ABSTRACT
The process of particle formation from evaporating droplets containing more than one solute was studied. Two-component microparticles were produced using a piezoceramic dispenser with an inner diameter of 30 µm. Initial droplets had a diameter in the range of 70–85 µm and contained sodium nitrate and potassium nitrate in different molar ratios of 30:70, 50:50, and 70:30, corresponding to weight ratios of 26.5:73.5, 45.7:54.3, and 66.2:33.8, in the form of aqueous solutions with initial concentrations of 1 or 10 mg/ml. The monodisperse droplets were dried in a dry laminar gas flow with temperatures of 50°C or 100°C. Different initial conditions affected the particle formation process and the particle morphology. The diameter of the final dried microparticles ranged from 4 to 10 µm. Their density varied from 1250 to 1950 mg/ml. The formulation and process conditions determined the distribution of chemical components in the dried microparticles, especially their surface composition as determined by energy-dispersive X-ray spectroscopy. The distribution of the chemical components was theoretically explained using characteristic times for the crystallization kinetics of the drying process. It was shown that the solute that reached supersaturation first formed most of the outer shell of the microparticles.
© 2016 American Association for Aerosol Research
EDITOR:
Introduction
The role of respiratory drug delivery in effective interventions for lung diseases continues to attract much interest (Beck-Broichsitter et al. Citation2014; Hoe et al. Citation2014; Lechuga-Ballesteros and Miller Citation2015; Zhou et al. Citation2015). The diameter of drug-containing particles delivered to the lung is commonly in the micrometer range (Longest et al. Citation2012; Islan et al. Citation2015). Particles with diameters between 1 and 5 µm tend to reach the lung upon delivery and can release the drug at the desired location (Longest et al. Citation2012). Another important property of medicinal microparticles is their morphology (Ko et al. Citation2002); microparticles with rough surfaces decrease cohesion forces (Pilcer and Amighi Citation2010; Aman et al. Citation2012). The solid phase is another parameter with an impact on the performance of respiratory dosage forms (Clark Citation1991; Pasquali et al. Citation2006). For example, some amorphous materials may show a low storage stability and higher reactivity (Pasquali et al. Citation2006).
The area of research describing and controlling the particle formation process of respiratory drugs is called particle engineering (Ragab and Rohani Citation2009). The particle formation process is the transformation of a solution droplet into a dry particle (Vicente et al. Citation2013). Several qualities make spray drying the preferred technique for the production of microparticles for respiratory drug delivery: minimal loss of activity of chemicals, compatibility with a wide range of solutions or suspensions, and control over important properties of the final particles (Filková et al. Citation2014). For a fundamental understanding of the microdroplet drying process in a spray dryer, experimental observation of model systems has proven fruitful. In particular, the experimental method using a free falling, monodisperse droplet chain shows several favorable qualities for analyzing the particle formation process: an ability to study rapid processes, representative droplet dimension in the micrometer range, and representative heat and mass transfer on isolated droplets (Vehring et al. Citation2007). A piezoceramic dispenser is generally used to produce such a monodisperse droplet chain because it is capable of doing so with high precision (Pechkova et al. Citation2015).
The particle formation process for single-component solution droplets has been investigated quite thoroughly (Shekunov and York Citation2000; Hakuta et al. Citation2003; Shoyele and Cawthorne Citation2006; Vehring et al. Citation2007; Zhang et al. Citation2011; Boraey et al. Citation2013; Vladisavljević et al. Citation2014; Baldelli et al. Citation2015). In contrast, few researchers have investigated particle formation from evaporating droplets with more than one chemical component (Zhang and Stangle Citation1994; Zhu and Hayward Citation2012; Hoe et al. Citation2014), which is the more relevant case for most formulations in respiratory drug delivery. The characteristics of multicomponent particles are strongly dependent on the composition of their surface or shell (De Villiers et al. Citation2002). Surface properties are fundamental for the delivery of respiratory drugs (Gonzalez et al. Citation1991; Sung et al. Citation2007) since they can influence both the location at which the drugs are delivered and the release profile of the drugs (Ai et al. Citation2005). As a consequence, particle formation studies that can reliably predict which components form the surface of microparticles are important for improving particulate dosage forms for respiratory drug delivery.
Previous particle formation studies (Vicente et al. Citation2013; Baldelli et al. Citation2015; Belotti et al. Citation2015) have focused on non-crystallizing systems, where the solute precipitates into an amorphous solid during the process. Such systems are relatively well understood (Vehring et al. Citation2007; Vicente et al. Citation2013; Boraey and Vehring Citation2014; Hoe et al. Citation2014). In this case, many properties of the final dried microparticles can be predicted from an analysis of the Peclet number (Vicente et al. Citation2013). The Peclet number is the ratio of the evaporation rate, indicating the speed at which the droplet shrinks, and the diffusion coefficient, indicating the speed at which the solute diffuses away from the surface (Boraey et al. Citation2013). It has been shown that low Peclet numbers predict solid microparticles, typically with a smooth surface and spherical shape (Vehring et al. Citation2007). This prediction is valid only for non-crystalline systems (Baldelli et al. Citation2016) because the Peclet number does not capture the impact of crystallization kinetics on particle formation. However, it is known from electron micrographs that crystals may grow on the surface of droplets, eventually forming a crystalline shell. Several respiratory drugs have a known tendency to crystallize or have been formulated with crystallizing excipients (Patravale and Kulkarni Citation2004; Niwa et al. Citation2012; Hoe et al. Citation2014; Young et al. Citation2015). In this case, the particle formation process includes a crystallization sub-process. The effect of crystallization on the particle formation process is still mostly unknown, the main reasons being the complexity of crystallization theory (Zhang et al. Citation2014) and the rapid kinetics involved in the drying of microdroplets. In order to predict the properties of spray-dried crystalline microparticles, future models describing the particle formation process need to incorporate theoretical approaches to the nucleation and crystallization processes. Furthermore, it has been difficult to experimentally determine crystallization kinetics in drying microdroplets, e.g., the time at which nucleation commences and the time at which crystals start to grow. Thus, further studies of the formation process of crystalline particles are needed to gain a better understanding of these important systems.
In this article, a comprehensive evaluation of the particle formation process for a crystallizing system is achieved by combining an experimental and a theoretical approach. The experimental approach uses a monodisperse droplet chain as a model system. The theoretical approach applies a relatively simple model that can predict the internal distribution of components in an evaporating droplet, the time they reach saturation on the surface, and the shell thickness of the resulting particle (Boraey and Vehring Citation2014). While this model has not been fully validated for crystallizing multi-component systems, it predicts that the time to reach saturation on the surface is the main variable determining the sequence in which components can form a shell (Baldelli et al. Citation2015). In the case of evaporating droplets composed of more than one solute, it was hypothesized that the solute requiring less time to reach saturation had a higher probability of nucleating and crystallizing on the surface first, thus forming the shell (Boraey and Vehring Citation2014).
Experimental section
Chemicals
The chemical components chosen were sodium nitrate (NaNO3), potassium nitrate (KNO3), and deionized water (catalog number 38796 – 1L, Sigma Aldrich, St Louis, MO, USA). The properties of NaNO3 (catalog number 221341–500G, Sigma Aldrich, St Louis, MO, USA) that are important for this study are its molecular weight of 84.99 g/mol, true density of 2260 mg/ml, glass transition temperature of 185–215°C, melting point of 306°C (Kracek et al. Citation1931), and aqueous solubility of 740 mg/ml and 860 mg/ml (Holden and Morrison Citation1982) at temperatures of 18.7°C and 31.9°C, respectively. The corresponding properties of KNO3 (catalog number 221295–500G, Sigma Aldrich, St Louis, MO, USA) are its molecular weight of 101.1 g/mol, true density of 2109 mg/ml, glass transition temperature of 128°C, melting point of 334°C, and aqueous solubility of 320 mg/ml and 570 mg/ml (Oriakhi Citation2009) at temperatures of 18.7°C and 31.9°C, respectively. Since the evaporation rate and, consequently, the wet bulb temperature are almost constant during the evaporation of each droplet prior to shell formation, the solubility is assumed to be constant for each drying gas temperature.
Experimental approach
Monodisperse droplet chain
Binary solutions of NaNO3 and KNO3 were generated that differed in the molar percentage of NaNO3, i.e., 30%, 50%, and 70%. The production of monodisperse microparticles has been explained in detail in a previous publication (Baldelli et al. Citation2015). Briefly, the monodisperse droplet chain was generated using a piezoceramic dispenser with an inner diameter of 30 µm. The initial droplet diameter was in the range of 70–75 µm. The droplets were injected into a flow tube with a temperature regulated gas flow. The monodisperse droplet chain was illuminated with a diode laser, and images were recorded with a digital camera to determine the distance between two consecutive droplets. From the distance, the aerodynamic diameter could be derived as a function of time as described below. The resulting dry microparticles were collected on a filter for further ultramicroscopy and Raman spectroscopic analysis.
Microparticles characterization: Final properties
An important property of the dried microparticles is the spatial distribution of the two chemical components on the surface and in the shell. The analysis of the near-surface component distribution was achieved using Scanning Electron Microscopy with X-ray spectroscopy (SEM/EDX) (Zeiss Sigma FESEM with EDX & EBSD, Zeiss, Jena, Germany). In general, EDX is not a surface-specific technique, but it can be preferentially adapted to provide information about elemental composition near the surface. To this end, the interaction volume was kept as small as possible by selecting minimal overvoltage. In this study, the highest X-ray energy used was 3.59 keV (potassium, Kβ). To provide reliable excitation, all samples were irradiated with electron beams with an energy of 5 keV (with one exception: 10 keV was used in the case with a drying temperature of 50°C, 10 mg/ml initial concentration and 1:1 molar ratio of the components). For this electron energy, the penetration depth into a sample coated with a 20 nm gold layer was on the order of 100 nm, and the X-ray signal was strongly weighted toward the surface (Robinson Citation1991). The diameters of the dry microparticles were in the range of 4–10 µm with a shell thickness larger than 0.1 μm in all cases. SEM/EDX measures characteristic X-ray intensity. Furthermore, every element has a standard intensity, calibrated for by the instrument manufacturer. The ratio between the measured intensity and the standard intensity is equivalent to the ratio of the mass or weight fractions (Goldstein et al. Citation2012), which can be converted to mole fractions using the molar mass of each component.
To measure the distribution of the components across the shell of the microparticles with higher spatial resolution, Transmission Electron Microscopy with X-ray spectroscopy (S/TEM) (CM 20 FEG, TEM S/TEM, Philips, Amsterdam, Netherlands) was used. In bio-techniques the characteristic X-ray lines were Kα and Kβ, with an emission energy of 1.041 keV and 1.071 keV for Na, and 3.312 keV and 3.59 keV for K, respectively (Sharma Citation2008).
Other properties analyzed were diameter, morphology, and solid phase. The diameter and the morphology of the final dried microparticles were measured using a Scanning Electron Microscope (SEM) (SEM LEO 1430, Zeiss, Jena, Germany). The images derived from the SEM were analyzed with the software ImageJ (ImageJ, Imaging Processing and Analysis in Java, National Institute of Health, 1997, Bethesda, MD, USA). To determine shell thickness and interior morphology, the microparticles were cut and imaged using a Helium Ion Microscope (HiM) (Zeiss Orion Helium Ion Microscope, Zeiss, Jena, Germany). The solid phase of the particles was analyzed using a custom macro Raman spectroscopy system with low-frequency shift capability. Details of this instrument and the associated methodology have been described previously (Wang et al. Citation2014).
Samples used for the TEM analysis
Microparticles were collected on a frit placed on a hollow Scanning Electron Microscopy (SEM) stub. Of these, a small quantity, about 1 mg, was transferred into a pencil-shaped mold (Catalog number 70902, flat embedding mold, Electron Microscopy Sciences, Hatfield, PA, USA). The microparticles were pushed with sharp tweezers (SS140-A Dumont, Ted Pella, Redding, CA, USA) to the tip of the pencil-shaped mold. The mold was then filled with an embedding medium (Catalog number 14300, Low Viscosity Embedding Media Spurr's Kit, Electron Microscopy Sciences, Hatfield, PA, USA). The embedding medium was composed of four components: epoxy ERL 4221, diglycidyl ether of polypropylene glycol (DER 736), nonenyl succinic anhydride (NSA), and the accelerator dimethylaminoethanol (DMAE). The embedding medium was cured in an oven (VWR 140, VWR, Radnor, PA, USA) at 70°C for 16–20 h. A section of the tip of the dried mold was chopped every 90 nm with an ultramicrotome (Ultracut E, Reichert-Jung, Leica Biosystems, Wetzlar, Germany), and the sectioned material was collected on a Transmission Electron Microscopy (TEM) grid.
Theoretical approach
A theoretical particle formation model has been described in detail in previous publications (Vehring et al. Citation2007; Boraey and Vehring Citation2014; Baldelli et al. Citation2015). For crystallizing multi-component systems, the parameters that can predict the onset and kinetics of the crystallization process are the most critical. Boraey et al. (Citation2013) introduced a description for the particle formation process of a droplet composed of more than one solute. The concentration of all solutes increases during evaporation because of loss of solvent. It is assumed that the solute that saturates first during the particle formation process forms a crystalline shell on the microparticle. Equation (Equation1[1] ) shows the expression for the time, ts,j, at which each component, j, reaches saturation on the surface of the evaporating droplet. d0 is the initial diameter, C0,j is the initial concentration, Csol,j is the solubility, and Ej is the enrichment of solute j on the surface of the droplet, relative to its average concentration. The surface enrichment is caused by the limited speed of solute diffusion away from the receding droplet surface. Expressions for E have been reported before (Boraey and Vehring Citation2014):
[1]
From Equation (Equation1[1] ), it can be derived that, all else being equal, the solute with lower solubility tends to precipitate earlier than the others. The surface enrichment is dependent on the diffusion coefficient of the solute in the liquid phase and the evaporation rate of the droplet. Both of these two parameters are strongly affected by the wet bulb temperature of the droplet. In addition, the time to reach saturation depends on the initial concentration of the solute in the solution, Equation (Equation1
[1] ). The theoretical model used to derive Equation (Equation1
[1] ) assumes a constant evaporation rate (Vehring et al. Citation2007; Boraey and Vehring Citation2014; Baldelli et al. Citation2015) but can be improved by applying it stepwise, assuming a constant evaporation rate for each time step only. This modification allows for corrections to material properties and other parameters that change as a function of time (Baldelli et al. Citation2015). In this case, the time to saturation is reached when the surface concentration of a solute reaches the solubility of the solute at the present conditions. A second parameter important for the kinetics of the crystallization process is the precipitation window, Δts, which is the difference between the time needed for the evaporation process to become complete and the time required to reach saturation. The evaporation process is assumed to be complete when the measured aerodynamic diameter no longer decreases. A related parameter, the crystallization window, Δtc, is the difference between the time needed for a particle to reach a constant aerodynamic diameter and the time for earliest possible onset of crystallization, tc. The time for crystallization defines the point in time when the surface concentration reaches sufficient supersaturation for crystals to nucleate and grow. Previous studies have determined the required supersaturation level in aqueous solutions at which crystals start to grow (Tang and Munkelwitz Citation1994; Graber et al. Citation1999). This level of supersaturation also changes according to the wet bulb temperature of the solution. For wet bulb temperatures of 18.7°C and 31.8°C, which correspond to the experimental conditions of this study, NaNO3 starts to crystallize at a supersaturation of 15.7 and 17.1 molar percentages or 97 (720 mg/ml) and 99 (850 mg/ml) weight percentages, respectively. For the same wet bulb temperatures, KNO3 starts to crystallize at a supersaturation of 8.2 and 9.1 molar percentages or 47 (155 mg/ml) and 60 (345 mg/ml) weight percentages, respectively (Graber et al. Citation1999).
From an analysis of images of the monodisperse droplet chains, the distance between two consecutive droplets was obtained, and from these data, along with the frequency at which each droplet was injected in the flow tube, the velocity of each droplet was derived. The settling velocity of the droplets or particles was calculated by subtracting the velocity of the gas flow from the total velocity of each droplet. Using Stokes' law, the aerodynamic diameter for each droplet position was derived from the settling velocity (Baldelli et al. Citation2015). Once the aerodynamic diameter was known as a function of time, the evaporation rate, and the droplet or particle mass, solute concentrations, and overall particle density were derived from a mass balance. The diffusion coefficient and viscosity were then adjusted to take into consideration the instantaneous concentrations and droplet temperature. With this information, the instantaneous Peclet number was calculated (Vehring et al. Citation2007), and surface enrichment and finally surface concentration emerged as a function of time.
The density of the evaporating droplets or particles was calculated using two different methods depending on the phase of the drying process, specifically the time at which a shell forms. Shell formation was assumed to occur when the volume equivalent diameter of the evaporating droplets was equal to the diameter of the final dried particle. Before shell formation, Equation (Equation2[2] ) was used, where da,0 is the initial aerodynamic diameter of the droplet, da,j is the aerodynamic diameter at time step j, and ρ* is the standard density [1 g/cm3]. A and B are parameters derived from the trend of the mass fraction and viscosity for the solutes used (Isono Citation1984; Mahiuddin and Ismail Citation1996). Both values of A and B depend on the wet bulb temperature: values of A vary from 0.0019 to 0.002 ml/mg for NaNO3 and 0.0027 to 0.0031 ml/mg for KNO3 at 18.7°C and 31.8°C, and values of B vary from −2.03 to −1.98 for NaNO3 and −2.76 to −3.01 for KNO3 at 18.7°C and 31.8°C (Isono Citation1984). This method is obtained from a mass balance assuming that the particles lack internal and external voids. For each time step, i, Equation (Equation2
[2] ) provides the particle density assuming only one component, j, is present. In Equation (Equation2
[2] ), the droplet or particle density, ρP, is equal to the true density, ρT, since no internal or external voids are involved:
[2]
Subsequently, the total particle density of the multicomponent droplet was approximated using Equation (Equation3[3] ), where Yj is the mass fraction of each component:
[3]
After the shell formation, Equation (Equation4[4] ) was used to determine the density of the droplets or particles. In Equation (Equation4
[4] ), dv,f is the diameter of the final dried particles:
[4]
The instantaneous diffusion coefficient, which was necessary to calculate the Peclet number and surface enrichment, was derived as a function of viscosity using the Stokes–Einstein equation (Evans et al. Citation2013; Slominski et al. Citation2014). The viscosity for each component, ηj, was approximated using Equation (Equation5[5] ) (Laliberté Citation2007). In Equation (Equation5
[5] ), α1, α2, α3, α4, α5, and α6 are constants (Laliberté Citation2007), different for NaNO3 and KNO3, while ww is the water content, and Tw is the wet bulb temperature:
[5]
The total viscosity, η, was then obtained using the Gambill equation for a mixture of liquids (Gambill Citation1959).
Results and discussion
Twelve cases, shown in , were studied to understand the influence of the initial conditions of the particle formation process on the distribution of chemical components in the final dried particles. The initial total solution concentration, C0, was either 1 or 10 mg/ml, and the drying gas temperature, TGas, was either 50°C or 100°C. Furthermore, the 12 cases differed in composition, i.e., the molar percentages of NaNO3 and KNO3 were 30:70, 50:50, and 70:30, respectively. The weight percentages were thus 26.5:73.5, 45.7:54.3, and 66.2:33.8, respectively.
Table 1. SEM images showing surface morphology, including crystal size, of the dried microparticles.
NaNO3 and KNO3 were chosen for their strong tendency to crystallize. Any material that tends to crystallize and has well-known properties, such as solubility, viscosity, and supersaturation state, at which it starts to crystallize, can be used. In the experimental design of this study, it was assumed that these materials would likely crystallize even during a fast microdroplet evaporation process. It was hypothesized that the crystallization would commence on or near the surface and then lead to the formation of a shell and of interior voids in the particles. For some of the produced microparticles the crystalline nature is obvious from their morphology, shown in . In three of the cases (top row left and right, and bottom row right), well-defined domains with a size of a few hundred nanometers are clearly recognizable on the surface. These domains are very likely individual crystals. In the other cases, discerning individual crystals is challenging. If present, either they could be too small to be clearly observable or they could have merged to form a continuous crystalline shell.
Further evidence for the proposed particle formation mechanism is provided in , which shows particle density as a function of time during the particle formation process for four selected cases with equal molar percentages of the two components. In the first drying phase (full symbol curves), in each of the cases considered, the density of the solution droplet increased as the water evaporated. This result was achieved using the data processing method assuming the absence of any voids in the particles, Equation (Equation2[2] ). Since the exact point when a void first appears is unknown, the calculation was carried on until the true densities of the solutes were reached. This extrapolation represents the case of a hypothetical, non-crystallizing system. However, this calculation predicts unrealistically small final particle diameters, inconsistent with the size of the dry particles determined by SEM. Therefore, a shell and, subsequently, voids must have formed. In , the larger and brighter data point approximates the time of shell formation, i.e., the point in time when the diameter of the droplet equals the final volume equivalent diameter of the dry particle. After this point, it is increasingly likely that the density trend follows Equation (Equation4
[4] ) (open symbols), which is based on the properties of the final particles. For all the curves shown, the predicted point of shell formation is at a density below the true density of the solutes used, indicating the presence of voids or solvent trapped in the formed shell in the evaporating microparticles. After shell formation, the trapped solvent continues to evaporate without a further change in the droplet diameter, which explains the observed drop in particle density.
Figure 1. The particle density as a function of time during evaporation and particle formation for droplets with equal molar amounts of NaNO3 and KNO3. The full symbol curves were calculated assuming no voids in the particle, i.e., a non-crystallizing system. The open symbol curves were calculated using the final diameter of the actual dry particles. The larger data point approximates the onset of shell formation.
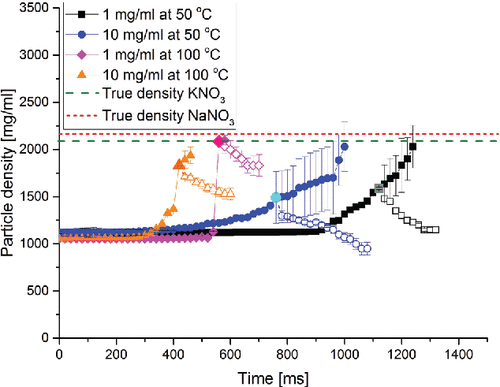
It is known that some non-crystallizing systems can also form hollow, low-density particles (Baldelli et al. Citation2015). In these systems, shell formation due to a very large surface enrichment correlates with large Peclet numbers. All 12 cases studied here, however, show a small Peclet number for most of the evaporation process, thereby indicating comparatively little enrichment on the surface. According to previous studies (Vehring et al. Citation2007; Boraey et al. Citation2013), microparticles with a high density and without voids would result in this case. This is not observed here, ruling out this mechanism.
This interpretation is consistent with the HiM images of cut particles shown in ; all the microparticles shown here contain varying amounts of voids. The voids in the particle shown in the lower left position are difficult to see because they are quite small. A comparison of the particles in the top row, which were dried at 50°C, with the ones in the bottom row, dried at 100°C, yields a result that is consistent with the trend shown in : higher evaporation rates cause denser particles with less void volume. For non-crystallizing systems, the opposite effect is expected: higher evaporation rates should lead to higher Peclet numbers, higher surface enrichment, and decreased particle density (Baldelli et al. Citation2015). Two peculiar features of the particles in require an explanation. First, the surface morphology of the microparticles shown seems to differ from that shown in . This is an artifact caused by the differing coating requirements for HiM and SEM. For HiM experiments, the microparticles were sputtered with a gold layer of about 100 nm thickness to securely attach them to the stub and thereby prevent the high-energy ion beam from removing them while cutting them, as it otherwise risked doing. For the SEM experiments, by contrast, the microparticles were sputtered with a much thinner gold layer of 20 nm; thus, the structure and the details of the surface were better recorded. The second peculiarity requiring explanation concerns the case with molar percentages of 50 NaNO3 and 50 KNO3 at 50°C (middle particle, top row), where the microparticle appears different than in the other cases. This section was probably not taken at the center of the microparticle; as a consequence, part of the crystal structure in the microparticle may have collapsed.
Table 2. Helium ion microscope images of sectioned microparticles dried from droplets with an initial concentration of 10 mg/ml.
The analysis of the solid phase of the dried microparticles, shown in , unambiguously verifies the previous interpretation of the results. lists the low-frequency shift Raman spectra of the produced microparticles together with the reference spectra of the crystalline raw materials. Both NaNO3 and KNO3 show characteristic lattice peaks in the low-frequency area of their Raman spectra. NaNO3 has its vibrational lattice modes at 98 and 195 cm−1 and KNO3 at 57 and 85 cm−1 (Shen et al. Citation1975; Brooker Citation1978). These modes are absent in amorphous material, which lacks an ordered crystal lattice.
Table 3. Low frequency shift Raman spectra of dried microparticles compared with the reference spectra of crystalline NaNO3 and crystalline KNO3. Cases with an initial concentration of 10 mg/ml are shown. Molar percentages are shown as a reference.
The selected cases shown in all show strong crystalline marker peaks. In all 12 cases, crystallinity-indicating peaks for at least one component were detected (data not shown). It can be concluded from the results discussed so far that NaNO3 and KNO3 can indeed crystallize within a few hundred milliseconds (compare ) and that the crystallization kinetics dominate the particle formation process.
Can a drying process that is dominated by crystallization kinetics be controlled, and how do characteristic parameters affect the properties of the final dried microparticles? A key parameter is the time available for crystallization, called the precipitation window, spanning the time from the attainment of supersaturation on the surface to the complete evaporation of solvent. The precipitation window has been shown to be the main parameter controlling crystal size and particle density in a single component system (Baldelli et al. Citation2016). In multi-component systems, each component has a different precipitation window. The particle formation process should be dominated by the component with the longest precipitation window because it may crystallize first. Hence, in , the particle density of the dry particles was plotted as a function of the longer of the two precipitation windows for all tested cases. Longer precipitation windows correlate with lower particle density of the dried microparticles, as predicted. The lower particle density is caused by a larger amount of voids. A long precipitation window indicates that the required time to reach saturation is of short duration. Thus, a shell can be formed earlier on a droplet that still has a large diameter corresponding to the larger diameter of the final dried microparticle. Moreover, a longer precipitation window indicates that the crystals have more time to nucleate and grow to a larger size on the surface, a tendency that should generate more internal voids (). It can be concluded that managing the time to reach saturation, and thus the precipitation window, allows control over the properties of the final dried microparticles, such as their density. The precipitation window can be predicted using an adaptation of Equation (Equation1[1] ). Subtracting the droplet lifetime (Boraey and Vehring Citation2014) yields
Figure 2. Particle density of the final dried microparticles as a function of the precipitation window for the component that first saturates on the surface. The solid lines at the top indicate the true densities of KNO3 and NaNO3.
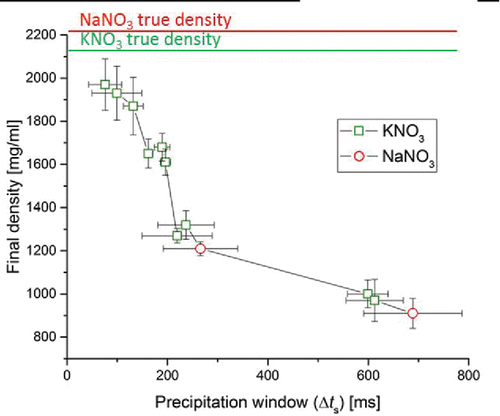
an equation that is useful in discussing which process and formulation parameters must be controlled to affect the precipitation window. Three main factors can be discerned: first, the precipitation window is strongly dependent on the initial droplet diameter, d0, which indicates that control of the atomizer in spray drying of crystalline systems is important. Second, the crystallization window increases with the initial concentration, C0, of a component in relation to its solubility, Csol. The latter can be affected by the total concentration of the formulation and by adjusting the mass fraction of a component in the formulation, but it is also dependent on the temperature of the droplet, which affects the solubility of the components. The droplet temperature and evaporation rate, the third factor, are both dependent on the drying gas temperature. According to this model, none of the listed process and formulation parameters can be varied freely without affecting the crystallization window and with it the morphology of the resulting particles.
From the Raman results, it can be concluded that crystals of either NaNO3 or KNO3 are present in most of the dried microparticles. The particle densities in show that most of these particles are hollow. Therefore, one can conclude that they have a mixed crystalline shell, which is in agreement with the morphology of the sectioned particles shown in .
Is it possible to control the radial distribution of the components in the shell? More specifically, is it possible to design particles with a specific component on the surface of the shell in such a way as to affect, for example, cohesion between particles? Based on the previous discussion, we hypothesize that the parameter that can be used to control the surface composition would be the time to reach saturation (Equation (Equation1[1] )). Specifically, we postulate that a component can be brought to the surface by shortening its time to reach saturation relative to the other component. This hypothesis is illustrated in , which shows the surface concentration for the two solutes as a function of time during the late phase of the evaporation. The cases for 1 mg/ml initial solution concentration at 50°C drying gas temperature and two different molar ratios, 30:70 and 70:30, are plotted. The time to reach saturation for each component is indicated when the solute reaches its solubility. It can be seen that the ratio of KNO3 and NaNO3 affects the sequence of events. The two materials have a different solubility for the same wet bulb temperature; for KNO3 it is easier to reach saturation because of its lower solubility. In the case of the 30:70 molar ratio of NaNO3 to KNO3, the latter is predicted to reach saturation about 90 ms earlier than NaNO3. If the molar ratio is reversed to 70:30, saturation is also reached in a reversed sequence, with NaNO3 now leading by about 20 ms. According to the hypothesis, the latter case should show an increased amount of sodium on the surface.
Figure 3. Surface concentrations of NaNO3 and KNO3 in evaporating droplets as a function of time for the cases with an initial concentration of 1 mg/ml at 50°C drying gas temperature and molar percentages of NaNO3 of 30% or 70% or weight percentages of NaNO3 of 26.5% or 66.2%. ts indicates the time to reach saturation. On the right-hand side, the plot of surface saturation normalized by the solubility of each solute is shown. The time to reach saturation is obtained when the surface concentration equals the solubility. The time for crystallization is reached at a supersaturation level of around 1.6 for NaNO3 and 1.9 for KNO3.
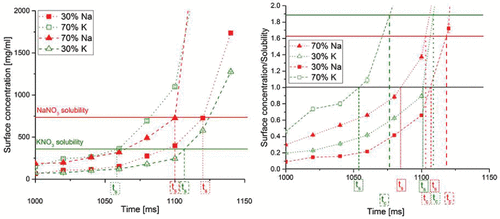
To test the hypothesis that the sequence of the times to reach saturation or crystallization can predict the radial distribution of the chemical components in the final dried microparticles, the times to reach saturation, ts,j, and the times for onset of crystallization, tc,j, were calculated for all 12 cases. To express the relative timing of the saturation and crystallization event for the two components, these times were subtracted from each other according to and
. These time differences, shown in , quantify how much earlier KNO3 reaches saturation and onset of crystallization on the surface than NaNO3. If the values become negative, the sequence is reversed, i.e., NaNO3 reaches these events first. In , the cases are grouped according to their initial conditions, such as total solution concentration, temperature of the drying gas, and molar ratio of NaNO3 and KNO3. It is apparent that KNO3 reaches saturation and onset of crystallization earlier than NaNO3 in almost all cases studied because of the higher solubility of NaNO3. Moreover, NaNO3 nucleates and commences crystal growth only after reaching a molar percentage of 20.7% or a weight percentage of 97% in water at a wet bulb temperature of 18.7°C. As a result, the conditions necessary for NaNO3 to nucleate are of high supersaturation (Tang and Munkelwitz Citation1994). KNO3, by contrast, crystallizes at a molar percentage of 8.3% or weight percentage of 47% at the same temperature. Because of the temperature dependence of the solubilities, an increase of the drying gas temperature and consequently an increase in wet bulb temperature favor the saturation of KNO3 first. Hence, the only cases that allowed NaNO3 to saturate first were the ones with a 70/30 NaNO3/KNO3 molar ratio or 66.2/33.8 NaNO3/KNO3 weight ratio at the lower drying gas temperature.
Table 4. SEM/EDX maps of the chemical components near the surface of the dried particles. Below each map the measured molar ratio of NaNO3 to KNO3 is listed together with the time differences between saturation and crystallization events for the components. Positive values indicate earlier saturation and crystallization for KNO3.
The hypothesis that the sequence in which the components reach saturation or onset of crystallization determines the radial distribution of chemical components in final dried microparticles was verified by comparison with the experimentally determined composition near the surface of the particles. In , the elemental maps from the SEM/EDX analysis for all 12 cases are shown. Green indicates potassium and red indicates sodium. Also listed are the measured molar ratios of the components in the EDX interaction volume near the surface. These results are semi-quantitative due to the difference in overvoltage for the characteristic X-ray peaks in the EDX spectrum.
The only case that has clearly more sodium than potassium on the surface (molar ratio 93/7, second row, right) is also the one with the largest negative values for Δtseq,c and Δtseq,s, i.e., NaNO3 reaches saturation 68 ms earlier and onset of crystallization 86 ms earlier than KNO3. The only other case with negative values for Δtseq,c and Δtseq,s also shows a measured enrichment, albeit smaller, of sodium relative to the nominal ratio. On the other hand, the cases with the highest surface coverage of potassium (molar ratio 14/86, top row left, and molar ratio 12/88, second row, left) also have the largest positive values for Δtseq,c and Δtseq,s. In these cases, KNO3 has a head start of more than 150 ms over NaNO3 for the onset of crystallization. In the other cases, both components reach saturation at about the same time, and the near surface composition does not differ by much from the nominal composition. In summary, the measured near surface composition is in very good agreement with the predictions, providing strong support for the tested hypothesis.
Because SEM/EDX technique provides limited depth resolution, for selected cases the elemental composition of the inner part of the microparticles was further analyzed using S-TEM. shows microtome cuts across the shell of the particles and the elemental composition across the shell as determined by S-TEM. The elemental composition is shown qualitatively as normalized X-ray intensity as a function of position on a scan line across the shell. Peaks shifted more to the left on the x axis (negative position values) are shifted in the direction of the surface. The zero position was chosen arbitrarily in the middle of the shell. An average curve obtained with a Gaussian fit and its center indicated with a vertical line are also shown. The top row of shows the case with surface enrichment of sodium (C0, 10 mg/ml at TGas 50°C for 70% NaNO3 and 30% of KNO3) and as comparison a case with close to nominal EDX composition (C0, 10 mg/ml at TGas of 50°C for 50% NaNO3 and 50% KNO3). The results are in agreement with the SEM/EDX findings. In the case where SEM/EDX measures near surface enrichment of sodium, S-TEM shows a sodium maximum shifted toward the surface relative to the potassium maximum, indicating that sodium crystallized earlier, as predicted. In the other case, both components seem well mixed, indicating that they have crystallized at about the same time.
Table 5. Elemental composition across the shell of sectioned microparticles determined by S-TEM. Peaks shifted to the left represent the chemical components present on the surface. Cases with a solution concentration of 10 mg/ml dried at 50°C for molar percentages of 70% of NaNO3 and 30% of KNO3 and 50% of NaNO3 and 50% of KNO3 are shown.
Conclusions
This study clarifies the particle formation process of a multicomponent droplet with crystallizing solutes. The properties of the dried microparticles can be linked to characteristic times describing the crystallization sub-process, specifically the time at which components reach supersaturation and onset of crystallization and the time available for crystallization. Morphological features of the dried particles, like particle density and radial distribution of components in the shell, can be affected by changing these characteristic times. These particle features can be predicted to some extent, and the influence of process and formulation variables can be evaluated. For example, both the theoretical model and the experimental results indicate that the chemical element to saturate first is predominantly distributed on the surface.
Drugs for pulmonary disease can be combined in structured, spray dried particles that are composed of a crystalline shell encapsulating the drug and excipients. It can be inferred from this study that many formulation and process parameters can affect the particle morphology of crystallizing systems. Among these parameters are atomized droplet diameter, drying gas temperature, feed concentration, and formulation composition. Therefore, these parameters need to be carefully controlled to ensure consistent particle morphology, which may affect the stability and aerosol efficiency of spray-dried particles for inhalation.
The conclusions of this study were drawn from a simple binary system. Further work is necessary to refine the mechanistic understanding of the particle formation process in multicomponent systems with crystallizing and non-crystallizing components.
Acknowledgments
The authors would like to acknowledge Arlene Oatway for her help in sectioning the microparticles and Anqiang He for his support in teaching the use of the S/TEM.
Funding
Funding from the Natural Sciences and Engineering Research Council of Canada (NSERC), Alberta Innovates Technology Futures, and the George Ford Chair endowment is gratefully acknowledged.
References
- Ai, H., Pink, J. J., Shuai, X., Boothman, D. A., and Gao, J. (2005). Interactions Between Self‐Assembled Polyelectrolyte Shells and Tumor Cells. J. Biomed. Mater Res. A, 73:303–312.
- Aman, Z. M., Joshi, S. E., Sloan, E. D., Sum, A. K., and Koh, C. A. (2012). Micromechanical Cohesion Force Measurements to Determine Cyclopentane Hydrate Interfacial Properties. J. Colloid Interf. Sci., 376:283–288.
- Baldelli, A., Boraey, M. A., Nobes, D. S., and Vehring, R. (2015). Analysis of the Particle Formation Process of Structured Microparticles. Mol. Pharmaceut., 12:2562–2573.
- Baldelli, A., Power, R. M., Miles, R. E. H., Reid, J. P., and Vehring, R. (2016). Effect of Crystallization Kinetics on the Properties of Spray Dried Microparticles. Aerosol Sci. Tech., 50:693–704.
- Beck-Broichsitter, M., Dalla-Bona, A. C., Kissel, T., Seeger, W., and Schmehl, T. (2014). Polymer Nanoparticle-Based Controlled Pulmonary Drug Delivery. Drug Delivery System. Vol. 1141. Springer, New York, pp. 133–145.
- Belotti, S., Rossi, A., Colombo, P., Bettini, R., Rekkas, D., Politis, S., and Buttini, F. (2015). Spray-Dried Amikacin Sulphate Powder for Inhalation in Cystic Fibrosis Patients: The Role of Ethanol in Particle Formation. Eur. J. Pharm. Biopharm., 93:165–172.
- Boraey, M. A., Hoe, S., Sharif, H., Miller, D. P., Lechuga-Ballesteros, D., and Vehring, R. (2013). Improvement of the Dispersibility of Spray-Dried Budesonide Powders Using Leucine in an Ethanol–Water Cosolvent System. Powder Technol., 236:171–178.
- Boraey, M. A., and Vehring, R. (2014). Diffusion Controlled Formation of Microparticles. J. Aerosol Sci., 67:131–143.
- Brooker, M. (1978). Raman Spectroscopic Investigations of Structural Aspects of the Different Phases of Lithium Sodium and Potassium Nitrate. J. Phys. Chem. Solids, 39:657–667.
- Clark, A. R. (1991). Metered Atomisation for Respiratory Drug Delivery. PhD Dissertation, Loughborough University, Leicestershire, UK, https://dspace.lboro.ac.uk/2134/7413 © Andrew Reginald Clark.
- De Villiers, S., Lindblom, N., Kalayanov, G., Gordon, S., Malmerfelt, A., Johansson, A., and Svensson, T. (2002). Active Immunization against Nicotine Suppresses Nicotine-Induced Dopamine Release in the Rat Nucleus Accumbens Shell. Respiration, 69:247–253.
- Evans, R., Deng, Z., Rogerson, A. K., McLachlan, A. S., Richards, J. J., Nilsson, M., and Morris, G. A. (2013). Quantitative Interpretation of Diffusion‐Ordered NMR Spectra: Can We Rationalize Small Molecule Diffusion Coefficients?. Angew. Chem. Int. Edit., 52:3199–3202.
- Filková, I., Huang, L. X., and Mujumdar, A. S. (2014). 9 Industrial Spray Drying Systems. Handbook of Industrial Drying. CRC Press, Boca Raton, pp. 191–225.
- Gambill, W. (1959). How to Estimate Mixtures Viscosities. Chem. Eng., 66:151–152.
- Goldstein, J., Newbury, D. E., Echlin, P., Joy, D. C., Romig Jr, A. D., Lyman, C. E., and Lifshin, E. (2012). Scanning Electron Microscopy and X-Ray Microanalysis: A Text for Biologists, Materials Scientists, and Geologists. Springer US, New York.
- Gonzalez, R., Zapatero, L., Caravaca, F., and Carreira, J. (1991), Identification of Soybean Proteins Responsible for Respiratory Allergies. Int. Arch. Allergy Imm., 95:53–57.
- Graber, T., Taboada, M., Alvarez, M., and Schmidt, E. (1999). Determination of Mass Transfer Coefficients for Crystal Growth of Nitrate Salts. Cryst. Res. Technol., 34:1269–1277.
- Hakuta, Y., Hayashi, H., and Arai, K. (2003). Fine Particle Formation Using Supercritical Fluids. Curr. Opin. Solid S M., 7:341–351.
- Hoe, S., Ivey, J. W., Boraey, M. A., Shamsaddini-Shahrbabak, A., Javaheri, E., Matinkhoo, S., and Vehring, R. (2014). Use of a Fundamental Approach to Spray-Drying Formulation Design to Facilitate the Development of Multi-Component Dry Powder Aerosols for Respiratory Drug Delivery. Pharm. Res., 31:449–465.
- Holden, A., and Morrison, P. (1982). Crystals and Crystal Growing. MIT Press, Cambridge, MA.
- Islan, G., Cacicedo, M., Bosio, V., and Castro, G. (2015). Development and Characterization of New Enzymatic Modified Hybrid Calcium Carbonate Microparticles to Obtain Nano-Architectured Surfaces for Enhanced Drug Loading. J. Colloid Interf. Sci., 439:76–87.
- Isono, T. (1984). Density, Viscosity, and Electrolytic Conductivity of Concentrated Aqueous Electrolyte Solutions at Several Temperatures. Alkaline-Earth Chlorides, Lanthanum Chloride, Sodium Chloride, Sodium Nitrate, Sodium Bromide, Potassium Nitrate, Potassium Bromide, and Cadmium Nitrate. J. Chem. Eng. Data, 29:45–52.
- Ko, J., Park, H., Hwang, S., Park, J., and Lee, J. (2002). Preparation and Characterization of Chitosan Microparticles Intended for Controlled Drug Delivery. Int. J. Pharm., 249:165–174.
- Kracek, F., Posnjak, E., and Hendricks, S. (1931). Gradual Transition in Sodium Nitrate. II. The Structure at Various Temperatures and Its Bearing on Molecular Rotation. J. Am. Chem. Soc., 53:3339–3348.
- Laliberté, M. (2007). Model for Calculating the Viscosity of Aqueous Solutions. J. Chem. Eng. Data, 52:321–335.
- Lechuga-Ballesteros, D., and Miller, D. P. (2015). Advances in Respiratory and Nasal Drug Delivery. Mol. Pharm., 12:2561–2561.
- Longest, P. W., Spence, B. M., Holbrook, L. T., Mossi, K. M., Son, Y.-J., and Hindle, M. (2012). Production of Inhalable Submicrometer Aerosols from Conventional Mesh Nebulizers for Improved Respiratory Drug Delivery. J. Aerosol Sci., 51:66–80.
- Mahiuddin, S., and Ismail, K. (1996). Temperature and Concentration Dependence of the Viscocity of Aqueous Sodium Nitrate and Sodium Thiosulphate Electrolytic Systems. Fluid Phase Equilib., 123:231–243.
- Niwa, T., Mizutani, D., and Danjo, K. (2012). Spray Freeze-Dried Porous Microparticles of a Poorly Water-Soluble Drug for Respiratory Delivery. Chem. Pharmaceut. Bull., 60:870–876.
- Oriakhi, C. O. (2009). Chemistry in Quantitative Language: Fundamentals of General Chemistry Calculations. Oxford University Press, USA.
- Pasquali, I., Bettini, R., and Giordano, F. (2006). Solid-State Chemistry and Particle Engineering with Supercritical Fluids in Pharmaceutics. Eur. J. Pharm. Sci., 27:299–310.
- Patravale, V., and Kulkarni, R. (2004). Nanosuspensions: A Promising Drug Delivery Strategy. J. Pharm. Pharmacol., 56:827–840.
- Pechkova, E., Peter, W., Bragazzi, N., Fernanda, F., and Nicolini, C. (2015). Nanoprobe Nappa Arrays for the Nanoconductimetric Analysis of Ultra-Low-Volume Protein Samples Using Piezoelectric Liquid Dispensing Technology. NanoWorld J., 1:26–31.
- Pilcer, G., and Amighi, K. (2010). Formulation Strategy and Use of Excipients in Pulmonary Drug Delivery. Int. J. Pharm., 392:1–19.
- Ragab, D. M., and Rohani, S. (2009). Particle Engineering Strategies Via Crystallization for Pulmonary Drug Delivery. Org. Process Res. Dev., 13:1215–1223.
- Robinson, J. W. (1991). Practical Handbook of Spectroscopy. CRC Press, Boca Raton, FL.
- Sharma, S. K. (2008). Atomic and Nuclear Physics. Pearson Education, India.
- Shekunov, B. Y., and York, P. (2000). Crystallization Processes in Pharmaceutical Technology and Drug Delivery Design. J. Cryst. Growth, 211:122–136.
- Shen, T., Mitra, S., Prask, H., and Trevino, S. (1975). Order-Disorder Phenomenon in Sodium Nitrate Studied by Low-Frequency Raman Scattering. Phys. Rev. B, 12:4530–4533.
- Shoyele, S. A., and Cawthorne, S. (2006). Particle Engineering Techniques for Inhaled Biopharmaceuticals. Adv. Drug Deliv. Rev., 58:1009–1029.
- Slominski, C. G., Kraynik, A. M., and Brady, J. F. (2014). The Einstein Shear Viscosity Correction for Non No-Slip Hyperspheres. J. Colloid Interf. Sci., 430:302–304.
- Sung, J. C., Pulliam, B. L., and Edwards, D. A. (2007). Nanoparticles for Drug Delivery to the Lungs. Trends Biotechnol., 25:563–570.
- Tang, I., and Munkelwitz, H. (1994). Water Activities, Densities, and Refractive Indices of Aqueous Sulfates and Sodium Nitrate Droplets of Atmospheric Importance. J. Geophys. Res. Atmos. (1984–2012), 99:18801–18808.
- Vehring, R., Foss, W. R., and Lechuga-Ballesteros, D. (2007). Particle Formation in Spray Drying. J. Aerosol Sci., 38:728–746.
- Vicente, J., Pinto, J., Menezes, J., and Gaspar, F. (2013). Fundamental Analysis of Particle Formation in Spray Drying. Powder Technol., 247:1–7.
- Vladisavljević, G. T., Shahmohamadi, H., Das, D. B., Ekanem, E. E., Tauanov, Z., and Sharma, L. (2014). Glass Capillary Microfluidics for Production of Monodispersed Poly (Dl-Lactic Acid) and Polycaprolactone Microparticles: Experiments and Numerical Simulations. J. Colloid Interf. Sci., 418:163–170.
- Wang, H., Boraey, M. A., Williams, L., Lechuga-Ballesteros, D., and Vehring, R. (2014). Low-Frequency Shift Dispersive Raman Spectroscopy for the Analysis of Respirable Dosage Forms. Int. J. Pharm., 469:197–205.
- Young, P. M., Salama, R. O., Zhu, B., Phillips, G., Crapper, J., Chan, H.-K., and Traini, D. (2015). Multi-Breath Dry Powder Inhaler for Delivery of Cohesive Powders in the Treatment of Bronchiectasis. Drug Dev. Ind. Pharm., 41:859–865.
- Zhang, J., Wu, L., Chan, H.-K., and Watanabe, W. (2011). Formation, Characterization, and Fate of Inhaled Drug Nanoparticles. Adv. Drug Deliv. Rev., 63:441–455.
- Zhang, Q.-N., Zhang, Y., Cai, C., Guo, Y.-C., Reid, J. P., and Zhang, Y.-H. (2014). In Situ Observation on the Dynamic Process of Evaporation and Crystallization of Sodium Nitrate Droplets on a ZnSe Substrate by FTIR-ATR. J. Phys. Chem. A, 118:2728–2737.
- Zhang, Y., and Stangle, G. C. (1994). Preparation of Fine Multicomponent Oxide Ceramic Powder by a Combustion Synthesis Process. J. Mater. Res., 9:1997–2004.
- Zhou, Q. T., Leung, S. S. Y., Tang, P., Parumasivam, T., Loh, Z. H., and Chan, H.-K. (2015). Inhaled Formulations and Pulmonary Drug Delivery Systems for Respiratory Infections. Adv. Drug Deliv. Rev., 85:83–99.
- Zhu, J., and Hayward, R. C. (2012). Interfacial Tension of Evaporating Emulsion Droplets Containing Amphiphilic Block Copolymers: Effects of Solvent and Polymer Composition. J. Colloid Interf. Sci., 365:275–279.