Abstract
In this work, we explored the possibility to detect sub-3 nm particles with commercially available TSI 3772 and Airmodus A20 Condensation Particle Counters (CPCs), when operated under modified temperature and inlet flow settings. We generated highly monodisperse sub-3 nm nanoparticles and characterized the CPCs with temperature differences between the saturator and the condenser varying from 36ºC (the 36/37 settings) to 40ºC (the 40/40 settings), while the factory settings were 17 and 24ºC. The 36/37 settings yielded no homogeneously nucleated background in dry conditions. With these settings, the detection efficiency was significantly improved from the factory settings, resulting in the detection of the smallest charged particles down to below 1.5 nm compared with the nominal cut-sizes of 10 and 7 nm. With the 40/40 settings and consequently higher supersaturation, homogeneous nucleation produced a background of around 0.5–2 cm−3, while the CPCs were sensitive to charged particles down to 1 nm in mobility diameter. The supersaturation field corresponding to the new operation conditions with the 36/37 settings was modeled by using COMSOL and OpenFOAM. The observations were reproduced very well by applying the heterogeneous nucleation theory to the obtained supersaturation field. Our work shows that the TSI 3772 and Airmodus A20 fine CPCs can have a comparable performance with a more expensive ultrafine CPC, such as TSI 3776, thus offering a widely available tool for the detection of sub-3 nm particles.
Copyright 2015 American Association for Aerosol Research
INTRODUCTION
To understand aerosol processes related to nanoparticle fabrication, combustion, and atmospheric particle formation, detection of the smallest, sub-3 nanometer (nm) particles, is required (McMurry et al. 2011). Measurement of particle size distributions is usually done by bringing a polydisperse aerosol sample into a known charge equilibrium with a radioactive charger, from which a monodisperse-size band of particles is selected with a differential mobility analyzer (DMA), and the concentration is measured with a condensation particle counter (CPC). If the charging probability, CPC detection efficiency, losses in DMA, and sampling lines are known, the amount of neutral particles can be determined via inversion. The whole system is called scanning mobility particle sizer (SMPS) or differential mobility particle sizer (DMPS) (McMurry Citation2000). Among inlet line losses and DMA sizing resolution, a difficulty in utilizing the DMPS system for sub-3 nm size distribution measurement is weak detection efficiency of a CPC for sub-3 nm particles. The cut-off diameter (diameter where the instrument detects 50% of the sampled particles) is dependent on the diffusion losses inside the instrument as well as on the supersaturation of the working fluid that is driven by the temperature difference between the saturation and the condenser (ΔT). The diffusion losses can be minimized by changes in the instrument design, while the supersaturation can be optimized by the selection of suitable working liquid (Iida et al. Citation2009) and operation temperatures (Mertes et al. Citation1995; Kuang et al. Citation2012b). The SMPS systems are rarely used for particles smaller than 2 nm (Kuang et al. Citation2012a) due to low activation efficiency of most CPCs for such small particles, and high sampling line losses for the smallest particles.
Before 1991, not many laminar-type CPCs were able to detect sub-4 nm particles (Agarwal and Sem Citation1980; Ahn and Liu Citation1990; Su et al. Citation1990; Bartz et al. Citation1985; Scheibel and Porstendorfer Citation1986). A major milestone was reached when Stolzenburg and McMurry (Citation1991) developed the first ultrafine CPC capable of detecting 3-nm particles. Among other developments, the design was based on a sheath flow inside the condenser of the CPC – introduced by Wilson et al. (Citation1983) – to lead the particles only to the middle of the condenser where the supersaturation was maximum while keeping the diffusional losses at their minimum. Later on, the fine CPC TSI 3010 was shown to be able to detect sub-5 nm particles, even down to 2.5 nm by modification of operation temperatures (Mertes et al. Citation1995; Russell et al. Citation1996; Wiedensohler et al. Citation1997). Another option for sizing of particles is realized by introducing a pulse height analysis to the ultrafine CPC optics. With the pulse height analysis it was possible to infer the initial seed size from pulse height scattered by the grown droplet (Weber et al. Citation1998).
The limiting factor in the cut-size improvement is set by homogeneous nucleation of working fluid. If ΔT inside the laminar flow ultrafine, or in any other CPC, is increased enough, homogeneous nucleation of the working liquid will occur. Sipilä et al. (Citation2009) utilized the pulse height analysis to separate homogeneously and heterogeneously grown droplets to measure sub-6 nm particle size distributions. The ultrafine CPC is also modified to operate with diethylene glycol as a working liquid, which has been shown to be a suitable detector in an SMPS system to detect atmospheric nucleation (Jiang et al. Citation2011), and has been also pushed down to sub-2 nm particle detection (Kuang et al. Citation2012b; Wimmer et al., Citation2013). Another laminar-type ultrafine CPC is based on water as a condensing liquid (Hering et al. Citation2005), from which a sheathed condenser ultrafine version was characterized by Iida et al. (Citation2008). The ultrafine water CPC can be boosted to sub-2 nm particle detection (Kangasluoma et al. Citation2014).
In mixing-type CPCs, the supersaturation of a working liquid is created by mixing a saturated flow turbulently with the sample flow. The first mixing CPCs able to reach sub-3 nm particle detection was developed by Seto et al. (Citation1997), and further by Gamero-Castano and de la Mora (Citation2000), Kim et al. (Citation2002), and Sgro and de la Mora (Citation2004). Due to the complexity of the mixing-type CPC, it has remained mostly a laboratory instrument, until a commercial version was made available by Airmodus (Vanhanen et al. Citation2011), which has been proven to be effective in analyzing initial steps of new particle formation (Kulmala et al. Citation2013). The mixing-type CPC offers advantages over laminar-type CPC for sub-3 nm particle detection due to smaller diffusion losses made possible by the design, and a possibility for size distribution measurement by varying the mixing ratio of the saturator and the sample flow, thus varying the cut-off diameter (Lehtipalo et al. 2014). The last of the three utilized methods for creating high supersaturation is adiabatic expansion. Kurten et al. (Citation2007) developed an automated expansion-type CPC and found better particle activation at 3 nm than TSI ultrafine 3025A. The expansion CPCs were the first ones used for environmental monitoring, but due to discontinuous sampling, these are not suitable detectors for DMPS systems. Expansion CPCs, however, offer high precision supersaturation control, and thus accurate laboratory studies on nucleation (Winkler et al. Citation2008, Citation2012).
In our article, we offer two new simple tools for sub-3 nm particle detection, which are the laminar-type TSI 3772 CPC (comparable with the TSI 3010 CPC) and laminar-type Airmodus A20 CPCs. We optimized the detection of sub-3 nm particles by increasing the temperature difference between the saturator and the condenser as high as possible without homogeneous nucleation, and explored the performance of the instruments in the situation when a small amount of homogeneous nucleation is acceptable.
EXPERIMENTAL
To characterize the CPCs, we generated negative tungsten oxide test particles with a hot wire generator (Peineke et al. Citation2006; Kangaluoma et al. 2015). A spring of tungsten wire is resistively heated up in a chamber flushed with N2, and vapor emitted from the surface forms particles after cooling down in the surrounding gas. A fraction of the particles are self-charged (Peineke and Schmidt-Ott Citation2008), omitting the need for particle charging. The self-charged negative tungsten oxide clusters were size-selected with a high-resolution Herrmann-type DMA (Herrmann et al. Citation2000), with mobility resolution of approximately 15 (peak voltage/full width at half maximum). The experimental setup is presented in . In this setup, the particles emitted from the hot wire are mostly tungsten oxide, with possibly a small fraction of some organic impurities (Kangasluoma et al. Citation2013). The detection efficiency was measured at 14 different mobility diameters for 1 min at each diameter, from which median detection efficiency was calculated as the ratio of the concentrations detected by a CPC and a reference electrometer (TSI 3068B). The minimum concentration detected by the electrometer was approximately 1000 cm−3 and maximum was 10,000 cm−3. All presented figures are raw detection efficiencies without any correction or scaling, except possible subtraction of the CPC homogeneous background concentration.
TSI 3772 and Airmodus A20 are laminar-type CPCs in which the whole aerosol sample flow is heated up and saturated with butanol vapor. Afterwards the saturated flow is brought to a multitube condenser (8 in 3772 and 6 in A20) where the flow is cooled down and particle growth occurs before optical detection. Nominal cut-off diameters of these instruments are 10 and 7 nm, inlet flow is 1 L per min (Lpm), and the factory temperature settings for saturator are 39 and 39ºC, condenser 22 and 15ºC, and optics 40 and 40ºC for 3772 and A20, respectively.
In contrast to TSI 3772 and Airmodus A20 CPCs, the corresponding ultrafine CPCs (TSI 3776 and Airmodus A11) are optimized for sub-3 nm particle detection. The ultrafine TSI 3776 is different compared with the fine CPCs in that the sample flow is divided into sheath flow, which is filtered and saturated, and that the sample flow is fed into the middle of the condenser with a small capillary. The sheath flow is saturated with butanol and brought to the condenser around the sample flow. This way the sample is at the point of the highest supersaturation in the condenser with minimized diffusion losses, as it is fed to the centerline. Another difference to the fine CPCs is that the optical detector is different in the sense that the detected flow rate through the detector is only 0.3 Lpm, of which 0.03 Lpm is aerosol flow, compared with the 1 Lpm of the fine CPCs. This is due to better optical detectors of the A20 and 3772 CPCs. Indeed, the optical detector of A20 and 3772 are based on the design of 3760, which has been introduced by TSI for clean rooms, where the particle concentrations larger than 10 nm are very low. The Airmodus A11 nano condensation nuclei counter (nCNC) comprises an A10 particle size magnifier (PSM) (Vanhanen et al. Citation2011) and an A20 CPC. The PSM is a mixing-type particle magnifier where diethylene glycol-saturated flow is mixed with the sample flow, and the mixed flow is cooled down in the condenser to grow the particles up to 90 nm. After the growth, the particles are counted with A20. The design of the PSM minimizes the inlet line wall losses and allows scanning of the saturator flow to measure size distributions of 1–2.5-nm particles, which are not studied here.
To enhance the small particle detection from the factory settings of the TSI 3772 and the Airmodus A20 CPCs, we increased the temperature difference of the saturator and condenser close to the maximum allowed by the CPC firmware. These conditions, referred to as “40/40,” employed saturator temperatures of 50ºC and condenser temperatures of 10ºC for both the TSI 3772 and Airmodus A20 CPCs, and operated the optics at 50ºC for the 3772 CPC and 51ºC for the A20 CPC. With these settings, the homogeneous nucleation of butanol started to occur, and the determined background concentration was approximately 0.2–2 cm−3 during our experiments in dry conditions for both of the instruments. This background was subtracted from the CPC signal when calculating detection efficiencies.
For experiments with maximum supersaturation without the homogeneous background (defined as 1 count min−1), the employed temperature settings, referred to as 36/37, were as follows: saturator temperatures of 46 and 47ºC, optics temperature of 47 and 48ºC for TSI 3772 and Airmodus A20, respectively, and the condenser temperature of 10ºC. The amount of homogeneous nucleation inside the instruments is sensitive to the temperature sensor calibration and can vary from instrument to instrument; therefore, we utilized the amount of homogeneous nucleation as a trace for supersaturation instead of set point temperatures.
To probe the possibility of increasing the detection efficiency by changing the inlet flow, we conducted an experiment with the A20 CPC, where the detection efficiency of 1.2-nm negative tungsten oxide was measured as a function of the inlet flow. The inlet flow was varied between 1 Lpm and 3 Lpm by removing critical orifice in A20, which controls the inlet flow, and a needle valve together with a mass flow meter was placed instead. From this set of experiments, we selected the flow corresponding to the maximum detection efficiency, and subsequently the cut-off diameter was measured with this flow rate. A compilation of the instruments and their operation conditions is given in .
TABLE 1 Instruments compared in this study with their operation conditions
To assess the sensitivity of CPCs to small ion clusters in large quantities, we pushed 2 Lpm of dry, particle-free air through a radioactive 14C source, and sampled the ions with fine CPCs operated at both 36/37 and 40/40 settings.
MODELING
In our model, we calculated the temperature, flow, and consequently the supersaturation field in one of the tubes of the multitube condenser of 3772 and A20, assuming that the condenser radius is 2.5 mm and length is 85 mm. The ingoing air was assumed to be uniformly saturated by the saturator with a uniform temperature profile. The flow inside the condenser tube was 1/6 (A20) or 1/8 Lpm (3772). The flow, temperature, and supersaturation profiles were obtained by using COMSOL Multiphysics simulation software. Laminar flow model with incompressible Navier–Stokes equations, including heat conduction, convection, and diffusion were used from COMSOL Multiphysics CFD module to simulate fluid flow, and heat and vapor transport in the conditioner. The supersaturation profile in the condenser was calculated assuming the inner walls are at preset temperature with a saturation ratio of unity close to the wall. Thermodynamical properties of n-butanol were taken from Magnusson et al. (Citation2003) and the supersaturation field was further used for calculating heterogeneous nucleation probability (Winkler et al. Citation2008; Stratmann et al. Citation2010). To model heterogenous nucleation, we discretized the modeled temperature and supersaturation field into axial (850) and radial (75 for supersaturation field modeling, which was further interpolated into 300 for heterogeneous nucleation model) bins, and with the flow field we were able to determine the time a particle spends in each bin. With this information, we are able to determine spatially and temporally resolved onset of heterogeneous nucleation, and therefore model the CPC detection efficiency that we can compare with experimental values. Here we assumed that all of the activated particles grow to detectable sizes. The modeled curve for the activation efficiency was calculated as a ratio of flux-averaged activated particle concentration and flux-averaged total particle concentration in the same manner as in Giechaskiel et al. (Citation2011). Flux-averaged concentration takes into account the parabolic flow velocity profile in the tube.
From the modeled supersaturation field, the activation efficiency was calculated by using heterogeneous nucleation theory, which was corrected for estimated inlet line penetration to obtain detection efficiency. The inlet line losses in the CPC were estimated with activation efficiency parametrization from Stolzenburg and McMurry (Citation1991) and diffusion loss parametrization by Gormley and Kennedy (Citation1949), for which the detection efficiency was fitted. With a measured plateau value of 0.87, the effective line length for inlet losses was fitted to be 1.13 m. The modeled inlet line length of 1.13 m is not a realistic value for the line beginning from the inlet of the CPC to the optics. We think that to make the experiment and simulation to match, this high value is needed since the final droplet size becomes bigger due to higher supersaturation. Presumably, these bigger particles will have larger losses (compared with the final droplet size obtained by the factory settings) in the nozzle due to higher inertia, which is not included in the simulation, as there we assumed that all particles that activate in the condenser will be counted by the optics. Therefore, the diffusion loss term includes all particle losses in the CPC.
The modeling of the supersaturation field was verified independently by repeating the simulations in OpenFOAM 2.3, which is a free, open source C++ software package for continuum mechanics problems, including computational fluid dynamics (Weller et al. Citation1998; OpenFOAM Citation2015). The standard icoFoam solver for the laminar flow of Newtonian fluids was modified to solve temperature and scalar transport, and thereby the supersaturation field. The transient solver was run for long enough to ensure convergence in the simple axial-symmetric tube decomposed into a hexahedral block mesh.
RESULTS
presents the detection efficiency curves for TSI 3772 and Airmodus A20 operated at inlet flows of 1 Lpm and two different temperature settings 36/37 and 40/40, with 0.2–2 cm−3 homogeneous nucleation background at the 40/40 settings. As a comparison, the detection efficiency curve for Airmodus A11 and TSI 3776 are given in . The cut-off for the 3776 CPC was measured at three different temperature settings: ΔT of 29 (factory settings), 34 (similar homogeneous background as in 36/37 settings of the fine CPCs), and 36ºC (similar homogeneous background as in the 40/40 settings). Our results show that the TSI 3772 and Airmodus A20 CPCs exhibit similar detection efficiency as the ultrafine 3776 CPC, with somewhat lower detection efficiency and a shallower detection efficiency curve. This is due to the higher inlet losses in the fine CPCs and probably because in the ultrafine CPC the aerosol is only in the middle of the condenser where the supersaturation is highest. When the fine CPCs are operated at the 40/40 settings, the detection for the smallest clusters increased approximately by a factor of 2 for sub-2 nm particles, with a non-zero detection also for the smallest generated 1.1-nm clusters compared with the 36/37 settings. The plateau value of the detection efficiency for the boosted A20 was measured to be at 0.87 at 20–30 nm with the 36/37 settings, and at 0.84 with the 40/40 settings. Probably, the cut-off plateau value is lower due to a bigger final droplet diameter, which caused additional losses of the activated particles in the nozzle leading to the optics of the instrument.
FIG. 2. Detection efficiencies for the tuned 3772 and A20 CPCs with inlet flows of 1 Lpm, and as a comparison A11 and tuned 3776. By tuning 3772 and A20, it is possible to reach detection efficiencies comparable with the ultrafine counters.
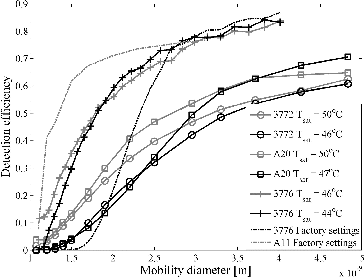
When the fine CPCs were operated at the 40/40 settings, they detected 500–1000 cm−3 ions from the charger, which shows that they are able to detect a small fraction of air ions too. When operated at the 36/37 settings, the fine CPCs did not detect any air ions from the radioactive charger. The concentration of ions formed in the radioactive chargers is estimated to be 0.8 e8 cm−3 in steady state (Reischl et al. Citation1996), therefore the detected fraction at the 40/40 settings is almost negligible.
By varying the A20 inlet flow we found that the maximum detection efficiency for 1.2-nm negative tungsten oxide particles at the 40/40 settings was 1.6 Lpm (). When the flow is increased from 1 Lpm, the diffusion losses inside the CPC become smaller while the supersaturation maximum still stays inside the condenser. At flow rates higher than 1.6 Lpm, even if the diffusion losses become still smaller, the supersaturation maximum in the condenser becomes lower, similarly as shown by Kuang et al. (Citation2012b). We measured the cut-off for A20 with 1.6 Lpm inlet flow at the same two temperature settings as used earlier. The result is presented in and the corresponding background concentrations are given in . Our data suggest that the sub-2 nm particle detection can be boosted further down by increasing the inlet flow. Probably, if the firmware of the CPCs would allow even higher ΔT, the detection efficiency could be increased more by increasing the inlet flow, which would reduce the sampling losses.
FIG. 3. A20 detection efficiency as a function of inlet flow for 1.2-nm negative tungsten oxide particles. The detection efficiency peaks at 1.6 Lpm.
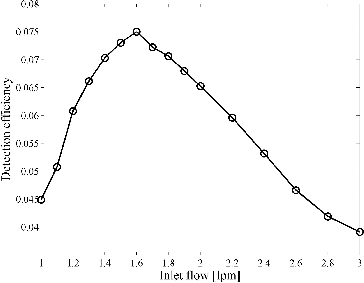
Modeling of the A20 condenser with ingoing sample air temperature at 45°C and condenser wall temperature at 10°C yielded a supersaturation profile presented in . The peak supersaturation was approximately 4.2, and the fields from COMSOL and OpenFOAM simulations were equal to within second decimal. Instead of using the saturator temperature settings, which were used in the experiments, the temperature used for the ingoing air in the model was tuned by comparing the model with the measured detection efficiency curve, and taking the best fitting temperature. As the CPC measurement principle relies on temperature difference between the saturator and the condenser, and the temperature sensors do not always reflect the real temperature inside the saturator or condenser, the supersaturation can vary from instrument to instrument with equal temperature set points. Therefore, there can also be differences between two CPCs of the same manufacturer and model in the absolute temperature readings. As a practical manifestation of this effect, the cut-off calibration for A20 at the brink of homogeneous nucleation was done with two different CPCs. While the one was operated at saturator temperature of 47ºC and the other at 43ºC, the performance evaluation revealed identical detection efficiency, confirming also the hypothesis of using the homogeneous nucleation as a practical trace for supersaturation. The modeled detection efficiency is presented in together with measured detection efficiency at the same settings. The big difference between activated and detected particles is, as said, probably due to the bigger final droplet size, for which the current design of the condenser, nozzle, and optics is not optimized. The agreement in detection efficiency is reasonably good between the model and the experiments, verifying the model performance and encouraging further development of CPCs.
CONCLUSIONS
Based on our laboratory experiments, we conclude that operation of the 3772 and A20 CPCs at high temperature difference between the saturator and the condenser yields to similar results compared with the ultrafine CPC design, thus offering access to sub-3 nm particle detection with conventional and more affordable fine CPCs 3772 and A20. The experimental cut-offs were well reproduced by model studies in COMSOL and OpenFOAM, when the heterogeneous nucleation theory was implemented. By setting the temperature differences of the fine CPCs to 36 and 37ºC, (brink of homogeneous nucleation), the measured detection efficiency was high enough to make them suitable to operate them as a detector in a DMPS system, which measures particles down to 3 nm. Further optimization of the inlet flow or temperatures will allow even 1-nm charged particle detection if small homogeneous background nucleation is accepted. CPCs with homogeneous nucleation can be operated, for example, as a part of a CPC battery (Kulmala et al. Citation2007), in laboratory tests or in an operation mode in which the particle size classification is done via supersaturation scanning by flow or temperature scan. Overall, as already mentioned by Stolzenburg and McMurry (Citation1991), the main obstacle for 1-nm particle detection is diffusion losses in the instrument inlet line.
Funding
This work was partly funded by European Research Council (ATMNUCLE, 227463), Academy of Finland (Center of Excellence Program projects 1118615 and 139656), Nordic Center for Excellence (CRAICC), European Commission seventh Framework program (ACTRIS, contract no. 262254; PEGASOS, contract no. 265148) and the Eurostars Programme under contract no. E!6911 (GANS project).
REFERENCES
- Agarwal, J. K., and Sem, G. J. (1980). Continuous-Flow, Single-Particle-Counting Condensation Nucleus Counter. J. Aerosol Sci., 11:343, doi: 10.1016/0021-8502(80)90042-7.
- Ahn, K. H., and Liu, B. Y. H. (1990). Particle Activation and Droplet Growth-Processes in Condensation Nucleus Counter. 2. Experimental-Study. J. Aerosol Sci., 21:263–275, doi: 10.1016/0021-8502(90)90009-M.
- Bartz, H., Fissan, H., Helsper, C., Kousaka, Y., Okuyama, K., Fukushima, N., Keady, P. B., Kerrigan, S., Fruin, S. A., McMurry, P. H., Pui, D. Y. H., and Stolzenburg, M. R. (1985). Response Characteristics for 4 Different Condensation Nucleus Counters to Particles in the 3-50 Nm Diameter Range. J. Aerosol Sci., 16:443–456, doi: 10.1016/0021-8502(85)90056-4.
- Gamero-Castano, M., and de la Mora, J. F. (2000). A Condensation Nucleus Counter (CNC) Sensitive to Singly Charged Sub-Nanometer Particles. J. Aerosol Sci., 31:757–772, doi: 10.1016/S0021-8502(99)00555-8.
- Giechaskiel, B., Wang, X., Gilliland, D., and Drossinos, Y. (2011). The Effect of Particle Chemical Composition on the Activation Probability in n-butanol Condensation Particle Counters. J. Aerosol Sci., 42:20–37, doi: 10.1016/j.jaerosci.2010.10.006.
- Gormley, P.G., and Kennedy, M. (1949). Diffusion from a Stream Flowing Through a Cylindrical Tube, in Proceedings of the Royal Irish Academy, 52A, pp. 163–169.
- Hering, S. V., Stolzenburg, M. R., Quant, F. R., Oberreit, D. R., and Keady, P. B. (2005). A Laminar-Flow, Water-Based Condensation Particle Counter (WCPC). Aerosol Sci. Tech., 39:659–672, doi: 10.1080/02786820500182123.
- Herrmann, W., Eichler, T., Bernardo, N., and Fernández de la Mora, J. (2000). Turbulent Transition Arises at Reynolds Number 35,000 in a Short Vienna Type DMA with a Large Laminarization Inlet. Abstract AAAR Conference, 15B5.
- Iida, K., Stolzenburg, M. R., McMurry, P. H., Smith, J. N., Quant, F. R., Oberreit, D. R., Keady, P. B., Eiguren-Fernandez, A., Lewis, G. S., Kreisberg, N. M., and Hering, S. V. (2008). An Ultrafine, Water-based Condensation Particle Counter and its Evaluation Under Field Conditions. Aerosol Sci. Tech., 42:862–871, doi: 10.1080/02786820802339579.
- Iida, K., Stolzenburg, M. R., and McMurry, P. H. (2009). Effect of Working Fluid on Sub-2 nm Particle Detection with a Laminar Flow Ultrafine Condensation Particle Counter. Aerosol Sci. Tech., 43:81–96, doi: 10.1080/02786820802488194.
- Jiang, J. K., Zhao, J., Chen, M. D., Eisele, F. L., Scheckman, J., Williams, B. J., Kuang, C. A., and McMurry, P. H. (2011). First Measurements of Neutral Atmospheric Cluster and 1–2 nm Particle Number Size Distributions During Nucleation Events. Aerosol Sci. Tech., 45:Ii-V, doi: 10.1080/02786826.2010.546817.
- Kangasluoma, J., Junninen, H., Lehtipalo, K., Mikkila, J., Vanhanen, J., Attoui, M., Sipila, M., Worsnop, D., Kulmala, M., and Petaja, T. (2013). Remarks on Ion Generation for CPC Detection Efficiency Studies in Sub-3-nm Size Range. Aerosol Sci. Tech., 47:556–563, doi: 10.1080/02786826.2013.773393.
- Kangasluoma, J., Kuang, C., Wimmer, D., Rissanen, M. P., Lehtipalo, K., Ehn, M., Worsnop, D. R., Wang, J., Kulmala, M., and Petaja, T. (2014). Sub-3 nm Particle Size and Composition-Dependent Response of a Nano-CPC Battery. Atmos. Meas. Tech., 7:689–700, doi: 10.5194/amt-7-689-2014.
- Kangasluoma, J., Attoui, M., Junninen, H., Lehtipalo, K., Samodurov, A., Korhonen, F., Sarnela, N., Schmidt-Ott, A., Worsnop, D., Kulmala, M., and Petäjä, T. (2015). Sizing of Neutral Sub 3 nm Tungsten Oxide Clusters using Airmodus Particle Size Magnifier. Journal of Aerosol Science, 87:53–62.
- Kim, C. S., Okuyama, K., and Shimada, M. (2002). Performance of a Mixing-type CNC for Nanoparticles at Low-Pressure Conditions. J. Aerosol Sci., 33:1389–1404, Pii S0021-8502(02)00092-7, doi: 10.1016/S0021-8502(02)00092-7.
- Kuang, C., Chen, M., Zhao, J., Smith, J., McMurry, P. H., and Wang, J. (2012a). Size and Time-Resolved Growth Rate Measurements of 1 to 5 nm Freshly Formed Atmospheric Nuclei. Atmos. Chem. Phys., 12:3573–3589, doi: 10.5194/acp-12-3573-2012.
- Kuang, C. A., Chen, M. D., McMurry, P. H., and Wang, J. (2012b). Modification of Laminar Flow Ultrafine Condensation Particle Counters for the Enhanced Detection of 1 nm Condensation Nuclei. Aerosol Sci. Tech., 46:309–315, doi: 10.1080/02786826.2011.626815.
- Kulmala, M., Mordas, G., Petaja, T., Gronholm, T., Aalto, P. P., Vehkamaki, H., Hienola, A. I., Herrmann, E., Sipila, M., Riipinen, I., Manninen, H. E., Hameri, K., Stratmann, F., Bilde, M., Winkler, P. M., Birmili, W., and Wagner, P. E. (2007). The Condensation Particle Counter Battery (CPCB): A New Tool to Investigate the Activation Properties of Nanoparticles. J. Aerosol Sci., 38:289–304, doi: 10.1016/j.jaerosci.2006.11.008.
- Kulmala, M., Kontkanen, J., Junninen, H., Lehtipalo, K., Manninen, H. E., Nieminen, T., Petaja, T., Sipila, M., Schobesberger, S., Rantala, P., Franchin, A., Jokinen, T., Jarvinen, E., Aijala, M., Kangasluoma, J., Hakala, J., Aalto, P. P., Paasonen, P., Mikkila, J., Vanhanen, J., Aalto, J., Hakola, H., Makkonen, U., Ruuskanen, T., Mauldin, R. L., Duplissy, J., Vehkamaki, H., Back, J., Kortelainen, A., Riipinen, I., Kurten, T., Johnston, M. V., Smith, J. N., Ehn, M., Mentel, T. F., Lehtinen, K. E. J., Laaksonen, A., Kerminen, V. M., and Worsnop, D. R. (2013). Direct Observations of Atmospheric Aerosol Nucleation. Science, 339:943–946, doi: 10.1126/science.1227385.
- Kurten, A., Curtius, J., Helleis, F., Lovejoy, E. R., and Borrmann, S. (2007). Development and Characterization of an Ion Trap Mass Spectrometer for the On-Line Chemical Analysis of Atmospheric Aerosol Particles. Int. J. Mass. Spectrom., 265:30–39, doi: 10.1016/j.ijms.2007.05.007.
- Lehtipalo, K., Leppä, J., Kontkanen, J., Kangasluoma, J., Franchin, A., Wimmer, D., Schobesberger, S., Junninen, H., Petäjä, T., Sipilä, M., Mikkilä, J., Vanhanen, J., Worsnop, D. R., and Kulmala, M. (2014). Methods for Determining Particle Size Distribution and Growth Rates Between 1 and 3 nm using the Particle Size Magnifier. Boreal Env. Res, 19 (Suppl. B):215–236.
- Magnusson, L. E., Koropchak, J. A., Anisimov, M. P., Poznjakovskiy, V. M., and de la Mora, J. F. (2003). Correlations for Vapor Nucleating Critical Embryo Parameters. J. Phys. Chem. Ref. Data, 32:1387–1410, doi: 10.1063/1.1555590.
- McMurry, P. H. (2000). The History of Condensation Nucleus Counters. Aerosol Sci. Tech., 33:297–322, doi: 10.1080/02786820050121512.
- McMurry, P. H., Kulmala, M., and Worsnop, D. R. (2011). Special Issue on Aerosol Measurements in the 1 nm Range. Aerosol Sci. Technol., 45, i.
- Mertes, S., Schroder, F., and Wiedensohler, A. (1995). The Particle-Detection Efficiency Curve of the Tsi-3010 Cpc as a Function of the Temperature Difference between Saturator and Condenser. Aerosol Sci. Tech., 23:257–261, doi: 10.1080/02786829508965310.
- OpenFOAM (2015). The Open Source CFD Toolbox, available at: http://www.openfoam.org (accessed 26 November 2014).
- Peineke, C., Attoui, M. B., and Schmidt-Ott, A. (2006). Using a Glowing Wire Generator for Production of Charged, Uniformly Sized Nanoparticles at High Concentrations. J. Aerosol Sci., 37:1651–1661, doi: 10.1016/j.jaerosci.2006.06.006.
- Peineke, C., and Schmidt-Ott, A. (2008). Explanation of Charged Nanoparticle Production from Hot Surfaces. J. Aerosol Sci., 39:244–252, doi: 10.1016/j.jaerosci.2007.12.004.
- Reischl, G. P., Makela, J. M., Karch, R., and Necid, J. (1996). Bipolar Charging of Ultrafine Particles in the Size Range Below 10 nm. J. Aerosol Sci., 27:931–949, doi: 10.1016/0021-8502(96)00026-2.
- Russell, L. M., Zhang, S. H., Flagan, R. C., Seinfeld, J. H., Stolzenburg, M. R., and Caldow, R. (1996). Radially Classified Aerosol Detector for Aircraft-Based Submicron Aerosol Measurements. J. Atmosp. Oceanic Technol., 13:598–609, doi: 10.1175/1520-0426(1996)013<0598:Rcadfa>2.0.Co;2.
- Scheibel, H. G., and Porstendorfer, J. (1986). Counting Efficiency and Detection Limit of Condensation Nuclei Counters for Submicrometer Aerosols .1. Theoretical Evaluation of the Influence of Heterogeneous Nucleation and Wall Losses. J. Colloid. Interf. Sci., 109:261–274, doi: 10.1016/0021-9797(86)90301-2.
- Seto, T., Okuyama, K., deJuan, L., and delaMora, J. F. (1997). Condensation of Supersaturated Vapors on Monovalent and Divalent Ions on Varying Size. J. Chem. Phys., 107:1576–1585, doi: 10.1063/1.474510.
- Sgro, L. A., and de la Mora, J. F. (2004). A Simple Turbulent Mixing CNC for Charged Particle Detection Down to 1.2 nm. Aerosol Sci. Tech., 38:1–11, doi: 10.1080/02786820490247560.
- Sipilä, M., Lehtipalo, K., Attoui, M., Neitola, K., Petaja, T., Aalto, P. P., O’Dowd, C. D., and Kulmala, M. (2009). Laboratory Verification of PH-CPC's Ability to Monitor Atmospheric Sub-3 nm Clusters. Aerosol Sci. Tech., 43:126–135, doi: 10.1080/02786820802506227.
- Stolzenburg, M. R., and McMurry, P. H. (1991). An Ultrafine Aerosol Condensation Nucleus Counter. Aerosol Sci. Tech., 14:48–65, doi: 10.1080/02786829108959470.
- Stratmann, F., Herrmann, E., Petaja, T., and Kulmala, M. (2010). Modelling Ag-Particle Activation and Growth in a TSI WCPC Model 3785. Atmos. Meas. Tech., 3:273–281.
- Su, Y. F., Cheng, Y. S., Newton, G. J., and Yeh, H. C. (1990). Counting Efficiency of the Tsi Model 3020 Condensation Nucleus Counter. Aerosol Sci. Tech., 12:1050–1054, doi: 10.1080/02786829008959414.
- Vanhanen, J., Mikkila, J., Lehtipalo, K., Sipila, M., Manninen, H. E., Siivola, E., Petaja, T., and Kulmala, M. (2011). Particle Size Magnifier for Nano-CN Detection. Aerosol Sci. Tech., 45:533–542, doi: 10.1080/02786826.2010.547889.
- Weber, R. J., Stolzenburg, M. R., Pandis, S. N., and McMurry, P. H. (1998). Inversion of Ultrafine Condensation Nucleus Counter Pulse Height Distributions to Obtain Nanoparticle (Similar to 3–10 nm) Size Distributions. J. Aerosol Sci., 29:601–615, doi: 10.1016/S0021-8502(97)10026-X.
- Weller, H. G., Tabor, G., Jasak, H., and Fureby, C. (1998). A Tensorial Approach to Computational Continuum Mechanics Using Object-Oriented Techniques. Comp. Phys., 12:620–631, doi: 10.1063/1.168744.
- Wiedensohler, A., Orsini, D., Covert, D. S., Coffmann, D., Cantrell, W., Havlicek, M., Brechtel, F. J., Russell, L. M., Weber, R. J., Gras, J., Hudson, J. G., and Litchy, M. (1997). Intercomparison Study of the Size-Dependent Counting Efficiency of 26 Condensation Particle Counters. Aerosol Sci. Tech., 27:224–242, doi: 10.1080/02786829708965469.
- Wilson, J. C., Blackshear, E. D., and Hyun, J. H. (1983). An Improved Continuous-Flow Condensation Nucleus Counter for Use in the Stratosphere. J. Aerosol Sci., 14:387–391, doi: 10.1016/0021-8502(83)90143-X.
- Wimmer, D., Lehtipalo, K., Franchin, A., Kangasluoma, J., Kreissl, F., Kurten, A., Kupc, A., Metzger, A., Mikkila, J., Petaja, T., Riccobono, F., Vanhanen, J., Kulmala, M., and Curtius, J. (2013). Performance of Diethylene Glycol-Based Particle Counters in the Sub-3 nm Size Range. Atmos. Meas. Tech., 6:1793–1804, doi: 10.5194/amt-6-1793-2013.
- Winkler, P. M., Steiner, G., Vrtala, A., Vehkamaki, H., Noppel, M., Lehtinen, K. E. J., Reischl, G. P., Wagner, P. E., and Kulmala, M. (2008). Heterogeneous Nucleation Experiments Bridging the Scale from Molecular Ion Clusters to Nanoparticles. Science, 319:1374–1377, doi: 10.1126/science.1149034.
- Winkler, P. M., Vrtala, A., Steiner, G., Wimmer, D., Vehkamaki, H., Lehtinen, K. E. J., Reischl, G. P., Kulmala, M., and Wagner, P. E. (2012). Quantitative Characterization of Critical Nanoclusters Nucleated on Large Single Molecules. Phys. Rev. Lett., 108, Art. No. 085701, doi: 10.1103/Physrevlett.108.085701.