ABSTRACT
The effective density ρeff of particles emitted from various types of automobile engines was measured using a differential mobility analyzer (DMA)–aerosol particle mass analyzer method, and their morphology was investigated via transmission electron microscopy analysis. The measured exhaust particles were particles emitted from diesel engines (DEs), gasoline direct injection spark ignition (DISI) engines, gasoline port fuel injection (PFI) engines, and liquefied petroleum gas (LPG) engines. ρeff and the morphology of the particles were measured after classification with the DMA, and six electrical mobility diameters Dm ranging from 30 to 300 nm were selected. ρeff was found to decrease as Dm increased for all particles. A morphological study showed that DE and DISI particles were mainly agglomerates and PFI and LPG particles were mainly nonagglomerates. Numbers and diameters of the primary particles in the agglomerates showed no systematic differences between DE and DISI particles at a given Dm. Rather, the primary particle diameter dp increased with increasing Dm of the agglomerates; the empirical relationship between the two diameters was found to be dp = 8.498ln(Dm) – 12.781 for DE and DISI particles. The core (elemental carbon) diameters in the primary particles of the DE particles increased as Dm increased and were estimated to range from 8.5 nm for Dm = 70 nm to 22.1 nm for Dm = 300 nm. Although the primary particle diameter and core diameter depend on Dm, the organic coating (shell) thickness, which ranged from 5.1 to 7.4 nm, was found to be independent of Dm.
Copyright © 2016 American Association for Aerosol Research
EDITOR:
1. Introduction
Diesel exhaust particles (DEPs) were once major contributors to the environmental burden of urban aerosols. However, because of the tightening of emission regulations and improvements in emission reduction technology, such as the addition of after-treatment devices, the amount of emitted DEPs has decreased in recent years. Thus, as diesel exhaust has become cleaner, the consideration of emissions from other fueled vehicles has increasingly gained importance.
As can be seen from the fact the emission of particulate matter from gasoline vehicles is not regulated by emission control in Japan, gasoline port fuel injection (PFI) vehicles emit much less particulate matter than diesel vehicles do. However, recent reports show that gasoline direct injection spark ignition (DISI) vehicles, which run at a stoichiometric air–fuel ratio, do emit particulate matter (Farron et al. Citation2011; Maricq et al. Citation2012); moreover, DISI vehicles emit much more particulate matter than PFI vehicles (Barone et al. Citation2012; Kobayashi et al. Citation2012; Fushimi et al. Citation2016). The number of DISI vehicles has been increasing because DISI vehicles have lower fuel consumption and emit less CO2. Furthermore, liquefied petroleum gas (LPG) vehicles have the third longest travel distance among the types of vehicles available in Japan. In Japan, 94% of the taxi fleet, which comprises a total of 260,000 vehicles, operates on LPG fuel (Ristovski et al. Citation2005). LPG is a possible alternative fuel because of its potential for reducing emissions, relatively low fuel price, and low CO2 emissions (Myung et al. Citation2012).
Traditionally, diesel engines emit increasingly amounts of soot particles at a high air-to-fuel ratio and a combustion chamber temperature of approximately 1800–2500 K. The size of soot particles is typically in the sub-micron range. This particle is a fractal-like agglomerate of primary particles of 15–30 nm in diameter composed of elemental carbon and traces of metallic ash and coated with condensed organic compounds and sulfate (Maricq Citation2007; Fujitani et al. Citation2012a). In contrast, nucleation-mode particles are smaller than typical soot particles. Nucleation-mode particles and soot particles are generated by different mechanisms and have different chemical compositions. The factors that affect the generation of nucleation-mode particles from diesel engines are the engine operating conditions, the sulfur content in the fuel and lubricating oil, the dilution ratio of clean air, the temperature and relative humidity of the air, and after-treatment devices. Organic compounds derived from lubricating oil are dominant in nucleation-mode particles and contain less elemental carbon (Fushimi et al. Citation2011).
It is not likely for the richness of the mixture in gasoline engines to be a major cause of soot production; instead, soot production in gasoline engines is likely caused primarily by the diffusion combustion of the fuel droplets that have not vaporized before combustion begins, the diffusion combustion of residual gasoline that adheres to the piston or cylinder walls in its liquid state, or a combination of the two (Kayes and Hochgreb Citation1999; Aikawa and Jetter Citation2014). In DISI engines, the size distribution of exhaust particles has been observed to possess two modes. The larger mode, named the accumulation mode, shows a mean diameter of 45–60 nm and is composed primarily of carbonaceous agglomerates and adsorbed material (Pirjola et al. Citation2015). The second, smaller mode, named the nucleation mode, often has a maximum mean diameter of 20 nm. Sgro et al. Citation(2012) reported that these very small particles form in the combustion chamber and are composed of nonvolatile cores of 1–5 nm in diameter mainly consisting of amorphous carbon; they also stated that semivolatile species condense on the surfaces of these particles as the exhaust cools in the tail pipe. Furthermore, the major organic components for both the accumulation- and nucleation-mode particles are C20–C28 hydrocarbons. The contribution of engine oil was estimated to be 10–30% for organics and the sum of the measured elements. The majority of the remaining fraction likely originates from gasoline fuel (Fushimi et al. Citation2016).
There have been few emission studies of LPG vehicles in comparison with diesel and gasoline vehicles. The fuel used in LPG vehicles has been found to be cleaner; however, in most cases, the differences between the particle number and mass emission factors of LPG and gasoline vehicles have not been found to be statistically significant because of the large variation in emissions among different vehicles (Ristovski et al. Citation2005). The emitted particles may be unburned micro-fuel droplets generated as a result of a relatively short homogeneous fuel–air mixture duration in the engine intake manifold, which was clarified by comparing liquid and gaseous phase LPG injection systems; liquid phase injection systems were found to produce relatively large particles at high concentrations (Lee et al. Citation2010). More recently, Myung et al. (Citation2012) investigated emissions from direct injection LPG vehicles, and a sub-30-nm nuclei mode was mainly found to form. However, they observed that the LPG vehicles reduced particle number emissions by two orders of magnitude in comparison with DISI vehicles. Further, as demonstrated in the summary by Momenimovahed et al. (Citation2013) of data obtained in previous studies and in their study, in comparison with LPG vehicles, PFI vehicles produce 4.6 times the number of particles with 2.1 times the mass.
Studies investigating the particle number and mass emission factors and attempting to characterize chemical species of particles from automobiles have been conducted largely from the perspective of their impact on environmental air quality and public health. In contrast, there have been fewer studies on the physical properties of automobile exhaust particles, such as their particle morphology (shape) and particle density. However, studying these physical properties is also important because they affect particle transport phenomena, such as dry deposition, cloud scavenging, non-isokinetic sampling artifacts, and lung deposition (Schmid et al. Citation2007), and are needed to convert among various equivalent particle sizes (e.g., aerodynamic diameter and electrical mobility diameter). Furthermore, particle shape determines the toxicity of inhaled particles; for example, the fiber length and aspect (length-to-width) ratio are important toxicity parameters for fiber particles (Stanton et al. Citation1981; Poland et al. Citation2008). Inhaled fiber particles may become embedded deep in the lungs, regardless of their length, and are easily deposited by the interception specific to airborne particles with fiber-like structures (Hinds Citation1999).
Structures related to the primary particle, such as the number of primary particles and their size, core diameter, and shell thickness, can affect the degree of absorption of solar radiation, especially for agglomerates. In this study, the term “primary particle” indicates one sphere of an agglomerate. Black carbon, which is a light-absorbing carbon, acts as a core and is covered by organic compounds and other species. The concentric-shell-and-core model predicts an increase in absorption above that of a pure absorbing particle by many calculations; that is, depositing a shell of non-absorbing material around a pure light absorbing carbon particle increases the absorption of the pure particle (Bond et al. Citation2006). The structural characteristics of agglomerates, such as their surface area, are also related to the toxicity of inhaled particles (Oberdorster Citation2000; Donaldson et al. Citation2001; Wittmaack Citation2007; Donaldson et al. Citation2008; Sager and Castranova Citation2009), and the agglomerate surface area increases as more particles agglomerate for a given equivalent size (e.g., aerodynamic size).
Although concurrent morphological studies and measurements of the effective density have been intensively conducted on DEPs (e.g., Park et al. Citation2003, Citation2004; Virtanen et al. Citation2004), there have been fewer comprehensive studies on particles emitted from DISI, PFI, and LPG engines. Morphological studies on DISI particles have been conducted by Mathis et al. Citation(2004), Barone et al. Citation(2012), and Dastanpour and Rogak (Citation2014); however, there have been no systematic experiments investigating equivalent size. Thus, it is difficult to elucidate the correlation between equivalent size and the physical size observed in transmission electron microscopy (TEM) images. Maricq and Xu (Citation2004) and Momenimovahed and Olfert (Citation2015) reported the effective density of DISI particles, and Quiros et al. (Citation2015) reported those of two GDI and two PFI vehicles as functions of the electrical mobility diameter, but their study contained no morphology data. No such data are available for particles emitted from LPG vehicles.
Agglomerates are assumed to be produced from equal-sized primary particles regardless of the size of the agglomerates, and this assumption has been used extensively in the generation of numerical agglomerates and the estimation of soot properties (Dastanpour and Rogak Citation2014). However, recent studies showed that the primary particle size changes with the agglomerate size (Barone et al. Citation2012; Lee et al. Citation2013; Seong et al. Citation2013, Citation2014; Dastanpour and Rogak Citation2014). Although the agglomerate size and primary particle size were discussed, the relationship between the mobility size and primary particles size has not yet been clarified for samples after classified by mobility size.
Therefore, in this study, the single particle mass and effective density of DEPs and other particles emitted from DISI, PFI, and LPG engines were measured with a differential mobility analyzer (DMA) and an aerosol particle mass analyzer (APM). In addition, a particle morphology study was conducted using a TEM to take images of the particles. The primary particle size in the agglomerates was measured, and the core diameter and shell thickness of the primary particle were estimated as a function of the electrical mobility diameter.
2. Materials and methods
2.1. Test vehicles and engine
lists the characteristics of the test vehicles and engine. An eight-liter diesel engine, located in the Nanoparticle Health Effect Laboratory of the National Institute for Environmental Studies (Fujitani et al. Citation2009, Citation2012b), was used to sample diesel exhaust particles. The engine was operated in two steady states: high idle state (2000 rpm and 0 Nm) and high torque state (1000 rpm and 300 Nm). These operations were selected because the ratios of elemental carbon to total carbon during these operations were both ends of the ratios in this engine for various engine speeds and torques. The bulk samples of the ratios for high idle and high torque states were 0.24 and 0.85, respectively (Fujitani et al. Citation2012a, Citationb). Although these operating conditions are not relevant to those used in real-world scenarios, the particle properties of most practical operating conditions, including real-world transition operating conditions, are within this range. Exhausts were introduced into a primary dilution tunnel and diluted with particle-free clean air at a flow rate of 100 m3/min to obtain dilution ratios of 16 and 28 in the high idle and high torque states, respectively. Other test vehicles were studied using a chassis dynamometer system at the National Institute of Environmental Studies (Nakashima et al. Citation2010). Samples were drawn from a full-flow dilution tunnel at 5–43 m3/min, and exhaust was diluted to ratios of 15–57 depending on the test vehicle. The tunnel background was also measured at a dilution flow of 5 m3/min. All vehicles were operated under steady state conditions from 20 to 80 km/h. The fuels and lubricating oils used in this study are available and commonly used in Japan. Low-sulfur content (6 ppm) diesel fuel and regular gasoline were used. The composition of the LPG liquid fuel used in the LPG A engine was 59.8% n-butane, 29.7% isobutene, and 10.2% propane, and the composition of the LPG gaseous fuel used in the LPG B engine was 66.8% isobutene, 22.8% n-butane, and 10.0% propane. Genuine lubricating oils were used in all test vehicles and the test engine.
Table 1. List of engines and vehicles used in this study.
2.2. Test of polystyrene latex particles
Monodisperse polystyrene latex (PSL) particles (STADEX series, JSR Corp., Tokyo, Japan) were measured in the same manner as the exhaust from the automobiles because these particles have a simple spherical shape, a standardized size, and a known material density of nearly unity, which makes them easy to use to check the measurement system. The PSL particles were aerosolized in the same manner as in a study by Fujitani et al. (Citation2015).
2.3. Instrumentation
The effective densities and particle morphologies were characterized according to the equivalent particle size (electrical mobility diameter) rather than the physical particle size because the equivalent particle size provides more meaningful information about airborne particles. lists the experimental combinations for each engine. The effective density was measured at least twice for each combination. Figure S1 shows a block diagram of the experimental system. From polydisperse particles in diluted exhaust, monodisperse particles with electrical mobility diameters Dm of 30, 50, 70, 100, 200, and 300 nm were classified by a DMA (Sibata Scientific Technology, Soka, Saitama, Japan) with a sheath-to-sample flow rate ratio of 10:1. The sample flow rate was set to 2.4 or 3.0 L/min.
Table 2. Combinations of experimental particle sizes and engine operations considered in this study.
An APM (Model 3600, Kanomax, Osaka, Japan) was used to determine the single particle mass m and particle effective density ρeff, which is calculated as[1]
Two condensation particle counters (CPC; CPC1: Model 3025A, CPC2: Model 3772, TSI, Inc., Shoreview, MN, USA) were used to measure the numbers of particles before and after they passed through the APM, respectively, and the particle number ratio was defined as the ratio of the particle number concentration measured by CPC2 to that measured by CPC1. The APM was operated at a variable voltage and a constant rotation. The rotation, which characterizes the classification performance, was set to λ = 0.22–0.40 (Ehara et al. Citation1996). The value of the rotation was checked with a pulse meter (Model 73402, Yokogawa Electric Corporation, Tokyo, Japan), and the voltage was monitored with a voltmeter (Fluke, Model 8846a, Everett, WA, USA). The particle mass was calculated from the voltage at the maximum particle number ratio. This means that the determined particle mass is the mass of highest population of particle type if there are some types (e.g., agglomerates, non-agglomerates) contained at the given particle size. Two scanning mobility particle sizers (SMPSs; Models 3034 and 3936, TSI, Inc.) were used to periodically check the size distribution of the particles before and after they passed through the DMA. After passing through the DMA, the air flow was distributed between the two instruments using a flow splitter (Model 3708, TSI, Inc.). Electrically conductive tubing and metal connectors were used in this experimental system.
The monodisperse particles were collected by an electrical precipitator (Sibata) for TEM observation. The electrical precipitator was operated at 9 kV, and charged particles were collected evenly on 200-mesh collodion membrane-coated copper grids (Nisshin EM, Tokyo, Japan) after passing through the DMA. The particle morphology was observed with a transmission electron microscope (JEM-2010, JEOL, Akishima, Japan). Particle images were taken at a magnification of 200,000 to yield a single particle per image. Images were processed with Adobe Photoshop (ver. 12.04). Between 20 and 50 images from each sample were analyzed. The numbers of primary particles per agglomerate were derived using a previously published method (Brasil et al. Citation1999). The three-dimensional (3D) morphological properties of the agglomerates were derived from the two-dimensional (2D) image. The primary particle diameter dp and overlap segment OV were obtained from the original image, and the 2D overlap coefficient COV, projected = OV/dp was determined from these two parameters. The original images were then converted to shaded images, and the projected area Aa of the agglomerate was determined. The number of primary particles Np in the agglomerate was derived using the 3D COV (derived from COV, projected), Aa, and the cross-sectional area of the primary particle ( = πdp2/4). In addition, the surface area of the agglomerate was derived using the 3D COV, Np, and dp.
For high idle operation in the Diesel A test, size-resolved particles were collected with NanoMOUDI-II cascade impactors (Model 125B, MSP, Shoreview, MN, USA). The configuration comprised thirteen stages with 50% cutoff sizes of 10000, 5600, 3200, 1800, 1000, 560, 320, 180, 100, 56, 32, 18, and 10 nm in aerodynamic size. Two impactors were used to simultaneously sample different substrates. Gold foil (Mitsubishi Materials, Tokyo, Japan; ø47 mm, 17 μm thickness) and polycarbonate filters (Nuclepore; ø47 mm, 0.05 μm pore size) were used as impaction substrates. The gold foil was analyzed for particle mass and carbon components, and the polycarbonate filters were analyzed for elements and inorganic ions in accordance with the method by Fushimi et al. (Citation2011). From these data, the mass of organic compounds (Org) was determined by subtracting the mass of elemental carbon (EC) and inorganic species from the particle mass. The aerodynamic diameter Dae can be converted to Dm as follows (Kasper Citation1982; Park et al. Citation2003):[2] where ρ0 is unit density (1 g/cm3) and Cae and Cm are slip correction factors. Then, the EC/Org mass ratio for each Dm was used to derive the properties of the agglomerates.
3. Results and discussion
Further analysis was not conducted for particles emitted from the Diesel C and LPG B engines, because emissions from these engines were extremely low. For these engines, the particle number concentrations of particles with Dm = 100 nm after passing through the DMA were low (0.059 and 0.148 cm−3 for Diesel C and LPG B, respectively) and were comparable to those of the dilution tunnel background (0.099 cm−3). For LPG engines, the emission rate of the gas phase-supplied fuel type is lower than that of the liquid phase-supplied fuel type; this result was also obtained by Lee et al. (Citation2010). Concentrations obtained during tests of other test engines were at least five times higher than those in the dilution tunnel background. TEM results of Diesel A for Dm = 30–200 nm under both engine operating conditions have also been reported by Fujitani et al. (Citation2012a) and are included in this article for comparison. In TEM analysis, there is some concern about the inclusion of multiply charged large particles. This issue was discussed by Fujitani et al. (Citation2012a) and is described in the Supplementary Material of this article.
3.1. Effective density
The measured effective densities ρeff of the PSL particles were lower than the reference values by manufacturer by approximately 5% for all particle sizes mainly because of diffusion loss inside the APM (Lin et al. Citation2014), especially for smaller particle; this result was used to correct all exhaust particles. shows the corrected ρeff values for the exhaust particles and the raw results for the PSL particles. The ρeff values for the PSL particles were almost constant near unity for all particle sizes. The PSL particles are single spherical particles and contain no voids; thus, the values of ρeff were equal to the material densities. Conversely, as reported in previous studies, ρeff values for exhaust particles decreased with increasing Dm, which depends on the material density and morphology (degree of voids) of the particles. At the given size, the differences in ρeff within each fueled engine were larger than those among different fueled engines, and there were no systematic differences between ρeff values of engines using different fuels.
Figure 1. Effective densities of particles. The results of the PSL particles are shown as raw data, and others were corrected using the PSL results. Values in parentheses are mass–mobility exponents calculated using Equation (Equation3[3] ). Error bars indicate the standard deviations of multiple measurements.
![Figure 1. Effective densities of particles. The results of the PSL particles are shown as raw data, and others were corrected using the PSL results. Values in parentheses are mass–mobility exponents calculated using Equation (Equation3[3] ). Error bars indicate the standard deviations of multiple measurements.](/cms/asset/a28f387d-c18c-41cb-b688-461a03715e75/uast_a_1218438_f0001_oc.gif)
For 100-nm diesel particles, previous studies (Virtanen et al. Citation2002; Park et al. Citation2003; Maricq and Xu Citation2004; Olfert et al. Citation2007) have reported that ρeff values ranged from approximately 0.5 to 1.1, whereas the range obtained in the present study was 0.61–0.76. Previous studies showed that the sulfur content of the fuel affected ρeff values, and higher effective densities were observed (Park et al. Citation2003; Olfert et al. Citation2007). Diesel oxidation catalysts (DOCs) favor this situation, and it was found that at high loads, DOCs increase in temperature and sulphate levels would then increase (Olfert et al. Citation2007). These studies used sulfur contents of <50 to 430 ppm. In this study, ultralow-sulfur content fuel was used, and the sulfate content of the particles was also quite low. Therefore, the presently reported ρeff values may be lower than those obtained in previous studies. Park et al. (Citation2003) obtained ρeff = 0.59 for Dm = 107 nm using fuel containing no sulfur; the present data was comparable to their data. Furthermore, vehicles with and without DOCs can be compared using ultralow-sulfur content fuel. In this case, ρeff for the 100-nm diesel particle was slightly smaller in the DOC vehicle, which is partially due to the removal of hydrocarbons that would have condensed into particles.
For 100-nm DISI particles (adjust using size dependence of ρeff), previous studies (Maricq and Xu Citation2004; Momenimovahed and Olfert Citation2015; Quiros et al. Citation2015) reported that ρeff ranged from 0.38 to 0.61. The present ρeff results ranging from 0.58 to 0.81 were relatively high compared with this previously reported range. For 100-nm PFI particles, relatively high ρeff values (0.79–0.82) compared with other exhaust particles considered in this study were measured. These ρeff values were also higher than the ρeff values (0.42–0.52 for 100-nm PFI particles adjust using size dependence of ρeff) reported by Quiros et al. (Citation2015). Due to comparable data is small, data of ρeff values, including image data, must be accumulated for various operating conditions and types of PFI vehicles. This article is the first to report ρeff values of LPG particles. The ρeff values obtained in this study were 1.19 for 30 nm and 0.64 for 100 nm, indicating a large variation in ρeff with particle size.
The fractural-like dimension (Park et al. Citation2003), recently commonly called the mass–mobility exponent Dfm (Sorensen Citation2011), is given by[3]
where C is a prefactor. The calculation results are shown in . For PSL particles, Dfm was 2.98, which indicates a compact shape. The engine exhaust particles were found to have Dfm values ranging from 2.49 to 2.79. Previously reported Dfm values of DEP range from 2.20 to 2.97 (Park et al. Citation2003; Maricq and Xu Citation2004; Olfert et al. Citation2007; Nakao et al. Citation2011). The Dfm values of DISI and PFI particles have been reported to be 2.3–2.56 (Maricq and Xu Citation2004; Momenimovahed and Olfert Citation2015; Quiros et al. Citation2015) and 2.67 (Quiros et al. Citation2015), respectively. Most Dfm values measured in this study were consistent with the ranges obtained in previous studies but were found to be in the upper limit of these ranges, whereas relatively low values were obtained for LPG particles.
3.2. Particle morphology
Using the TEM images obtained in this study, particles were classified based on particle morphology as agglomerates, non-agglomerates, and other shapes, including bar- and mat-like particles. In addition, particles were classified based on electron opacity and electron transparency to qualitatively describe the different materials that comprise the particles, as observed in the TEM images (Okada and Heintzenberg Citation2003; Barone and Zhu Citation2008). shows the particle morphology distribution for each Dm.
Figure 2. Numbers of typical particle types as fractions of the total number of particles at various electrical mobility diameters. (a) Diesel A (high idle), (b) Diesel A (high torque), (c) Diesel B 60 km/h, (d) DISI A 20 km/h, (e) DISI A 60 km/h, (f) DISI B 60 km/h, (g) PFI A 80 km/h, (h) PFI B 80 km/h, (i) LPG A 60 km/h. N.M.: Not measured.
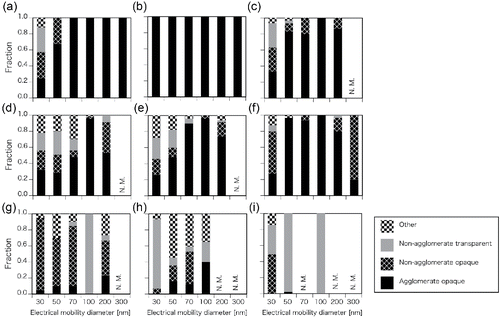
show TEM images of the major types of particles for Dm = 30 and 100 nm, respectively. TEM images of other particle types are provided in the Supplementary Material (Figures S3–S6). In the smaller particle size range, the particle type was typically variable, but opaque agglomerate particles were dominant in the DEPs and DISI particles over the entire size range. These agglomerates are soot particles and agglomerates consisting of tiny primary particles. Conversely, particles from PFI A, PFI B, and LPG A were predominantly non-agglomerates over the entire size range; this is likely the signature of mist particles. There are many tiny electron-dense spots in LPG A particles (), and silicone was detected by energy dispersive X-ray analysis. The source of this silicone was unclear but was likely deriving from lubricating oil, from particles contained in intake air for engine combustion, or from mechanical parts in the engine or the parts connecting the exhaust pipe and dilution tunnel. However, the former of two possibilities are not likely because the estimated emission rate of these two sources does not accurately reflect the actual emission rate of silicone in the exhaust obtained by bulk chemical analysis of filter sample.
Figure 3. TEM images of typical particles with electrical mobility diameters Dm of 30 nm. (a) PSL particle, (b-1) Diesel A (high idle): Agglo, (b-2) Diesel A (high idle): Non-agglo-tra, (c) Diesel A (high torque): Agglo, (d) Diesel B 60 km/h: Agglo, (e) DISI A 20 km/h: Agglo, (f) DISI A 60 km/h: Agglo, (g-1) DISI B 60 km/h: Agglo, (g-2) DISI B 60 km/h: Non-agglo-opa, (h) PFI A 80 km/h: Non-agglo-opa, (i) PFI B 80 km/h: Non-agglo-tra, (j) LPG A 60 km/h: Non-agglo-opa. Scale bars with a length of 100 nm are included for reference. Agglo: Agglomerate electron-opaque particle, Non-agglo-opa: Non-agglomerate electron-opaque particle, and Non-agglo-tra: Non-agglomerate electron-transparent particle.
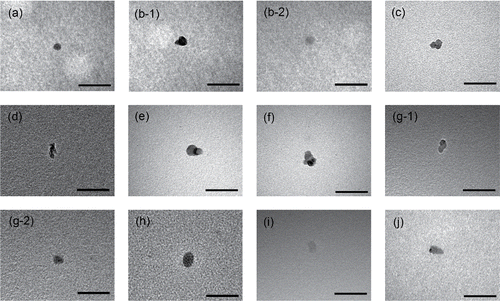
Figure 4. TEM images of typical particles with electrical mobility diameters Dm of 100 nm. (a) PSL particle, (b) Diesel A (high idle): Agglo, (c) Diesel A (high torque): Agglo, (d) Diesel B 60 km/h: Agglo, (e) DISI A 20 km/h: Agglo, (f) DISI A 60 km/h: Agglo, (g) DISI B 60km/h: Agglo, (h) PFI A 80 km/h: Non-agglomerate-tra, (i) PFI B 80 km/h: Non-agglomerate-tra, (j) LPG A 60 km/h: Non-agglomerate-tra. Scale bars with a length of 100 nm are included for reference. Agglo: Agglomerate electron-opaque particle, and Non-agglomerate-tra: Non-agglomerate electron-transparent particle.
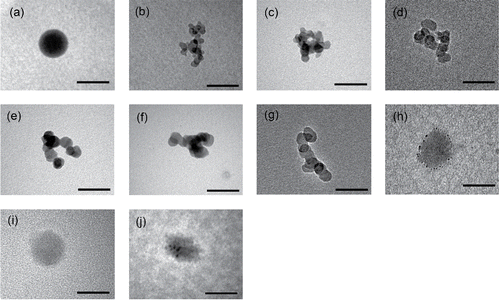
3.3. Physical properties of non-agglomerates
Non-agglomerates and agglomerates are discussed separately. PFI and LPG particles mainly consist of non-agglomerates and are shown to have compact shapes for all sizes in the TEM images. A round shape in a TEM image is a signature of spherical airborne particles, which are likely mainly generated by condensation during the cooling process after exiting the combustion chamber in the absence of soot particles. Non-agglomerates are speculated to consist mainly of organic compounds and little EC, and organic compounds are derived from unburned fuel and lubricating oil. Therefore, a particle generation mechanism other than the soot generation mechanism is important for PFI and LPG engines in steady state operation. Soot particles are organic vapor sinks via absorption and adsorption; therefore, the absence of soot particles promotes the condensation of organic compounds, thus forming spherical particles.
Dfm in Equation (Equation3[3] ) depends on the effective density, which in turn depends on not only the particle morphology but also the material density. Non-agglomerates are compactly shaped, and it is expected that they contain no voids; thus, their effective densities are directly impacted by the material density. If the material density were constant for all particle sizes, Dfm would be near 3.0. However, the Dfm values of these particles were lower than 3.0, and that of the LPG particles was especially low (2.49). The relatively low Dfm values of these particles were the result of increasing material densities with decreasing particle size. This in turn was due to enriched lubricating additives, such as metal components, which may act as nucleation cores.
Previous studies show that heterogeneous nucleation occurs in diesel exhausts. The volatility measurement indicates that nucleation-mode particles contain a nonvolatile core (Ronkko et al. Citation2007). Miller et al. (Citation2007) studied the role of organic compounds and metals from lubrication oil on particle formation using a vehicle whose diesel engine was driven with hydrogen gas. No soot particles were emitted from this vehicle because no organics were contained in the fuel. Their study suggested that less volatile elements, such as iron and calcium cores, were coated by higher-volatility species around them. These cores were generated after the initial combustion and during piston retraction via vaporized elements and underwent nucleation. The metals were derived from additives in lubricating oil and mechanical abrasion in the engine (Miller et al. Citation2007). This generation mechanism is also applicable to PFI and LPG vehicles because lubricating oil is used in these vehicles.
At Dm = 30 nm in Diesel A (High idle), non-agglomerate particles were found to be dominant by TEM measurement () and have the lowest ρeff (0.75 g/cm3 in ) among the particles of the different engines at this Dm. The ρeff value was close to the liquid density of diesel fuel (0.81 g/cm3) or lubricating oil (0.87 g/cm3). The EC/Org ratio from the chemical speciation of the MOUDI sample also indicated that EC was a minor component (5% of the particle mass, as given in Table S3). Gas chromatography–mass spectrometry analysis conducted in a previous study indicated that the contribution of lubricating oil to the sum of the measured components was approximately 80% for particles of 30 nm in diameter (Fushimi et al. Citation2011). Considering these facts, the effective density of the particles with Dm = 30 nm in Diesel A (High idle) mainly indicates the presence of Org-dominant singlet particles rather than agglomerates. There were less soot particles of this size present, and thus it is expected that singlet particles undergo the same generation mechanism as the PFI and LPG particles investigated in this study.
3.4. Physical properties of agglomerates
Detailed image analysis of the agglomerates was conducted. shows the primary particle diameter dp and the number Np of primary particles in agglomerates of each size contained in DEPs and DISI particles. Figure S7 shows an example of the distribution of dp and the lognormal curve fitted to the distribution, and Table S2 lists the averages and standard deviations of dp at different Dm values. The average dp values ranged from 14.3 to 36.7 nm and 12.7 to 34.7 nm for DEPs and DISI particles, respectively. TEM observations of these agglomerates indicated that the number Np of primary particles and the primary particle diameter dp increased as the electrical mobility diameter Dm of the agglomerates increased. At a given Dm, there are no systematic differences between the properties (Np, dp) of agglomerates obtained under different engine operating conditions, engine speeds, average air–fuel equivalence ratios, or types of fuel, even if differences exist between the organic compounds of diesel and gasoline or between the oxygen concentrations in different combustion chambers.
Figure 5. Physical properties of agglomerates. (a) Average primary particle diameter dp. (b) Average primary particle number Np in a single agglomerate. Error bars indicate the standard deviations of the measured values for dp and the propagation of error during each step of the calculation for Np.
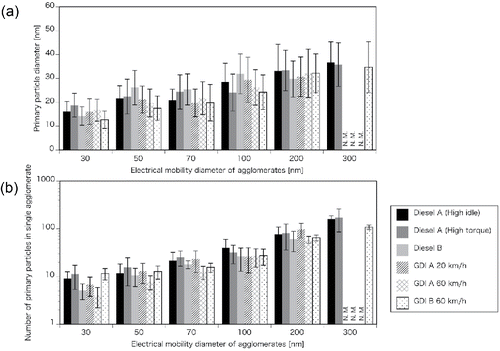
The following empirical relationship was found between Dm and average dp for all agglomerates measured in this study:[4]
The correlation between these two parameters was found to be high (R = 0.94). Furthermore, regarding environmental concerns, this suggests that the apportionment of the sources of agglomerates from diesel and DISI vehicles in an environment with multiple sources of traffic exhaust is difficult using only the properties of the agglomerates.
Previous studies have reported that large agglomerates have large dp values for DISI particles (Barone et al. Citation2012; Lee et al. Citation2013; Seong et al. Citation2013, Citation2014; Dastanpour and Rogak Citation2014) and particles from various other combustion sources (Dastanpour and Rogak Citation2014). Although Mathis et al. (Citation2005) did not present results related to the sizes of agglomerates, they found that dp decreased when the fuel injection pressure was increased, the injection was initiated earlier, or the rate of exhaust gas circulation is lower. They also revealed that dp decreased when the maximum flame temperature increased, rather time available for soot oxidation during the expansion stroke in the combustion chamber was insensitive on the dp. Seong et al. (Citation2013, Citation2014) reported that injection timing affected the agglomerate and primary particle sizes via changing fuel-air mixing for DISI engines. Barone et al. (Citation2012) and Dastanpour and Rogak (Citation2014) also speculated that larger agglomerates composed of larger primary particles form in microscopic fuel-rich regions of the combustion chamber favorable for primary particle formation whereas smaller agglomerates composed of smaller primary particles form in microscopic lean regions, which was suggested by the fact that the primary particle polydispersity in ensembles of soot particles.
In this study, the combustion conditions were not controlled, but particles were emitted under certain steady-state engine operating conditions and were sampled to determine the different agglomerate sizes. Therefore, primary and agglomerates particle sizes may depend on both the local air–fuel equivalence ratio and the local temperature in the combustion chamber.
3.5. Estimation of core diameter and shell thickness of primary particles in agglomerates
The core diameter, which is composed of EC, and the organic coating (shell) thickness of agglomerates was estimated for the high idle operation of Diesel A. In the Diesel A test, particles with Dm = 30 and 50 nm consisted of different types of particles (). Particles with these electrical mobility diameters were excluded from the estimation.
The generation of agglomerates actually involves a complex series of chemical and physical processes. Amann and Siegla (Citation1982) reviewed the generation mechanism of diesel soot agglomerates as follows. Nucleation occurs during combustion, followed by surface growth caused by EC, and finally agglomeration to form soot particles. Surface growth on agglomerates by EC may partially fill the crevices at the junctures of adjoining spherules to provide a peanut-like configuration. These processes occur in an engine cylinder over time. After emission from the tail pipe of the vehicle, the adsorption and condensation of the soot by hydrocarbons begin. Although these processes are discussed as discrete processes, they tend to occur concurrently.
Thus, a soot particle model that models soot particle formation as the condensation of Org after the agglomeration of the EC core (Figure S8) was considered. To derive the core size and shell thickness, the mass of the agglomerate measured by the APM (APM method), the EC and Org fractions from the chemical speciation of the MOUDI sample (MOUDI method), and the material volume of the agglomerate obtained from the number Np of spherical primary particles and their average primary particle diameters dp derived from TEM images (TEM method) were used. Furthermore, the material densities of the EC and Org were considered to be 2.0 and 0.87 g/cm3, respectively (Fuller et al. Citation1999; Geller et al. Citation2006; Park et al. Citation2004), and the elements and inorganic ions in the agglomerates were ignored because they comprised less than 1% of the particle mass (Table S3).
First, the consistency among the MOUDI, APM, and TEM methods was confirmed using the material volume of the agglomerate. The material volume of the agglomerate was determined using the TEM method or calculated from the EC/Org data, the material densities of the EC and Org, and the mass of the agglomerate (APM+MOUDI method). The material volume of the agglomerate derived using the TEM method was found to be systematically lower than that derived using the APM+MOUDI method (Figure S9). It is suspected that the material volume derived using the TEM method is systematically smaller than the actual volume. Although the overlap segment was considered, either the Np or dp value was underestimated. However, the possibility of underestimating dp is considered to be small because dp is directly measured from TEM images whereas Np is estimated and is thus more likely have some uncertainty. Furthermore Dastanpour and Rogak (Citation2014) estimated the shielding effect, which means that smaller particles are more likely to be covered by large particles, and demonstrated that this effect caused Np to be underestimated by a much greater amount than dp. Then, the Np value was corrected such that it agreed with the material volume determined using the APM+MOUDI method (Table S4). The underestimation of Np was found to be 23–43% of the original values. The surface areas of the agglomerates were then calculated using dp and the corrected Np value (Table S4), and the shell thickness was obtained as the Org material volume divided by the surface area of the agglomerate. Assuming that each primary particle is spherical and has one core, the core diameter was calculated as the EC material volume divided by Np. summarizes the plausible properties of agglomerates.
Table 3. Summary of plausible properties (average ± standard deviation) of agglomerates for Diesel A.
The derived core size and shell thickness can be used to obtain the primary particle size, and this size was found to be consistent with the direct measurement by TEM within the measurement variation. The core diameter was estimated to be 41–60% of the measured primary particle diameter dp, and this fractions increases with increasing Dm. Although dp and the core diameter depend on Dm, the shell thickness, which ranged from 5.1 to 7.4 nm, was found to be independent of Dm. The growth of the shell thickness (condensation of Org) should be faster in smaller particles; therefore, the fact that the shell thickness does not depend on Dm means that particles of all sizes have sufficient time for growth while passing through the combustion chamber and tailpipe. Previous thermodenuder experiments (Sakurai et al. Citation2003; Ghazi et al. Citation2013; Graves et al. Citation2015), which are a direct method of determining the change in the mass of a particle with temperature, have indicated that the mass fraction of nonvolatile material of soot particles increases as the mobility size increases. In this study, the primary particle diameter dp and the mass fraction of nonvolatile material (EC core) in agglomerate were found to increase as Dm increased, which corresponds to what were observed in the agglomerates by thermodenuder experiments.
4. Summary
In this study, a DMA–APM method was used to measure the effective density of particles emitted from various fuel engines under steady-state conditions. A TEM was also used to observe the morphology of these particles. This is the first article to report the effective density and TEM images of particles emitted from LPG vehicles. For both DEP and DISI particles, the decrease in the effective density as Dm increased from 30 to 300 nm was caused by agglomeration. The primary particle size and the number of primary particles in an agglomerate were similar for both DEPs and DISI particles, and both were found to increase with increasing Dm. In contrast, the PFI and LPG particles are non-agglomerates with no voids. The round shape of these particles observed in TEM images is a signature of spherical airborne particles, which are likely mainly generated by condensation during the cooling process in the absence of soot particles after exiting the combustion chamber. It is speculated that non-agglomerates mainly consist of organic compounds, which are derived from unburned fuel and lubricating oil with low amounts of EC.
UAST_1218438_Supplementary_file.zip
Download Zip (7.9 MB)Acknowledgments
We thank Prof. K. Sera of the cyclotron center of Iwate Medical University for his assistance with elemental analysis by PIXE. We also thank Mr. T. Goto and Mr. S. Hayami of the LPG Vehicle Promoting Association in Japan for arranging studies of PFI and LPG vehicles and aiding in the discussion of our results. Dr. H. Sakurai of the National Institute of Advanced Industrial Science and Technology, Japan, provided us with useful suggestions for our data. We also thank Ms. S. Kinoshita (NIES), Ms. Y. Yoshikawa (NIES), Mr. T. Fujii (Horiba Techno Service), Mr. H. Konno (Horiba Techno Service), Ms. M. Ihara (NIES), and Mr. Y. Sugaya (NIES) for their excellent technical assistance. We also thank the editor and anonymous reviewers for their careful reading of the manuscript, critical remarks, and valuable insight, which helped us to greatly improve the manuscript.
Funding
This study was mainly supported by competitive funds from the National Institute for Environmental Studies (2013–2015). This research was also partially supported by the Environment Research and Technology Development Fund (C-1002) of the Ministry of the Environment, Japan.
References
- Aikawa, K., and Jetter, J. J. (2014). Impact of Gasoline Composition on Particulate Matter Emissions from a Direct-Injection Gasoline Engine: Applicability of the Particulate Matter Index. Int. J. Engine Res., 15:298–306.
- Amann, C. A., and Siegla, D. C. (1982). Diesel Particulates—What They are and Why. Aerosol Sci. Technol., 1:73–101.
- Barone, T. L., Storey, J. M. E., Youngquist, A. D., and Szybist, J. P. (2012). An Analysis of Direct-Injection Spark-Ignition (DISI) Soot Morphology. Atmos. Environ., 49:268–274.
- Barone, T. L., and Zhu, Y. F. (2008). The Morphology of Ultrafine Particles on and Near Major Freeways. Atmos. Environ., 42:6749–6758.
- Bond, T. C., Habib, G., and Bergstrom, R. W. (2006). Limitations in the Enhancement of Visible Light Absorption Due to Mixing State. J. Geophys. Res-Atmos., 111. doi:10.1029/2006JD007315.
- Brasil, A. M., Farias, T. L., and Carvalho, M. G. (1999). A Recipe for Image Characterization of Fractal-Like Aggregates. J Aerosol Sci., 30:1379–1389.
- Dastanpour, R., and Rogak, S. N. (2014). Observations of a Correlation Between Primary Particle and Aggregate Size for Soot Particles. Aerosol Sci. Technol., 48:1043–1049.
- Donaldson, K., Borm, P. J. A., Oberdorster, G., Pinkerton, K. E., Stone, V., and Tran, C. L. (2008). Concordance Between in vitro and in vivo Dosimetry in the Proinflammatory Effects of Low-Toxicity, Low-Solubility Particles: The Key Role of the Proximal Alveolar Region. Inhal. Toxicol., 20:53–62.
- Donaldson, K., Stone, V., Clouter, A., Renwick, L., and MacNee, W. (2001). Ultrafine Particles. Occup. Environ. Med., 58:211–216.
- Ehara, K., Hagwood, C., and Coakley, K. J. (1996). Novel Method to Classify Aerosol Particles According to Their Mass-to-Charge Ratio—Aerosol Particle Mass Analyser. J Aerosol Sci., 27:217–234.
- Farron, C., Matthias, N., Foster, D. E., Andrie, M., Krieger, R., Najt, P., Narayanaswamy, K., Solomon, A., and Zelenyuk, A. (2011). Particulate Characteristics for Varying Engine Operation in a Gasoline Spark Ignited, Direct Injection Engine. SAE Int. 2011-01-1220. doi:10.4271/2011#01#1220.
- Fujitani, Y., Hirano, S., Kobayashi, S., Tanabe, K., Suzuki, A., Furuyama, A., and Kobayashi, T. (2009). Characterization of Dilution Conditions for Diesel Nanoparticle Inhalation Studies. Inhal. Toxicol., 21:200–209.
- Fujitani, Y., Saitoh, K., Fushimi, A., Takahashi, K., Hasegawa, S., Tanabe, K., Kobayashi, S., Furuyama, A., Hirano, S., and Takami, A. (2012b). Effect of Isothermal Dilution on Emission Factors of Organic Carbon and n-alkanes in the Particle and Gas Phases of Diesel Exhaust. Atmos. Environ., 59:389–397.
- Fujitani, Y., Sakamoto, T., and Misawa, K. (2012a). Quantitative Determination of Composition of Particle Type by Morphology of Nanoparticles in Diesel Exhaust and Roadside Atmosphere. Civil & Environmental Engineering S1:002.
- Fujitani, Y., Sugaya, Y., Hashiguchi, M., Furuyama, A., Hirano, S., and Takami, A. (2015). Particle Deposition Efficiency at Air–Liquid Interface of a Cell Exposure Chamber. J Aerosol Sci., 81:90–99.
- Fuller, K. A., Malm, W. C., and Kreidenweis, S. M. (1999). Effects of Mixing on Extinction by Carbonaceous Particles. J. Geophys. Res., 104:15941.
- Fushimi, A., Kondo, Y., Kobayashi, S., Fujitani, Y., Saitoh, K., Takami, A., and Tanabe, K. (2016). Chemical Composition and Source of Fine and Nanoparticles from Recent Direct Injection Gasoline Passenger Cars: Effects of Fuel and Ambient Temperature. Atmos. Environ., 124:77–84.
- Fushimi, A., Saitoh, K., Fujitani, Y., Hasegawa, S., Takahashi, K., Tanabe, K., and Kobayashi, S. (2011). Organic-Rich Nanoparticles (diameter: 10–30 nm) in Diesel Exhaust: Fuel and Oil Contribution Based on Chemical Composition. Atmos. Environ., 45:6326–6336.
- Geller, M., Biswas, S., and Sioutas, C. (2006). Determination of Particle Effective Density in Urban Environments with a Differential Mobility Analyzer and Aerosol Particle Mass Analyzer. Aerosol Sci. Technol., 40:709–723.
- Ghazi, R., Tjong, H., Soewono, A., Rogak, S. N., and Olfert, J. S. (2013). Mass, Mobility, Volatility, and Morphology of Soot Particles Generated by a McKenna and Inverted Burner. Aerosol Sci. Technol., 47:395–405.
- Graves, B., Olfert, J., Patychuk, B., Dastanpour, R., and Rogak, S. (2015). Characterization of Particulate Matter Morphology and Volatility from a Compression-Ignition Natural-Gas Direct-Injection Engine. Aerosol Sci. Technol., 49:589–598.
- Hinds, W. C. (1999). Aerosol Technology: Properties, Behavior, and Measurement of Airborne Particles. 2nd edn, Wiley, New York, p. 239.
- Kasper, G. (1982). Dynamics and Measurement of Smokes. I Size Characterization of Nonspherical Particles. Aerosol Sci. Technol., 1:187–199.
- Kayes, D., and Hochgreb, S. (1999). Mechanisms of Particulate Matter Formation in Spark-Ignition Engines. 3. Model of PM Formation. Environ. Sci. Technol., 33:3978–3992.
- Kobayashi, S., Kondo, Y., Fushimi, A., Fujitani, Y., Saitoh, K., Takami, A., and Tanabe, K. (2012). Particulate Matter Emissions from Direct Injection Gasoline Passenger Car. Trans. Soc. Automot. Eng. Japan, 43:1009–1014 (in Japanese).
- Lee, J. W., Do, H. S., Kweon, S. I., Park, K. K., and Hong, J. H. (2010). Effect of Various LPG Supply Systems on Exhaust Particle Emission in Spark-Ignited Combustion Engine. Int. J. Auto Tech-Kor., 11:793–800.
- Lee, K. O., Seong, H., Sakai, S., Hageman, M., and Rothamer, D. (2013). Detailed Morphological Properties of Nanoparticles from Gasoline Direct Injection Engine Combustion of Ethanol Blends. SAE Int. 2013-24-0185. doi:10.4271/2013-24-0185.
- Lin, G.-Y., Liao, B.-X., Tzeng, N.-J., Chen, C.-W., Uang, S.-N., Chen, S.-C., Pui, D. Y. H., and Tsai, C.-J. (2014). The Effect of Nanoparticle Convection-Diffusion Loss on the Transfer Function of an Aerosol Particle Mass Analyzer. Aerosol Sci. Technol., 48:583–592.
- Maricq, M. M. (2007). Chemical Characterization of Particulate Emissions from Diesel Engines: A Review. J. Aerosol Sci., 38:1079–1118.
- Maricq, M. M., Szente, J. J., and Jahr, K. (2012). The Impact of Ethanol Fuel Blends on PM Emissions from a Light-Duty GDI Vehicle. Aerosol Sci. Technol., 46:576–583.
- Maricq, M. M., and Xu, N. (2004). The Effective Density and Fractal Dimension of Soot Particles from Premixed Flames and Motor Vehicle Exhaust. J Aerosol Sci., 35:1251–1274.
- Mathis, U., Kaegi, R., Mohr, M., and Zenobi, R. (2004). TEM Analysis of Volatile Nanoparticles from Particle Trap Equipped Diesel and Direct-Injection Spark-Ignition Vehicles. Atmos. Environ., 38:4347–4355.
- Mathis, U., Mohr, M., Kaegi, R., Bertola, A., and Boulouchos, K. (2005). Influence of Diesel Engine Combustion Parameters on Primary Soot Particle Diameter. Environ. Sci. Technol., 39:1887–1892.
- Miller, A. L., Stipe, C. B., Habjan, M. C., and Ahlstrand, G. G. (2007). Role of Lubrication Oil in Particulate Emissions from a Hydrogen-Powered Internal Combustion Engine. Environ. Sci. Technol., 41:6828–6835.
- Momenimovahed, A., and Olfert, J. S. (2015). Effective Density and Volatility of Particles Emitted from Gasoline Direct Injection Vehicles and Implications for Particle Mass Measurement. Aerosol Sci. Technol., 49:1051–1062.
- Momenimovahed, A., Olfert, J. S., Checkel, M. D., Pathak, S., Sood, V., Robindro, L., Singal, S. K., Jain, A. K., and Garg, M. O. (2013). Effect of Fuel Choice on Nanoparticle Emission Factors in LPG-Gasoline bi-Fuel Vehicles. Int J Auto Tech-Kor., 14:1–11.
- Myung, C. L., Kim, J., Choi, K., Hwang, I. G., and Park, S. (2012). Comparative Study of Engine Control Strategies for Particulate Emissions from Direct Injection Light-Duty Vehicle Fueled with Gasoline and Liquid Phase Liquefied Petroleum Gas (LPG). Fuel, 94:348–355.
- Nakao, S., Shrivastava, M., Nguyen, A., Jung, H. J., and Cocker, D. (2011). Interpretation of Secondary Organic Aerosol Formation from Diesel Exhaust Photooxidation in an Environmental Chamber. Aerosol Sci. Technol., 45:964–972.
- Nakashima, Y., Kamei, N., Kobayashi, S., and Kajii, Y. (2010). Total OH Reactivity and VOC Analyses for Gasoline Vehicular Exhaust with a Chassis Dynamometer. Atmos. Environ., 44:468–475.
- Oberdorster, G. (2000). Toxicology of Ultrafine Particles: in vivo Studies. Philos. Trans. A Math. Phys. Eng. Sci., 358:2719–2739.
- Okada, K., and Heintzenberg, J. (2003). Size Distribution, State of Mixture and Morphology of Urban Aerosol Particles at Given Electrical Mobilities. J Aerosol Sci., 34:1539–1553.
- Olfert, J. S., Symonds, J. P. R., and Collings, N. (2007). The Effective Density and Fractal Dimension of Particles Emitted from a Light-Duty Diesel Vehicle with a Diesel Oxidation Catalyst. J Aerosol Sci., 38:69–82.
- Park, K., Cao, F., Kittelson, D. B., and McMurry, P. H. (2003). Relationship Between Particle Mass and Mobility for Diesel Exhaust Particles. Environ. Sci. Technol., 37:577–583.
- Park, K., Kittelson, D. B., and McMurry, P. H. (2004). Structural Properties of Diesel Exhaust Particles Measured by Transmission Electron Microscopy (TEM): Relationships to Particle Mass and Mobility. Aerosol Sci. Technol., 38:881–889.
- Pirjola, L., Karjalainen, P., Heikkila, J., Saari, S., Tzamkiozis, T., Ntziachristos, L., Kulmala, K., Keskinen, J., and Ronkko, T. (2015). Effects of Fresh Lubricant Oils on Particle Emissions Emitted by a Modern Gasoline Direct Injection Passenger Car. Environ. Sci. Technol., 49:3644–3652.
- Poland, C. A., Duffin, R., Kinloch, I., Maynard, A., Wallace, W. A. H., Seaton, A., Stone, V., Brown, S., MacNee, W., and Donaldson, K. (2008). Carbon Nanotubes Introduced into the Abdominal Cavity of Mice Show Asbestos-Like Pathogenicity in a Pilot Study. Nature Nanotech., 3:423–428.
- Quiros, D. C., Hu, S., Hu, S., Lee, E. S., Sardar, S., Wang, X., Olfert, J. S., Jung, H. S., Zhu, Y., and Huai, T. (2015). Particle Effective Density and Mass During Steady-State Operation of GDI, PFI, and Diesel Passenger Cars. J. Aerosol Sci., 83:39–54.
- Ristovski, Z. D., Jayaratne, E. R., Morawska, L., Ayoko, G. A., and Lim, M. (2005). Particle and Carbon Dioxide Emissions from Passenger Vehicles Operating on Unleaded Petrol and LPG Fuel. Sci. Total Environ., 345:93–98.
- Ronkko, T., Virtanen, A., Kannosto, J., Keskinen, J., Lappi, M., and Pirjola, L. (2007). Nucleation Mode Particles with a Nonvolatile Core in the Exhaust of a Heavy Duty Diesel Vehicle. Environ. Sci. Technol., 41:6384–6389.
- Sager, T. M., and Castranova, V. (2009). Surface Area of Particle Administered Versus Mass in Determining the Pulmonary Toxicity of Ultrafine and Fine Carbon Black: Comparison to Ultrafine Titanium Dioxide. Part. Fibre Toxicol., 6:15.
- Sakurai, H., Park, K., McMurry, P. H., Zarling, D. D., Kittelson, D. B., and Ziemann, P. J. (2003). Size-Dependent Mixing Characteristics of Volatile and Nonvolatile Components in Diesel Exhaust Aerosols. Environ. Sci. Technol., 37:5482–5495.
- Schmid, O., Karg, E., Hagen, D. E., Whitefield, P. D., and Ferron, G. A. (2007). On the Effective Density of Non-Spherical Particles as Derived from Combined Measurements of Aerodynamic and Mobility Equivalent Size. J. Aerosol. Sci., 38:431–443.
- Seong, H., Choi, S., and Lee, K. (2014). Examination of Nanoparticles From Gasoline Direct-Injection (GDI) Engines using Transmission Electron Microscopy (TEM). Int. J. Auto. Tech-.Kor. 15:175–181.
- Seong, H., Lee, K., and Choi, S. (2013). Effects of Engine Operating Parameters on Morphology of Particulates from a Gasoline Direct Injection (GDI) Engine. SAE Int.
- Sgro, L. A., Sementa, P., Vaglieco, B. M., Rusciano, G., D'Anna, A., and Minutolo, P. (2012). Investigating the Origin of Nuclei Particles in GDI Engine Exhausts. Combust. Flame, 159:1687–1692.
- Sorensen, C. M. (2011). The Mobility of Fractal Aggregates: A Review. Aerosol Sci. Technol., 45:765–779.
- Stanton, M. F., Layard, M., Tegeris, A., Miller, E., May, M., Morgan, E., and Smith, A. (1981). Relation of Particle Dimension to Carcinogenicity in Amphibole Asbestoses and Other Fibrous Minerals. J. Natl. Cancer Inst., 67:965–975.
- Virtanen, A., Ristimäki, J., Marjamäki, M., Vaaraslahti, K., Keskinen, J., and Lappi, M. (2002). Effective Density of Diesel Exhaust Particles as a Function of Size. SAE Int. 2002-01-0056.
- Virtanen, A. K., Ristimaki, J. M., Vaaraslahti, K. M., and Keskinen, J. (2004). Effect of Engine Load on Diesel Soot Particles. Environ. Sci. Technol., 38:2551–2556.
- Wittmaack, K. (2007). In Search of the Most Relevant Parameter for Quantifying Lung Inflammatory Response to Nanoparticle Exposure: Particle Number, Surface Area, or What? Environ. Health Perspect., 115:187–194.