taken with a time difference of 20 s between each photo; it is noted that the birefringence at 0 V in (b) and (g) is much lower than under the applied field (see SI for a T-dependence of Δn). (k) shows the origin of layer chirality [Citation112] and (l) shows the energy diagram of the diastereomeric coupling between molecular chirality and layer chirality. Reprinted with modifications from ref. [Citation90], copyright 2016, RSC.
![Figure 22. (Colour online) Textures as observed for the SmCsPA* phase of compound (S)-PP/Ocit under an applied DC field at T = 43°C; in a 6 μm polyimide coated ITO cell with planar alignment; (c) and (h) were taken immediately after field reversal, whereas (d-f) and (i, j) were taken with a time difference of 20 s between each photo; it is noted that the birefringence at 0 V in (b) and (g) is much lower than under the applied field (see SI for a T-dependence of Δn). (k) shows the origin of layer chirality [Citation112] and (l) shows the energy diagram of the diastereomeric coupling between molecular chirality and layer chirality. Reprinted with modifications from ref. [Citation90], copyright 2016, RSC.](/cms/asset/80e94f82-56dd-4f93-b480-a4622bd56d83/tlct_a_2010142_f0022_c.jpg)
5.3. Giant magnetic field effects
Another interesting and unprecedented chirality effect was observed in the smectic phases of BB/12-cit having only one branched chiral chain and an achiral linear chain at the opposite end () [Citation202]. For this compound huge magnetic field effects on the layer spacing were found with an opposite effect of the magnetic field on racemate and pure enantiomer. Whereas a layer expansion by 0.2–0.4 nm is observed for the racemate ()), a layer shrinkage by up to 0.6 nm is found for the enantiomer under an applied magnetic field of only one Tesla ()). This is explained as an effect of the magnetic field on the core order parameter. In the racemate the field induced increase of the orientational order parameter leads to a denser core packing; this increases the alkyl chain order and decreases the possibility of chain intercalation which in turns rises the layer spacing ()). For the enantiomeric pure compound, the heliconical (longitudinal) twist allows an optimized packing of the transiently helical molecules with a preferred helix sense due to the coupling of permanent molecular and transient conformational chirality. This means that already without applied magnetic field the chains are in the most stretched conformation and the layer spacing at 90°C corresponds to that observed for the racemate under the magnetic field [Citation202]. It is known that the magnetic field has a stronger suppressing effect on the longitudinal than on the transversal twist and this at first removes the heliconical (longitudinal) twist [Citation203,Citation204]. Because the chirality of the molecules is permanent, conformational helicity has to be preserved. Therefore, the longitudinal twist cannot simply be removed, but it is replaced by a developing transversal twist within the layers. This transversal twist distorts the layers, reduces the order parameter and hence the packing density. This provides more space between the alkyl chains for chain folding or intercalation, thus reducing the layer spacing under the applied magnetic field ()) [Citation202].
Figure 23. (Colour online) (a,b) Effects of the magnetic field on the orientational order parameter and layer distance of the racemic and uniformly chiral compound BB/O12-cit; (c,d) show the d-values of the SAXS depending on temperature and magnetic field [Citation202].
![Figure 23. (Colour online) (a,b) Effects of the magnetic field on the orientational order parameter and layer distance of the racemic and uniformly chiral compound BB/O12-cit; (c,d) show the d-values of the SAXS depending on temperature and magnetic field [Citation202].](/cms/asset/1f54420a-f49e-45a1-96da-df5e7bc4fbb4/tlct_a_2010142_f0023_oc.jpg)
It appears that this strong magnetic effect is related to the non-symmetric molecular structure of the molecule having one branched and one linear chain, which provides just the right chain volume for a transition between intercalated and non-intercalated smectic phases.
A related TT-based compound with one chiral lactate side chain was recently reported to show a ferroelectric-like switching in a uniaxial smectic phase, but no comparison with the racemate was made in this case [Citation205].
5.4. Helical superstructure and chirality frustrated LC phases
Besides the above-mentioned chirality effects, the molecular chirality affects the phase structure in the usual way by introducing helical twist. In contrast to rod-like molecules where strong chirality is required to obtain new chirality modulated phases, for the bent molecules even stereogenic centers having a weak helical twisting power are sufficient to induce highly frustrated LC phases. For example, compound (S)-BB/Ocit shows a broad BPIII phase (blue fog phase) with unusually large Kerr constants () [Citation90,Citation206]. Different types of blue phases were also induced in the nematic phases of achiral 4-cyanoresorcinols by addition of chiral dopants, and in nematic/cholesteric phases of rod-like molecules by adding 4-cyanoresorcinol derived BCLCs, indicating their amplification effect on helicity [Citation207–209]. This is also evidenced by the formation of the highly frustrated Twist Grain Boundary phases (TGBA and TGBC) by the 4-cyanoresorcinol BP/Oi-am with a weakly chiral isoamyl group at only one end [Citation210]. This indicates the much stronger effects of permanent molecular and transient conformational chirality on the self-assembly of BCLCs compared to linear mesogens.

It is noted here, that the first example of TGB phases of bent-core mesogens [Citation211] was observed by Sadashiva et al. and he was also involved in the report about induction of BPIII phases by addition of bent-core mesogens to cholesteric phases of rod-like molecules [Citation212], once again showing his seminal contributions to different aspects of LC research.
6. Hockey-stick molecules, dimesogens and oligomesogens
Besides the bent-core molecules with two polyaromatic wings of almost identical length also related hockey stick molecules, having a different length of the wings have been reported [Citation213]. Moreover, some end-connected homo- and heterodimers [Citation214,Citation215] as well as different types of laterally connected heterodimers combining 4-cyanoresorcinol based BCLCs with rod-like mesogens have been described [Citation216]. It is noted that some dimesogens (e.g. Di3) can show especially broad regions of the cybotactic nematic phase [Citation215]. Three selected examples of dimesogens are shown in
, among them compound Di3 combining the 4-cyanoresorcinol core with a 4-cyanobiphenyl (CB) unit. It is noted that the CB unit was also combined with other bent cores leading to broad cybotactic nematic phase regions [Citation217,Citation218] eventually involving the NTB phase [Citation218]. However, polymeric, dendrimeric and other larger molecular structure involving the 4-cyanoresorcinol unit have not been investigated so far.7. Summary
The current state of knowledge of the LC self-assembly of 4-cyanoresorcinol based BCLC is reviewed. The 4-cyano group at the central 1,3-substituted benzene ring of bent-core molecules causes a reduced molecular bend and thus provides compounds at the cross-over between classical rod-like and typical bent-core mesogens. This gives rise to at least five new LC phase structures (SmCsPR, SmCsPAR, SmALTPF, SmCLsPF, Sm(CP)hel). The CN group also supports the formation of cybotactic nematic phases (NCybC, NCybA). Lowering the temperature or lengthening the terminal alkyl chains leads to an almost continuous transition between these nematic and smectic phases by the growth of the cybotactic SmC clusters. Within the smectic phases, a continuous transition takes place from the apolar de Vries-like SmA and the SmCs phases via paraelectric and superparaelectric ranges (SmAPR, SmCsPR[*], SmAPAR, SmCsPAR) and finally to polar smectic phases (SmCsPA, SmCaPA) by the growth of polar SmCsPF domains. As the polar order becomes long-range, a series of new LC phases emerges just before the transition to the antiferroelectric phases. Among them, modulated smectic phases (M1, SmsPA) for compounds had a strong tendency to form tilted phases and a new heliconical smectic phase (Sm(CP)hel) if there is reduced tilt. The heliconical phase occurs at the SmCaPA– SmCsPA transition and either replaces the chiral SmCaPA phase as ground state structure or the racemic SmCsPA phase as an E-field induced long-time stable phase. It is considered as analogous to the SmCα* phase found for permanently chiral rod-like molecules.
It is also shown that diastereomerism arising between the molecular chirality and the superstructural helical and layer chiralities has a significant influence on the mesophase structures and the switching behavior. It is postulated that the change from enantiophobic (conglomerate forming) to enantiophilic (racemate forming) self-assembly contributes to the unusual transition from the tilted polar smectic phases with intrinsic layer chirality to alternative achiral polar phases, either being non-tilted (SmALTPF) or assuming a leaning-type tilt (SmCLsPF).
The heliconical Sm(CP)hel phase is of practical interest for fast switching electro-optical devices, either using the in-plane switching of the secondary optical axis or the V-shaped grey scale switching by helix deformation. A local helical organization is even retained in the adjacent SmCaPA phase, which also shows a fast switching of the secondary optical axis. The ferroelectric switching in the non-tilted SmALTPF and the leaning phase and the strong electroclinic and magnetic field effects of the permanently chiral 4-cyanoresorcinols provide additionial applications.
Overall, 4-cyanoresorcinol based BCLC represent a unique class of compounds providing a variety of new phenomena, phase structures, effects and applications and thus improve our basic understanding of complex self-assembly in LC and other soft matter systems.
Supporting_Information2_revised1.pdf
Download PDF (6.2 MB)Acknowledgments
The work reviewed in this manuscript is the result of several Master’s and PhD students (C. Keith, A. Lehmann, E. Westphal, M. Poppe), postdocs (S. Poppe, M. Prehm) and guest researchers (M. Alaasar, G. Shanker) over the recent decade working at Halle University and the collaboration with numerous scientists and their groups from different other places (N. Clark, Boulder; M.K. Das, Siliguri; A. Eremin, Magdeburg; J. Lagerwall, Luxemburg; F. Liu, Xi’an; H. Ocak and B. Bilgin-Eran, Istanbul and J. K. Vij, Dublin). Special thanks to M. Alaasar and H. Ocak, who designed and synthesized the azobenzene-based and chiral BCLCs, respectively, and to J. K. Vij who has supervised most of the electrooptical investigations and switching experiments. The authors work in this area has been supported by the EU with the FP7 funded collaborative project BIND (216025) and the Deutsche Forschungsgemeinschaft (Ts 39/24-1/2).
Disclosure statement
No potential conflict of interest was reported by the author(s).
Supplementary material
Supplemental data for this article can be accessed here
Additional information
Funding
References
- Chandrasekhar S, Sadashiva BK, Suresh KA. Liquid crystals of disc-like molecules. Pramana. 1977;9:471–480.
- Madhusudana NV, Sadashiva BK, Moodithaya KPL. Re-entrant nematic phase in pure compound at atmospheric pressure. Curr Sci. 1979;48:613–614.
- Chandrasekhar S, Ratna BR, Sadashiva BK, et al. A thermotropic biaxial nematic liquid crystal. Mol Cryst Liq Cryst. 1988;165:123–130.
- Chandrasekhar S, Sadashiva BK, Srikanta BS. Paramagnetic nematic liquid crystals. Mol Cryst Liq Cryst. 1987;15:93–107.
- Reddy RA, Sadashiva BK. Influence of fluorine substituent on the mesomorphic properties of five-ring ester banana-shaped molecules. Liq Cryst. 2003;30:1031–1050.
- Reddy RA, Raghunathan VA, Sadashiva BK. Novel ferroelectric and antiferroelectric smectic and columnar mesophases in fluorinated symmetrical bent-core compounds. Chem Mater. 2005;17:274–283.
- Reddy RA, Sadashiva BK. New phase sequences in banana-shaped mesogens: influence of fluorine substituent in compounds derived from 2,7-dihydroxynaphthalene. J Mater Chem. 2004;14:1936–1947.
- Reddy RA, Sadashiva BK. Direct transition from a nematic phase to a polar biaxial smectic A phase in a homologous series of unsymmetrically substituted bent-core compounds. J Mater Chem. 2004;14:310–319.
- Reddy RA, Sadashiva BK, Pratibha R, et al. Biaxial smectic A phase in homologous series of compounds composed of highly polar unsymmetrically substituted bent-core molecules. J Mater Chem. 2002;12:943–950.
- Murthy HNS, Sadashiva BK. A polar biaxial smectic A phase in new unsymmetrical compounds composed of bent-core molecules. Liq Cryst. 2004;31:567–578.
- Guo L, Dhara S, Sadashiva BK, et al. Polar switching in the smectic-AdPA phase composed of asymmetric bent-core molecules. Phys Rev E. 2010;81:011703.
- Dantlgraber G, Eremin A, Diele S, et al. Chirality and macroscopic polar order in a ferroelectric smectic liquid crystalline phase formed by achiral polyphilic bent-core molecules. Angew Chem Int Ed. 2002;41:2408–2412.
- Amaranatha RA, Sadashiva BK. Ferroelectric properties exhibited by mesophases of compounds composed of achiral banana-shaped molecules. J Mater Chem. 2002;12:2627–2632.
- Pratibha R, Madhusudana NV, Sadashiva BK. An orientational transition of bent-core molecules in an anisotropic matrix. Science. 2000;288:2184–2187.
- Hegmann T, Kain J, Diele S, et al. Evidence for the existence of the mcmillan phase in a binary system of a metallomesogen and 2,4,7-Trinitrofluorenone. Angew Chem Int Ed. 2001;40:887–890.
- Gray GW, Harrison KJ, Nash JA. New family of nematic liquid crystals for displays. Electron Lett. 1973;9:130–131.
- Sadashiva BK, Reddy RA, Pratibha R, et al. Biaxial smectic A liquid crystal in a pure compound. Chem Commun. 2001;2140–2141.
- Sadashiva BK, Reddy RA, Pratibha R, et al. Biaxial smectic A phase in homologous series of compounds composed of highly polar unsymmetrically substituted bent-core molecules. J Mater Chem. 2002;12:943–950.
- Keith C, Reddy RA, Hauser A, et al. Silicon-containing polyphilic bent-core molecules: the importance of nanosegregation for the development of chirality and polar order in liquid crystalline phases formed by achiral molecules. J Am Chem Soc. 2006;126:3051–3066.
- Keith C, Reddy RA, Tschierske C. The first example of a liquid crystalline side-chain polymer with bent-core mesogenic units: ferroelectric switching and spontaneous achiral symmetry breaking in an achiral polymer. Chem Commun. 2005;871–873.
- Keith C, Reddy RA, Hahn H, et al. The carbosilane unit as a stable building block for liquid crystal design: a new class of ferroelectric switching banana-shaped mesogens. Chem Commun. 2004;1898–1899.
- Reddy RA, Tschierske C. Bent-core liquid crystals: polar order, superstructural chirality and spontaneous desymmetrisation in soft matter systems. J Mater Chem. 2006;16:907–961.
- Zhang Y, Baumeister U, Tschierske C, et al. Achiral bent-core molecules with a series of linear or branched carbosilane termini: dark conglomerate phases, supramolecular chirality and macroscopic polar order. Chem Mater. 2010;22:2869–2884.
- Reddy RA, Zhu C, Shao R, et al. Spontaneous ferroelectric order in a bent-core smectic liquid crystal of fluid orthorhombic layers. Science. 2011;332:72–77.
- Meyer RB. Structural problems in liquid crystal physics, molecular fluids. In: Balian RG, and Weil Geditors. Les houches summer school in theoretical physics. New York (NY): Gordon and Breach; 1976. p. 271–343.
- Dozov I. On the spontaneous symmetry breaking in the mesophases of achiral banana-shaped molecules. Europhys Lett. 2001;56:247–253.
- Panov VP, Nagaraj M, Vij JK, et al. Spontaneous periodic deformations in nonchiral planar aligned bimesogens with a nematic-nematic transition and a negative elastic constant. Phys Rev Lett. 2010;105:167801.
- Cestari M, Diez-Berart S, Dunmur DA, et al. Phase behavior and properties of the liquid-crystal dimer 1″,7″-bis (4-cyanobiphenyl-4ʹ-yl) heptane: a twist-Bend nematic liquid crystal. Phys Rev E. 2011;84:031704.
- Henderson PA, Imrie CT. Methylene-linked liquid crystal dimers and the twist-Bend nematic phase. Liq Cryst. 2011;38:1407–1414.
- Borshch V, Kim YK, Xiang J, et al. Nematic twist-Bend phase with nanoscale modulation of molecular orientation. Nat Commun. 2013;4:2635.
- Chen D, Porada JH, Hooper JB, et al. Chiral heliconical ground state of nanoscale pitch in a nematic liquid crystal of achiral molecular dimers. Proc Natl Acad Sci USA. 2013;110:15931–15936.
- Mandle RJ, Davis EJ, Archbold CT, et al. Microscopy studies of the nematic NTB phase of 1,11-di-(100-cyanobiphenyl-4-yl)undecane. J Mater Chem C. 2014;2:556–566.
- Paterson DA, Abberley JP, Harrison WTA, et al. Cyanobiphenyl-based liquid crystal dimers and the twist-Bend nematic phase. Liq Cryst. 2017;44:127–146.
- Mandle RJ, Goodby JW. Molecular flexibility and bend in semi-rigid liquid crystals: implications for the heliconical nematic ground state. Chem Eur J. 2019;25:14454–14459.
- Mandle JM. The dependency of twist-Bend nematic liquid crystals on molecular structure: a progression from dimers to trimers, oligomers and polymers. Soft Matter. 2016;12:7883–7901.
- Reddy RA, Sadashiva BK. Helical superstructures in the mesophase of compounds derived from 2-cyanoresorcinol. Liq Cryst. 2002;29:1365–1367.
- Murthy HNS, Sadashiva BK. Banana-shaped mesogens: a new homologous series of compounds exhibiting the B7 mesophase. Liq Cryst. 2003;30:1051–1055.
- Reddy RA, Sadashiva BK. Unusual mesomorphic behaviour in bent-core compounds derived from 5-cyanoresorcinol. Liq Cryst. 2004;31:1069–1081.
- Pelzl G, Diele S, Jakli A, et al. Helical superstructures in a novel smectic mesophase formed by achiral banana-shaped molecules. Liq Cryst. 2006;33:1519–1523.
- Coleman DA, Fernsler J, Chattham N, et al. Polarization-modulated smectic liquid crystal phases. Science. 2003;301:1204–1211.
- Weissflog W, Kovalenko L, Wirth I, et al. SmA–SmC–B2 polymorphism in an achiral cyano substituted banana-shaped mesogen. Liq Cryst. 2000;27:677–681.
- Dunemann U, Schröder MW, Reddy RA, et al. The influence of lateral substituents on the mesophase behaviour of bananashaped mesogens. Part II. J Mater Chem. 2005;15:4051–4061.
- Kovalenko L, Schröder MW, Reddy RA, et al. Unusual mesomorphic behaviour of new bent-core mesogens derived from 4-cyanoresorcinol. Liq Cryst. 2005;32:857–865.
- Pelzl G, Weissflog W. Mesophase behaviour at the borderline between calamitic and banana-shaped mesogens. In: Ramamoorthy A, editor. Thermotropic Liquid Crystals: Recent Advances. The Netherlands: Springer; 2007. p. 1–58.
- Weissflog W, Murthy HNS, Diele S, et al. Relationships between molecular structure and physical properties in bent-core mesogens. Phil Trans Royal Soc A. 2006;364:2657–2679.
- Eremin A, Diele S, Pelzl G, et al. Experimental evidence for an achiral orthogonal biaxial smectic phase without in-plane order exhibiting antiferroelectric switching behavior. Phys Rev E. 2001;64:051707.
- Pan L, McCoy BK, Wang S, et al. Surface and bulk uniaxial to biaxial smectic-A transition in a bent core liquid crystal. Phys Rev Lett. 2010;105:117802.
- Pociecha D, Gorecka E, Čepič M, et al. Polar order and tilt in achiral smectic phases. Phys Rev E. 2006;74:021702.
- Keith C, Lehmann A, Baumeister U, et al. Nematic phases of bent-core mesogens. Soft Matter. 2010;6:1704–1721.
- Pelzl G, Diele S, Weissflog W. Banana-shaped compounds-A new field of liquid crystals. Adv Mater. 1999;11:707–724.
- Takezoe H, Takanishi Y. Bent-core liquid crystals: their mysterious and attractive world. Jpn J Appl Phys. 2006;45:597–625.
- Takezoe H, Eremin A. Bent-shaped Liquid Crystals. Structures and Physical Properties. Boca Raton: CRC Press; 2017.
- Tschierske C, Photinos DJ. Biaxial nematic phases. J Mater Chem. 2010;20:4263–4294.
- Tschierske C, Zaschke H. A mild and convenient esterification of sensitive carboxylic acids. J prakt Chem. 1989;331:365–366.
- Shanker G, Nagaraj M, Kocot A, et al. Nematic phases in 1,2,4-oxadiazole-based bent-core liquid crystals: is there a ferroelectric switching? Adv Funct Mater. 2012;22:1671–1683.
- Vita F, Adamo FC, Francescangeli O. Polar order in bent-core nematics: an overview. J Mol Liq. 2018;267:564–573.
- Vita F, Adamo FC, Pisani M, et al. Nanostructure of unconventional liquid crystals investigated by synchrotron radiation. Nanomaterials. 2020;10:1679.
- Jakli A. Liquid crystals of the twenty-first century – nematic phase of bent-core molecules. Liq Cryst Rev. 2013;1:65–82.
- de Vries A. X-ray photographic studies of liquid crystals I. A cybotactic nematic phase. Mol Cryst Liq Cryst. 1970;10:219–236.
- Hong SH, Verduzco R, Williams JC, et al. Short-range smectic order in bent-core nematic liquid crystals. Soft Matter. 2010;6:4819–4827.
- Ghilardi M, Adamo FC, Vita F, et al. Comparative 2H NMR and X-ray dffraction investigation of a bent-core liquid crystal showing a nematic phase. Crystals. 2020;10:284.
- Shanker G, Prehm M, Nagaraj M, et al. 1,2,4-oxadiazole-based bent-core liquid crystals with cybotactic nematic phases. ChemPhysChem. 2014;15:1323–1335.
- Francescangeli O, Vita F, Samulski ET. The cybotactic nematic phase of bent-core mesogens: state of the art and future developments. Soft Matter. 2014;10:7685–7691.
- Gleeson HF, Kaur S, Görtz V, et al. The nematic phases of bent-core liquid crystals. ChemPhysChem. 2014;15:1251–1260.
- Jang Y, Panov VP, Kocot A, et al. Short-range correlations seen in the nematic phase of bent-core liquid crystals by dielectric and electro-optic studies. Phys Rev E. 2011;84:060701(R).
- Panarin YP, Sreenilayam SP, Vij JK, et al. Formation and development of nanometer-sized cybotactic clusters in bent-core nematic liquid crystalline compounds. Beilstein J Nanotechnol. 2018;9:1288–1296.
- Vaupotic N, Szydlowska J, Salamonczyk M , et al. Structure studies of the nematic phase formed by bent-core molecules.Phys Rev E. 2009;80:030701.
- Zhang C, Gao M, Diorio N, et al. Direct observation of smectic layers in thermotropic liquid crystals. Phys Rev Lett. 2012;109:107802.
- Gao M, Kim Y-K, Zhang C, et al. Direct observation of liquid crystals using cryo-TEM: specimen preparation and low-dose imaging. Mic Res Technol. 2014;77:754–772.
- Chakraborty A, Das MK, Das B, et al. Rotational viscosity measurements of bent-core nematogens. Soft Matter. 2013;9:4273–4283.
- Balachandran R, Panov VP, Vij JK, et al. Effect of cybotactic clusters on the elastic and flexoelectric properties of bent-core liquid crystals belonging to the same homologous series. Phys Rev E. 2013;88:032503.
- Sreenilayam SP, Panarin YP, Vij JK, et al. Flexoelectric polarization studies in bent-core nematic liquid crystals. Phys Rev E. 2015;92:022502.
- Madhuri PL, Hiremath US, Yelamaggad CV, et al. Influence of virtual surfaces on Frank elastic constants in a polymer-stabilized bent-core nematic liquid crystal. Phys Rev E. 2016;93:042706.
- Cestari M, Frezza E, Ferrarini A, et al. Crucial role of molecular curvature for the bend elastic and flexoelectric properties of liquid crystals: mesogenic dimers as a case study. J Mater Chem. 2011;21:12303–12308.
- Varshini GV, Rao DSS, Hiremath US, et al. Dielectric and viscoelastic investigations in a binary system of soft- and rigid-bent mesogens exhibiting the twist-Bend nematic phase. J Mol Liq. 2012;323:114987.
- Tadapatri P, Hiremath US, Yelamaggad CV, et al. Patterned electroconvective states in a bent-core nematic liquid crystal. J Phys Chem B. 2010;114:10–21.
- Krishnamurthy KS, Tadapatri P, Viswanatha P. Dislocations and metastable chevrons in the electroconvective in plane normal roll state of a bent core nematic liquid crystal. Soft Matter. 2014;10:7316–7327.
- Jang Y, Panov VP, Keith C, et al. Sign reversal in the dielectric anisotropy as functions of temperature and frequency in the nematic phase of a bent-core liquid crystal. Appl Phys Lett. 2010;97:152903.
- Tschierske C, Ungar G. Mirror symmetry breaking by chirality synchronisation in liquids and liquid crystals of achiral molecules. ChemPhysChem. 2016;17:9–26.
- Tschierske C. Mirror symmetry breaking in liquids and liquid crystals. Liq Cryst. 2018;45:2221–2252.
- Latinwo F, Stillinger FH, Debenedetti G. Molecular model for chirality phenomena. J Chem Phys. 2016;145:154503.
- Shanker G, Nagaraj M, Kocot A, et al. Nematic phases in 1,2,4-oxadiazole-based bent-core liquid crystals: is there a F erroelectric switching? Adv Funct Mater. 2012;22:1671–1683.
- Goodby JW, Collings PJ, Kato, et al. Handbook of Liquid Crystals. 2nd ed. Vol. 5 (Weinheim: Wiley-VCH) 2014 ; p. 34–36.
- Breckon R, Chakraborty S, Zhang C, et al. Nanostructures of nematic materials of laterally branched molecules. ChemPhysChem. 2014;15:1457–1462.
- Kim Y-K, Cukrov G, Vita F, et al. Search for microscopic and macroscopic biaxiality in the cybotactic nematic phase of new oxadiazole bent-core mesogens. Phys Rev E. 2016;93:062701.
- Kim Y-K, Majumdar M, Senyuk BI, et al. Search for biaxiality in a shape-persistent bent-core nematic liquid crystal. Soft Matter. 2012;8:8880–8890.
- Nagaraj M, Panarin YP, Manna U, et al. Electric field induced biaxiality and the electrooptic effecting a bent-core nematic liquid crystal. Appl Phys Lett. 2010;96:011106.
- Jang Y, Panov VP, Kocot A, et al. Optical confirmation of biaxial nematic (Nb) phase in a bent-core mesogen. Appl Phys Lett. 2009;95:183304.
- Chiappini M, Drwenski T, van Roij R, et al. Biaxial, twist-bend, and splay-bend nematic phases of banana-shaped particles, revealed by lifting the “smectic blanket”. Phys Rev Lett. 2019;123:068001.
- Ocak H, Bilgin-Eran B, Prehm M, et al. Effects of chain branching and chirality on liquid crystalline phases of bent-core molecules: blue phases, de Vries transitions and switching of diastereomeric states. Soft Matter. 2011;7:8266–8280.
- Green AAS, Tuchband MR, Shao R, et al. Chiral incommensurate helical phase in a smectic of achiral bent-core mesogens. Phys Rev Lett. 2019;122:107801.
- Lehmann A, Alaasar M, Poppe M, et al. Stereochemical rules govern the soft self-assembly of achiral compounds: understanding the heliconical liquid-crystalline phases of bent-core mesogens. Chem Eur J. 2020;26:4714–4733.
- Nagaraj M, Lehmann A, Prehm M, et al. Evidence of a polar cybotactic smectic A phase in a new fluorine substituted bent-core compound. J Mater Chem. 2011;21:17098–17103.
- Kim Y-K, Cukrov G, Xiang J, et al. Domain walls and anchoring transitions mimicking nematic biaxiality in the oxadiazole bent-core liquid crystal C7. Soft Matter. 2015;11:3963–3970.
- Arakawa Y, Ishida Y, Komatsu K, et al. Thioether-linked benzylideneaniline-based twist-Bend nematic liquid crystal dimers: insights into spacer lengths, mesogenic arm structures, and linkage types. Tetrahedron. 2021;95:132351.
- Cao Y, Feng C, Jakli A, et al. Deciphering chiral structures in soft materials via resonant soft and tender X-ray scattering. Giant. 2020;2:100018.
- Lewandowski W, Vaupotič N, Pociecha D, et al. Chirality of liquid crystals formed from achiral molecules revealed by resonant X-ray scattering. Adv Mater. 2020;1905591.
- Alaasar M, Prehm M, Nagaraj M, et al. A liquid crystalline phase with uniform tilt, local polar order and capability of symmetry breaking. Adv Mater. 2013;25:2186–2191.
- Alaasar M, Prehm M, May K, et al. 4-cyanoresorcinol-based bent-core mesogens with azobenzene wings: emergence of sterically stabilized polar order in liquid crystalline phases. Adv Funct Mater. 2014;24:1703–1717.
- Alaasar M, Prehm M, Poppe S, et al. Development of polar order by liquid-crystal self-assembly of weakly bent molecules. Chem Eur J. 2017;23:5541–5556.
- Alaasar M, Prehm M, Tamba M-G, et al. Development of polar order in the liquid crystalline phases of a 4-cyanoresorcinol-based bent-core mesogen with fluorinated azobenzene wings. ChemPhysChem. 2016;17:278–287.
- Alaasar M, Prehm M, Belau S, et al. Polar order, mirror symmetry breaking, and photoswitching of chirality and polarity in functional bent-core mesogens. Chem Eur J. 2019;25:6362–6377.
- Poppe M, Alaasar M, Lehmann A, et al. Controlling the formation of heliconical smectic phases by molecular design of achiral bent-core molecules. J Mater Chem C. 2020;8:3316–3336.
- Pociecha D, Cepic M, Gorecka E, et al. Ferroelectric mesophase with randomized interlayer structure. Phys Rev Lett. 2003;91:185501.
- Shimbo Y, Gorecka E, Pociecha D, et al. Electric-field-induced polar biaxial order in a nontilted smectic phase of an asymmetric bent-core liquid crystal. Phys Rev Lett. 2006;97:113901.
- Gupta M, Datta S, Radhika S, et al. Randomly polarised smectic A phase exhibited by bent-core molecules: experimental and theoretical studies. Soft Matter. 2011;7:4735–4741.
- Gomola K, Guo L, Pociecha D, et al. An optically uniaxial antiferroelectric smectic phase in asymmetrical bent-core compounds containing a 3-aminophenol central unit. J Mater Chem. 2010;20:7944–7952.
- Sebastian N, Belau S, Eremin A, et al. Emergence of polar order and tilt in terephthalate based bent-core liquid crystals. Phys Chem Chem Phys. 2017;19:5895–5905.
- Shanker G, Prehm M, Nagaraj M, et al. Development of polar order in a bent-core liquid crystal with a new sequence of two orthogonal smectic and an adjacent nematic phase. J Mater Chem. 2012;21:18711–18714.
- Alaasar M, Prehm M, Poppe M, et al. Development of polar order and tilt in lamellar liquid crystalline phases of a bent-core mesogen. Soft Matter. 2014;10:5003–5016.
- Chakraborty S, Das MK, Keith C, et al. Study of ferro- and anti-ferroelectric polar order in mesophases exhibited by bent-core mesogens. Mater Adv. 2020;1:5902.
- Link DR, Natale G, Shao R, et al. Spontaneous formation of macroscopic chiral domains in a fluid smectic phase of achiral molecules. Science. 1997;278:1924–1927.
- Keith C, Prehm M, Paranin YP, et al. Development of polar order in liquid crystalline phases of a banana compound with a unique sequence of three orthogonal phases. Chem Commun. 2010;46:3702–3704.
- Sreenilayam S, Panarin YP, Vij JK, et al. Biaxial order parameter in the homologous series of orthogonal bent-core smectic liquid crystals. Phys Rev E. 2013;88:012504.
- Sreenilayam S, Panarin YP, Vij JK, et al. Physical properties of SmAb phase in an achiral bent-core smectic liquid crystals. Ferroelectrics. 2012;431:196–201.
- Sreenilayam S, Panarin YP, Vij JK, et al. Occurrence of five different orthogonal smectic phases in a bent-core (BC) liquid crystal. Mol Cryst Liq Cryst. 2015;610:116–121.
- Nagaraj M, Sreenilayam S, Panarin YP, et al. Electric field induced transformations and dielectric properties in non-tilted phases of a bent-core smectic liquid crystal. Mol Cryst Liq Cryst. 2011;540:82–87.
- Sreenilayam S, Nagaraj M, Panarin YP, et al. Properties of non-tilted bent-core smectic liquid crystals. Mol Cryst Liq Cryst. 2012;553:140–146.
- Sreenilayam S, Nagaraj M, Panarin YP, et al. Structure and polymorphism of biaxial bent-core smectic liquid crystals. Mol Cryst Liq Cryst. 2012;553:133–139.
- Sreenilayam S, Panarin YP, Vij JK, et al. Biaxial order parameter in an achiral bent-core smectic liquid crystals. Ferroelectrics. 2012;431:190–195.
- Wirth I, Diele S, Eremin A, et al. New variants of polymorphism in banana-shaped mesogens with cyano-substituted central core. J Mater Chem. 2001;11:1642–1650.
- Panarin YP, Nagaraj M, Vij JK, et al. Field-induced transformations in the biaxial order of non-tilted phases in a bent-core smectic liquid crystal. Eur Phys Lett. 2010;92:26002.
- Sreenilayam SP, Panarin YP, Vij JK, et al. Fast linear electrooptic effect in non-chiral bent core nematic liquid crystals. Ferroelectrics. 2016;495:35–42.
- Nagaraj M, Panarin YP, Vij JK, et al. Liquid crystal display modes in a nontilted bent-core biaxial smectic liquid crystal. Appl Phys Lett. 2010;97:213505.
- Sreenilayam SP, Panarin YP, Vij KJ, et al. Development of ferroelectricity in the smectic phases of 4-cyanoresorcinol derived achiral bent-core liquid crystals with long terminal alkyl chains. Phys Rev Mater. 2017;1:035604.
- Panarin YP, Sreenilayam SP, Swaminathan V, et al. Observation of an anomalous SmA-SmC-SmA phase sequence in a bent- core liquid crystal derived from 4-cyanoresorcinol. Phys Rev Res. 2020;2:013118.
- Sreenilayam SP, Panarin YP, Vij JK, et al. Spontaneous helix formation in non-chiral bent-core liquid crystals with fast linear electro-optic effect. Nat Commun. 2016;7:11369.
- Vij JK, Panarin YP, Sreenilayam SP, et al. Investigation of the heliconical smectic SmCSPFhel phase in achiral bent-core mesogens derived from 4-cyanoresorcinol. Phys Rev Mater. 2019;3:045603.
- Panarin YP, Nagaraj M, Sreenilayam S, et al. Sequence of four orthogonal smectic phases in an achiral bent-core liquid crystal: evidence for the SmAPα phase. Phys Rev Lett. 2011;107:247801.
- Lagerwall ST. Ferroelectric and Antiferroelectric Liquid Crystals. Weinheim: Wiley-VCH; 1999.
- Fukuda A, Takanishi Y, Isozaki T, et al. Antiferroelectric chiral smectic liquid crystals. J Mater Chem. 1994;4:997–1016.
- Takezoe H, Gorecka E, Cepic M. Antiferroelectric liquid crystals: interplay of simplicity and complexity. Rev Mod Phys. 2010;82:897–937.
- Nakata M, Shao R-F, Maclennan JE, et al. Electric-field-induced chirality flipping in smectic liquid crystals: the role of anisotropic viscosity. Phys Rev Lett 2006 . 96():067802
- Panarin YP, Sreenilayam SP, Vij JK, et al. A fast linear electro-optical effect in a non-chiral bent-core liquid crystal. J Mater Chem C. 2017;5:12585–12590.
- Meyer C, Davidson P, Constantin D. Freedericksz-like transition in a biaxial smectic-A phase. Phys Rev X. 2021;11:031012.
- Mach P, Pindak R, Levelut A-M, et al. Structures of chiral smectic-C mesophases revealed by polarization-analyzed resonant x-ray scattering. Phys Rev E. 1999;60:6793–6802.
- Lagerwall JPF. Demonstration of the antiferroelectric aspect of the helical superstructures in Sm-C*, Sm-Cα*, and Sm-Ca* liquid crystals. Phys Rev E. 2005;71:051703.
- Panov VP, Shtykov NM, Fukuda A, et al. Self-assembled uniaxial and biaxial multilayer structures in chiral smectic liquid crystals frustrated between ferro- and antiferroelectricity. Phys Rev E. 2004;69:060701(R).
- Lazar C, Yang K, Glaser MA, et al. Ferroelectric liquid crystal induced by a bridged biphenyl dopant with helical topography. J Mater Chem. 2002;12:586–592.
- Greco C, Ferrarini A. Entropy-driven chiral order in a system of achiral bent particles. Phys Rev Lett. 2015;115:147801.
- Matsuyama A. Phase transitions of heliconical smectic-C and heliconical nematic phases in banana-shaped liquid crystals. Phys Rev E. 2020;101:050701(R).
- Abberley JP, Killah R, Walker R, et al. Heliconical smectic phases formed by achiral molecules. Nat Commun. 2018;9:228.
- Salamończyk M, Vaupotič N, Pociecha D, et al. Multi-level chirality in liquid crystals formed by achiral molecules. Nat Commun. 2019;10:1922.
- Mandle RJ, Goodby JW. A twist-Bend nematic to an intercalated, anticlinic, biaxial phase transition in liquid crystal bimesogens. Soft Matter. 2016;12:1436–1443.
- Walker R, Pociecha D, Storey JMD, et al. Remarkable smectic phase behaviour in odd-membered liquid crystal dimers: the CT6O.m series. J Mater Chem C. 2021;9:5167–5173.
- Beguin L, Emsley JW, Lelli M, et al. The chirality of a twist−Bend nematic phase identified by NMR spectroscopy. J Phys Chem B. 2012;116:7940–7951.
- Ivsic T, Vinkovic M, Baumeister U, et al. Milestone in the NTB phase investigation and beyond: direct insight into molecular self-assembly. Soft Matter. 2014;10:9334–9342.
- Stevenson WD, Zeng XB, Welsch C, et al. Macroscopic chirality of twist-Bend nematic phase in bent dimers confirmed by circular dichroism. J Mater Chem C. 2020;8:1041–1047.
- Salamonczyk M, Mandle RJ, Makal A, et al. Double helical structure of the twist-Bend nematic phase investigated by resonant X-ray scattering at the carbon and sulfur K-edges. Soft Matter. 2018;14:9760–9763.
- Stevenson WD, Ahmed Z, Zeng XB, et al. Molecular organization in the twist–Bend nematic phase by resonant X-ray scattering at the Se K-edge and by SAXS, WAXS and GIXRD. Phys Chem Chem Phys. 2017;19:13449–13454.
- Emsley JW, Lelli M, Lesage A, et al. A comparison of the conformational distributions of the achiral symmetric liquid crystal dimer CB7CB in the achiral nematic and chiral twist-bend nematic phases. J Phys Chem B. 2013;117:6547–6557.
- Shi Y, Sun Z, Chen R, et al. Effect of conformational chirality on optical activity observed in a smectic of achiral, bent-core molecules. J Phys Chem B. 2017;121:6944–6950.
- Matsuyama A. Twist-bend nematic phases of bananashaped molecules with an axial chirality. Liq Cryst. 2019;46:2301–2321.
- Toxvaerd S. Molecular dynamics simulations of isomerization kinetics in condensed fluids. Phys Rev Lett. 2000;22:4747–4750.
- Dressel C, Reppe T, Prehm M, et al. Chiral self-sorting and amplification in isotropic liquids of achiral molecules. Nat Chem. 2014;6:971–977.
- Zeng X, Ungar G. Spontaneously chiral cubic liquid crystal: three interpenetrating networks with twist. J Mater Chem. 2020;8:5389–5398.
- Lu H, Zeng XB, Ungar G, et al. The solution of the puzzle of smectic-Q: the phase structure and the origin of spontaneous chirality. Angew Chem Int Ed. 2018;57:2835–2840.
- Dressel C, Liu F, Prehm M, et al. Dynamic mirror-symmetry breaking in bicontinuous cubic phases. Angew Chem Int Ed. 2014;53:13115–13120.
- Reppe T, Poppe S, Cai X, et al. Spontaneous mirror symmetry breaking in benzil-based soft crystalline, cubic liquid crystalline and isotropic liquid phases. Chem Sci. 2020;11:5902–5908.
- Zeldovich YB. Pseudoscalar liquid crystals. Zh Eksp Teor Fiz. 1971;67:2357–2361.
- Kats EI. Spontaneous chiral symmetry breaking in liquid crystals. Low Temp Phys. 2017;43:5–7.
- Takezoe H. Spontaneous achiral symmetry breaking in liquid crystalline phases. Top Curr Chem. 2012;318:303–330.
- Jacques J, Collet A, Wilen SH. Enantiomers, racemates, and resolutions. New York: J. Wiley & Sons, Inc; 1981.
- Dutta S, Gellman AJ. Enantiomer surface chemistry: conglomerate versus racemate formation on surfaces. Chem Soc Rev. 2017;46:7787–7839.
- Fuhrhop J-H, Helfrich W. Fluid and solid fibers made of lipid molecular bilayers. Chem Rev. 1993;93:1585.
- Novotna V, Glogarova M, Kaspar M, et al. Reentrant orthogonal smectic-A phase below a tilted smectic-C phase in a chiral compound. Phys Rev E. 2011;83:020701(R.
- Weissflog W, Lischka C, Diele S, et al. The inverse phase sequence SmA–SmC in symmetric dimeric liquid crystals. Liq Cryst. 2000;27:43–50.
- Mertelj A, Cmok L, Sebastián N, et al. Splay nematic phase. Phys Rev X. 2018;8:041025.
- Chen X, Körblova E, Dong D, et al. First-principles experimental demonstration of ferroelectricity in a thermotropic nematic liquid crystal: polar domains and striking electro-optics. Proc Natl Acad Sci USA. 2020;117:14021–14031.
- Chiappini M, Dijkstra M. A generalized density-modulated twist-splay-Bend phase of banana-shaped particles. Nat Commun. 2021;12:2157.
- Revignas D, Ferrarini A. From bend to splay dominated elasticity in nematics. Crystals. 2021;11:831.
- Fernández-Rico C, Chiappini M, Yanagishima T, et al. Shaping colloidal bananas to reveal biaxial, splay-Bend nematic, and smectic phases. Science. 2020;369:950–955.
- Pelzl G, Eremin A, Diele S, et al. Spontaneous chiral ordering in the nematic phase of an achiral bananashaped compound. J Mater Chem. 2002;12:2591–2593.
- Görtz V, Southern C, Roberts NW, et al. Unusual properties of a bent-core liquid-crystalline fluid. Soft Matter. 2009;5:463–471.
- Salter PS, Tschierske C, Elston SJ, et al. Flexoelectric measurements of a bent-core nematic liquid crystal. Phys Rev E. 2011;84:031708.
- Jang Y, Balachandran R, Keith C, et al. Chirality of an achiral bent-core nematic mesogens observed in planar and homeotropic cells under certain conditions. Soft Matter. 2012;8:10479–10485.
- Dietrich CF. Lyotropic nematic liquid crystals: interplay between small twist elastic constant and chirality effects under confined geometries. Liq Cryst Today. 2021;30:2–14.
- Longa L, Pająk G, Wydro T. Chiral symmetry breaking in bent-core liquid crystals. Phys Rev E. 2009;7:040701(R).
- Pleiner H, Brand HR. Tetrahedral order in liquid crystals. Braz J Phys. 2016;46:565–595.
- Hough LE, Spannuth M, Nakata M, et al. Chiral isotropic liquids from achiral molecules. Science. 2009;325:452–456.
- Le KV, Takezoe H, Araoka F. Chiral superstructure mesophases of achiral bent-shaped molecules –hierarchical chirality amplification and physical properties. Adv Mater. 2017;1602737.
- Westphal E, Gallardo H, Sebastian N, et al. Liquid crystalline self-assembly of 2,5-diphenyl-1,3,4-oxadiazole based bent-core molecules and the influence of carbosilane end-groups. J Mater Chem. 2019;7:3064–3081.
- Yoshizawa A. Molecular design of flexible liquid crystal oligomers stabilising the chiral frustrated phases. Liq Cryst. 2017;44:1877–1893.
- Westphal E, Gallardo H, Caramori GF, et al. Polar order and symmetry breaking at the bounary between bent-core and rodlike molecular forms. When 4-cyanoresorcinol meets carbosilane end groups. Chem Eur J. 2016;22:8181–8197.
- Alaasar M, Prehm M, Tschierske C. Influence of halogen substituent on the mesomorphic properties of five-ring banana-shaped molecules with azobenzene wings. Liq Cryst. 2013;40:656–668.
- Alaasar M, Prehm M, Tschierske C. A new room temperature dark conglomerate mesophase formed by bent-core molecules combining 4-iodoresorcinol with azobenzene units. Chem Commun. 2013;49:11062–11064.
- Alaasar M, Prehm M, Brautzsch M, et al. 4-methylresorcinol-based bent-core liquid crystals with azobenzene wings – a new class of compounds with dark conglomerate phases. J Mater Chem C. 2014;2:5487–5501.
- Alaasar M, Prehm M, Brautzsch M, et al. Dark conglomerate phases of azobenzene derived bent-core mesogens – relationships between the molecular structure and mirror symmetry breaking in soft matter. Soft Matter. 2014;10:7285–7296.
- Alaasar M, Prehm M, Tschierske C. Helical nano-crystallite (HNC) phases: chirality synchronization of achiral bent-core mesogens in a new type of dark conglomerates. Chem Eur J. 2016;22:6583–6597.
- Alaasar M, Prehm M, Tschierske C. Mirror symmetry breaking in fluorinated bent-core mesogens. RSC Adv. 2016;6:82890–82899.
- Keith C, Reddy RA, Prehm M, et al. Layer frustration, polar order and chirality in liquid crystalline phases silyl-terminated achiral bent-core molecules. Chem Eur J. 2007;13:2556–2577.
- Lansac Y, Maiti PK, Clark NA, et al. Phase behavior of bent-core molecules. Phys Rev E. 2003;67:011703.
- Chattham N, Tamba M-G, Stannarius R, et al. Leaning-type polar smectic-C phase in a freely suspended bent-core liquid crystal film. Phys Rev E. 2015;91:030502(R.
- Chattham N, Korblova E, Shao R, et al. de Gennes’ triclinic smectics – not so far-fetched after all. Liq Cryst. 2009;36:1309–1317.
- Kovářová A, Světlík S, Kozmík V, et al. Unusual polymorphism in new bent-shaped liquid crystals based on biphenyl as a central molecular core. Beilstein J Org Chem. 2014;10:794–807.
- Chen W-H, Chuang W-T, Jeng U-S, et al. New SmCG phases in a hydrogen-bonded bent-core liquid crystal featuring a branched siloxane terminal group. J Am Chem Soc. 2011;133:15674–15685.
- Zhang C, Diorio N, Radhika S, et al. Two distinct modulated layer structures of an asymmetric bent-shape smectic liquid crystal. Liq Cryst. 2012;39:1149–1153.
- Ocak H, Poppe M, Bilgin-Eran B, et al. Effects of molecular chirality on self-assembly and switching in liquid crystals at the cross-over between rod-like and bent shapes. Soft Matter. 2016;12:7405–7422.
- Walba DM, Körblova E, Shao R, et al. A ferroelectric liquid crystal conglomerate composed of racemic molecules. Science. 2000;288:2181–2184.
- Kumazawa K, Nakata M, Araoka F, et al. Important role played by interlayer steric interactions for the emergence of the ferroelectric phase in bent-core mesogens. J Mater Chem. 2004;14:157–164.
- Zhang Y, O’Callaghan MJ, Baumeister U, et al. Bent-core mesogens with branched carbosilane termini: flipping suprastructural chirality without reversing polarity. Angew Chem Int Ed. 2008;47:6892–6896.
- Ocak H, Bilgin Eran B, Nuray S, et al. Extraordinary magnetic field effects on the LC phases of homochiral and racemic 4-cyanoresorcinol-based diamagnetic bent-core mesogens. J Mater Chem C. 2021;9:1895–1910.
- Domenici V, Marini A, Veracini CA, et al. Effect of the magnetic field on the supramolecular structure of chiral smectic C phases: 2HNMR studies. ChemPhysChem. 2007;8:2575–2587.
- Domenici V, Veracini CA, Novotna V, et al. Twist grain boundary liquid-crystalline phases under the effect of the magnetic field: a complete 2H and 13C NMR study. ChemPhysChem. 2008;9:556–566.
- Ocak H, Canli NY, Bilgin Eran B. synthesis, mesomorphic and dielectric properties of new bent-core liquid crystal with a terminal lactate group. J Mol Struct. 2021;1223:128975.
- Le KV, Hafuri M, Ocak H, et al. Unusual electro-optic Kerr response in a self-stabilized amorphous blue phase with nanoscale smectic clusters. ChemPhysChem. 2016;17:1425–1429.
- Gim M-J, Hur S-T, Park KW, et al. Photoisomerization-induced stable liquid crystalline cubic blue phase. Chem Commun. 2012;48:9968–9970.
- Gim M-J, Han G, Choi SW, et al. Thermal phase transition behaviours of the blue phase of bent-core nematogen and chiral dopant mixtures under different boundary conditions. Soft Matter. 2014;10:8224–8228.
- Liu H, Shen D, Wang X, et al. Wide blue phase range induced by bent-shaped molecules with acrylate end groups. Opt Mater Exp. 2016;6:436.
- Ocak H, Bilgin-Eran B, Güzeller D, et al. Twist grain boundary (TGB) states of chiral liquid crystalline bent-core mesogens. Chem Commun. 2015;51:7512–7515.
- Reddy RA, Sadashiva BK, Baumeister U. Liquid crystalline properties of unsymmetrical bent-core compounds containing chiral moieties. J Mater Chem. 2005;15:3303–3316.
- Taushanoff S, Van Le K, Williams J, et al. Stable amorphous blue phase of bent-core nematic liquid crystals doped with a chiral material. J Mater Chem. 2010;20:5893–5898.
- Alaasar M, Poppe S, Kerzig C, et al. Cluster phases of 4-cyanoresorcinol derived hockey-stick liquid crystals. J Mater Chem C. 2017;5:8454–8468.
- Kosata B, Tamba G-M, Baumeister U, et al. Liquid-crystalline dimers composed of bent-core mesogenic units. Chem Mater. 2006;18:691–701.
- Wang Y, Yoon HG, Bisoyi HK, et al. Hybrid rod-like and bent-core liquid crystal dimers exhibiting biaxial smectic a and nematic phases. J Mater Chem. 2012;22:20363–20367.
- Shanker G, Prehm M, Tschierske C. Laterally connected bent-core dimers and bent-core-rod couples with nematic liquid crystalline phases. J Mater Chem. 2012;22:168–174.
- Yelamaggad CV, Prasad SK, Nair GG, et al. Low-molar-mass, monodispersive, bent-rod dimer exhibiting biaxial nematic and smectic a phases. Angew Chem Int Ed. 2004;43:3429–3432.
- Wang Y, Zheng Z-G, Bisoyi HK, et al. Thermally reversible full color selective reflection in a self-organized helical superstructure enabled by a bent-core oligomesogen exhibiting a twist-Bend nematic phase. Mater Horiz. 2016;3:442–446.