ABSTRACT
A particle generation apparatus and methodology is described for its application to prepare heterogeneous particles of defined chemical composition and their subsequent delivery to human lung cell cultures. A concentric type nebulizer was used together with a single pass spray chamber that was operated at two different temperature settings, either room temperature or to produce intact particles, at nonuniform temperature with the central axis measuring 54°C. Silica (SiO2) particles in the micrometer size range were produced from the aggregation of nanoparticles. Tumor necrosis factor (TNF)-α, a well-characterized cytokine, was incorporated as a secondary soluble component within host silica particles for application as a proof-of-concept heterogeneous particle type. The overall particle deposition and wetting efficiency into media was 2.0 ± 0.4%, and the average size of particles that impacted and sank in the growth medium was 3.4 µm. The particle dose, reported as particle-to-cell ratio, spanned 0.1 to ∼2.5. Co-cultures of A549 and differentiated human monocytic cells (THP-1*) exposed to silica particles caused IL-6 upregulation, but not IL-8. For the silica plus TNF-α particle type, ICAM-1 signal increased in A549 mono-cultures as the particle-to-cell ratio increased, yet an estimated 90% of the TNF-α was denatured or possibly bound to the silica particle host, and was therefore bio-unavailable. Method quantitation using particles having two different chemical compositions has been described, illustrating capability for subsequent systematic investigation of the role of particles having different chemical composition in the human health aspects of the particulate air pollution issue.
Copyright © 2016 American Association for Aerosol Research
EDITOR:
Introduction
Numerous particle-centric approaches have been developed to learn information regarding the relationship between particulate matter (PM) exposure and adverse effects on human health (Bakand et al. Citation2005; Elder et al. Citation2004; Grass et al. Citation2010; Sillanpää et al. Citation2008). One strategy is the use of sampled ambient particles (Akhtar et al. Citation2014; Dagher et al. Citation2005; Farina et al. Citation2013; Zosky et al. Citation2014). Encompassing coarse through to ultrafine particle sizes, size segregated or not, as well as water and insoluble fractions, all have been used in dose-response studies, and all have been observed to up-regulate pro-inflammatory mediators (Jalava et al. Citation2005; Naimabadi et al. Citation2016; Soukup et al. Citation2000; Velali et al. Citation2016). Within the soluble fraction, many small oxidized organic compounds, transition metals, sodium, ammonium, nitrate, and sulfate ions have been identified, whereas the insoluble fraction is comprised of low solubility minerals, soots, automobile wear particles (e.g., brake pads and tire fragments), and industrial-related dusts (Adamson et al. Citation1999; Clague et al. Citation1999; Ghio et al. Citation1999; Imrich et al. Citation2000; Watson and Valberg Citation2001).
In the use of sampled ambient particle types, dose–response studies often introduce particles as aqueous suspensions to either growth medium bathing lung cell cultures or instilled in live subjects (Berube et al. 2009; Suwa et al, Citation2002). These particular studies probe the whole spectrum of ambient particle chemical composition. In contrast, studies that employ the same general dose methodology but use commercially-available particles as mimics of specific insoluble particle types in PM to probe their responses in dose response studies. Silica particles, for instance, have been characterized extensively (Hetland et al. Citation2001; Lin et al. Citation2006; Wottrich et al. Citation2004). Extension of this general dose-response approach, by introducing additional components to the dosing regimen, has also been described (Kaan and Hegele Citation2003; Schulz et al. Citation2002). Priming involves the introduction of a secondary, soluble component to the cell culture medium prior to a time-delayed introduction of an insoluble particle type. Lipopolysaccharide (LPS), a component in the cell wall of Gram negative bacteria, has been introduced to the growth medium of a cell culture in its soluble form prior to the addition of carbon nanotubes (CNTs) (Pulskamp et al. Citation2007). Such combined exposures have resulted cellular responses that were described as synergistic (Mauderley and Samet Citation2009). In the aforementioned CNT + LPS study, the LPS-primed cells showed increased cytokine TNF-α secretion upon CNT treatment (Pulskamp et al. Citation2007).
In another variation, apparatuses have been designed to more closely mimic inhalation exposure, wherein aerosols are generated in situ and then delivered onto cell cultures (Brobell et al. Citation2013; Haddrell et al. Citation2005; Lenz et al. Citation2009; Sillanpää et al. Citation2008). The rationale for this approach offers potential of in situ mimicry of atmospheric particle chemical composition and delivery of particle to lung tissue via the air using, for example, cultures grown at the air-liquid interface (Bitterle et al. Citation2006).
In this work, described is a particle generation and exposure system capable of delivering micrometer-size particles at controlled compositions, containing both insoluble and soluble fractions, onto cell cultures, and methodology to attain overall dose-response quantitation. The apparatus used to generate aerosols, whose composition can be customized through alteration of the starting mixture, was a commercially available concentric nebulizer coupled with a large internal volume spray chamber (Boumans Citation1987). Analogous apparatuses are used in studies of ordered particle structures (Motl et al. 2013; Lee et al. 2016). An intended use of this apparatus and methodology is to investigate instances of synergy between ambient particle compounds based on the down-stream effects as reported by lung cell culture responses to identify which compositions are comparatively more toxic.
The particle composition chosen for this study was silica, also known as silicon dioxide or SiO2. Silica is high in abundance in PM10 (U.S. EPA Citation1996). Silica is a mineral predominately found in rocks, sands and soils. Natural or anthropogenic activities involving the movement of earth such as wind erosion, volcanic eruptions, farming, mining, or disturbances of silica-containing compounds, as in the fabrication of concrete, can result in silica emissions. A study in Rome, Italy, reported total silica content, amorphous plus crystalline, as 3.7% relative to nine other common PM10 species, e.g. carbonates, metals, and sulphates, based on the detection frequency of particles (Puledda et al. Citation1999). In that same study, the mass concentration (µg m−3) of quartz, the most common form of crystalline silica, averaged 2.6% mass content relative to total PM10. In another study, samples were collected upwind and at multiple sites downwind from a sand and gravel facility in central California (Shiraki and Holmen Citation2002). Quartz was reported at values ranging from 10% to 16% upwind and 10% to 33% downwind based on its mass fraction relative to PM10. The levels of silica, as with all primary aerosols, vary spatially due to proximity to, and activity from, emission sources.
Silica inhalation exposure has been linked to several respiratory diseases (OSHA Citation2016). For example, silicosis describes the condition in which inflammation and subsequent fibrotic scarring leads to symptoms such as dry cough, increased sputum production, and impairment of lung function (Leung et al. Citation2012). In 1997, the International Agency for Research on Cancer (IARC) classified crystalline silica as carcinogenic to man, (IARC Working Group on the Evaluation of Carcinogenic Risks to Humans) while chronic obstructive pulmonary disease (COPD) is another substantial respiratory disease linked to inhalation exposure to silica (U.S. EPA Citation1996). With the negative human health affects of silica, and its abundance in the atmosphere, it was selected as the primary, insoluble component, in this study. In addition, given the sources of silica, silica and other crustal dusts are known to transport biological molecules and organisms that cause strong immunological responses into the atmosphere (Brodie et al. 2007; Tang Citation2009).
Tumor necrosis factor (TNF)-α is a potent cytokine that is released during inflammation. TNF-α has also been used extensively as a positive control in in vitro experimentation. Although TNF-α is not present in the atmosphere, it was incorporated into the silica particles for the purpose of demonstrating the ability to dose multi-component particles types. In addition, the application of the silica + TNF-α particles was performed specifically to quantitate the methodology, through measurement of comparative responses from lung cells dosed with these particle types relative to soluble TNF-α.
The human alveolar epithelial cell line A549 (ATCC) is commonly used in dose–response studies and was used as the lung monoculture model for this work. Human lung cell co-cultures comprised of A549 cells and macrophages have been reported as having increased sensitivity to particles regarding cytokine secretion relative to A549 monocultures. Intercellular communication between different cell types within co-cultures provides a more accurate representation of the in vivo environment of the lung (Tao and Kobzik Citation2002). Macrophage-like cells, originating from differentiation of human monocytic cell lines THP-1 (ATCC), were also used in co-culture with A549 cells in this work to extend the dynamic range of cytokine response to lower particle doses (Alfaro-Moreno et al. Citation2007; Wottrich et al. Citation2004).
Experimental
Materials
LUDOX® TM-50 (Sigma-Aldrich, 420778, Oakville, Ontario, Canada) is a silica colloid of amorphous nanoparticles ranging from 20 to 30 nm in diameter; density 1.4 g cm−3. LUDOX® TM-50 was used as the source of silica as the primary composition of particles generated and dosed onto cultures in this work. Polyethylene glycol (PEG) (Sigma-Aldrich, 202444), with an average molecular weight of 3,350 g mol−1, was incorporated into the particles to assist in the wettability of deposited particles. FluoSpheres® (Invitrogen, F-8787, Burlington, Ontario, Canada), provided at 2% solids as a colloid, are latex beads approximately 20 nm in diameter that contain an estimated ∼180 fluorescein molecules entrapped within each bead. These beads were incorporated into the particles to facilitate particle observation, via fluorescence microscopy, when particles were deposited onto dry cover slips, into growth medium, and when in contact with and internalized in cells.
The components described above were used to prepare a starting mixture with the following composition: 11 mg mL−1 silica, 7.85 mg mL−1 PEG, and 1 mg mL−1 FluoSpheres® in distilled deionized water collected at ≥ 18.0 MΩ. The term starting mixture is used since silica and FluoSpheres® were in colloidal form, whereas the PEG was in solution because the solution was nebulized and the resultant aerosol processed during transit through a spray chamber. The starting mixture was mixed using a vortex mixer and sterile filtered with a 0.2 micrometer filter (VWR, 28145-477) immediately prior to use. After filtration, the starting mixture was aerosolized using the apparatus described below. De-solvation of aerosol droplets produced a single particle per droplet for the spray chamber operated at elevated temperature, and each silica particle is referred to using the abbreviation Another starting mixture was prepared with the silica and PEG at the same concentrations, but the FluoSpheres® were replaced by fluorescein sodium salt (Sigma-Aldrich, F6377) at 500 ng mL−1 (∼13 nM).
In several experiments, human TNF-α (Sigma-Aldrich, H8916) was incorporated at 5 µg mL−1 into the starting mixture along with silica, PEG, and FluoSpheres®, at their respective concentrations defined above. The 5 µg mL−1 concentration used for TNF-α corresponds to a 2200:1 mass ratio for silica:TNF-α, or, the number of SiO2 nanoparticles (20-30 nm) to the number of soluble TNF-α monomers as ∼1:3. Particles created with this starting mixture are abbreviated as .
A549 cell culture
A549 cells (American Type Culture Collection (ATCC), CCL-185), a type II human alveolar bronchoalveolar carcinoma cell line, were used as the monoculture. Passage numbers for the A549 cells in monocultures were from 88 to 110. In preparation for receipt of a particle dose, cell cultures were grown to ∼90% confluence on “Cell+” 6-well plates (Sarstedt, 83.1839.300) in growth medium composed of F-12K medium (Sigma-Aldrich, N3520), which included 10% (v/v) fetal bovine serum (Invitrogen, 12483020). Prior to particle exposure, the growth medium was removed and replaced with 2 mL of serum-free medium. Cell counting was performed with a hemacytometer (Hausser Scientific, 1492). Unless stated otherwise, cells were counted prior to the deposition of particles.
THP-1 cells
THP-1 cells (ATCC, TIB-202) are a peripheral blood monocyte cell line and are cultured in growth medium composed of Roswell Park Memorial Institute (RPMI) 1640 medium (ATCC, 30-2001), 10% (v/v) fetal bovine serum, and 0.05 mM 2-mercaptoethanol (Sigma-Aldrich, M3148). The passage number was listed as unknown upon receipt. Those cells were passaged from 1 to 20 in the course of this work. To differentiate into macrophages, THP-1 cells were treated with 200 nM phorbolmyristate acetate (PMA) (Invitrogen, TLRL-PMA) and incubated for 48 hours. These differentiated cells will be referred to as THP-1* cells henceforth. THP-1* cells are unlike their nondifferentiated form in that they no longer divide and they do adhere to the surface of the culture dish.
Monocultures of THP-1* cells were planned for dose-response experiments, but ultimately were not performed because of the difficulty to obtain a confluent culture. It was observed that if too many THP-1 cells (blood monocytes) were differentiated into THP-1* cells (macrophages), those cells underwent facile aggregation resulting in regions of over-confluence and regions with little to no cells present. Given this practical difficulty, and that monocultures of macrophages were not physiologically relevant to this work, THP-1* monocultures were not utilized in this study.
Co-culture of A549 and THP-1 cells
Co-cultures were comprised of A549 cells and THP-1* cells at a 15:1 ratio by growing cells at the seeding densities and times described below. The co-cultures took four days to grow to confluence. On day one, A549 cells were seeded into Sarstedt ‘Cell+’ 6-well plates at ∼150,000 cells per well in F-12K growth medium. ∼55,000 THP-1 cells per well were added to each well on day two in a 1:1 ratio of F-12K growth medium and RPMI growth medium with 200 nM PMA. By day four the co-cultures grew to ∼90% confluence, and the THP-1 cells differentiated into THP-1* cells. Also on day four, the medium was replaced with serum-free medium of the two medium types (F-12K:RPMI at a 1:1 (v/v) ratio plus 200 nM PMA) prior to particle exposure. For the co-cultures, passage numbers of the A549 cells were between 110 and 120. Assessment of the number of cells was ascertained directly by counting cells using a hemacytometer after particle exposure plus an 18-hour incubation period.
Aerosol generation
Prior to working with cell cultures, aerosol generation was performed using a different starting solution for the purpose of providing information regarding droplet primary size generated by the nebulizer, and to measure airborne particle sizes exiting the spray chamber. A 25 mM aqueous solution of MgCl2 (Sigma-Aldrich, M9272) was used. A Sioutas Cascade Impactor (SKC Inc., Houston, Texas, USA) and a Rotary Pump (Allegro Safety T100M Rotary Vane, Piedmont, South Carolina, USA) were used as recommended by the manufacturer to collect size-fractionated particles of MgCl2(s) that exited the spray chamber on filters within the impactor. Sonication of each filter in aqueous solution at pH 3 was performed prior to determining the mass of Mg2+ recovered from each filter using atomic absorption spectrometry (AAS) and calibration using the method of external standards. The results obtained were, particle size and recovery, respectively, >2.5 µm 1 ± 1%, 1.0-2.5 µm 12 ± 4%, 0.5-1.0 µm 22 ± 2%, 0.25-0.5 µm 30 ± 3%, and <0.25 µm 9 ± 2% (n = 3). This data established the capability to generate particles with a size distribution having a mean particle size in the fine fraction (i.e., <2.5 µm) for a starting solution of MgCl2 at 25 mM. The overall recovery of MgCl2 was 75% ± 5 (n = 6), with the difference impacting on the wall of the spray chamber.
With knowledge of the average MgCl2(s) particle size generated, an estimate of the primary droplet size was evaluated, assuming the average particle was ∼3 micrometers in diameter, was solid and spherical, and a density of magnesium chloride 2.3 g cm−3 was used. The average mass of MgCl2 per particle was calculated as ∼0.15 pg. Based on the initial concentration of the starting solution, 25 mM, the original volume of starting solution per primary droplet was calculated as ∼23 micrometers in diameter. This primary droplet average size calculation will have been an overestimate if the particles that impacted on to the filters of the cascade impactor had not completely dried.
Particle generation and delivery to cell cultures
The apparatus assembled to nebulize starting mixtures and to deliver particles is depicted in . A 1 ml syringe was connected to an infusion pump (Harvard Apparatus, 702000) to deliver a starting mixture to a concentric nebulizer (Meinhard, TR-50-A1) at a rate between 1 to 250 µL min−1. Concentric nebulizers of this design have been used extensively for liquid sample introduction in plasma atomic spectroscopy (Boumans Citation1987). Meinhard Fit Kits #1 and #2 were used to connect the syringe outlet to the tubing attached to the solution inlet of the nebulizer and the gas flow to the nebulizer, respectively. The unrestricted solution uptake of the nebulizer's flow rate was ∼1 mL min−1. However, restricted solution uptake rates were used. This approach increases sample throughput efficiency from the point of introduction to the nebulizer through to the exit of the spray chamber, and is referred to as starving the nebulizer (Longerich Citation1993; Sánchez et al. Citation2010). The reduced liquid sample flow rate decreases initial droplet size in the aerosol, reduces impaction of droplets on the spray chamber wall and onto other droplets, and introduces less overall solvent into the spray chamber. Collectively, this results in an overall improvement in throughput efficiency for the combined steps of aerosol generation and transmission through the spray chamber. Nebulant gas flow was controlled by a mass flow controller (MKS Instruments, 1159B, Andover, Massachusetts, USA) used in combination with a single channel power supply readout (MKS Instruments, 246B) at the settings recommended by the manufacturer, 1 L min−1, 50 psi. The nebulant gas was extra-dry compressed air (Praxair, AI 0.0XD-T, Burnaby, British Columbia, Canada).
Figure 1. Depiction of the aerosol generation apparatus. (a) Sample solution inlet. (b) Nebulant gas (extra-dry air) inlet. (c) Concentric nebulizer. (d) Single pass spray chamber. (e) Six-well plate. Diagram is not to scale.
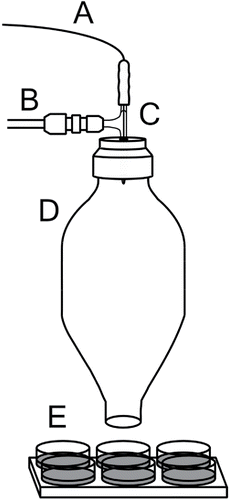
The spray chamber used is described as single-pass, after the geometric design of the chamber. With the nebulant gas flow rate of the nebulizer set at its optimum design rate of 1 L min−1, the gas flow inside the spray chamber was turbulent. The aerosol generated by the nebulizer entered a spray chamber that was maintained at either room temperature (RT) or a nonuniform elevated temperature (nuET) via external heat lamps to increase the rate of solvent evaporation from the droplets. Temperatures were measured at several locations within the chamber at steady state. This was performed while nebulizing ultrapure water through the chamber. Under steady-state conditions, a glass laboratory thermometer was inserted through the spray chamber outlet whilst mounted on a stand. The center of the chamber and chamber outlet were measured as 54°C and 34°C, respectively. Temperatures within the spray chamber at positions closer to the external lamps gave higher recordings, and were as high as 89°C. The air temperature adjacent to the sample solution inlet tubing (A in) did not exceed 36°C. In use, each droplet within the aerosol underwent loss of solvent, and the dispersed solids, silica nanoparticles and FluoSpheres® aggregated. For all and
results reported, the spray chamber was operated at the nuET unless stated otherwise.
In dosing a cell culture with particles, the outlet of a spray chamber having an internal diameter (di) of 27.8 mm was positioned directly over-top a single well (di = 35 mm) of a 6-well plate at a separation gap of 20 mm. After the exposure time for each well had elapsed, the chamber outlet was positioned over another well in sequence until the dosing requirements were achieved for the entire 6-well plate. Variable exposure times were employed to generate different particle-cell dose ratios. The volume of serum-free medium used per well (2 mL) was a trade-off between trying to minimize the depth of the serum-free medium to keep the concentration of soluble compounds delivered on particles as high as possible and keep secreted mediators at detectable concentrations whilst having a depth of serum-free medium necessary to avoid cell death due to drying. Particle delivery to each well, as described above, was performed in a sterile environment in a certified biosafety hood. After deposition of particles, samples were placed in an incubator for 18 hours. When assessing the size distribution of that settled onto the surface of a cell culture, measurements were made 2 h. and 18 h. after particle exposure using microscopy and multi-purpose software Vision Assistant (National Instruments, Austin, Texas, USA).
Additional preliminary work involving cell cultures investigated air-liquid interface supports for cell cultures. The flow rate of gas exiting the spray chamber caused drying and ultimately cell death during the <10 min. exposure period. Attempts to increase the hydrostatic pressure to prevent cell drying were unsuccessful. Thus, our chosen methodology to prepare particles precluded the use of air-liquid interface supports.
To obtain a measure of particle deposition and wetting efficiency, another starting mixture was prepared using the same concentrations of SiO2 and PEG, fluorescein at 13 nM, but no FluoSpheres®. In this experiment, the liquid introduction rate into the nebulizer was 5 µL min−1, and the duration of nebulization was 5 min. After an incubation period of 2 h, the supernatant (2 mL) was collected and transferred into a cuvette. The fluorescence signal intensity measured from these solutions was compared to external calibration curves prepared by adding known concentrations of fluorescein directly to serum-free medium. The working assumption was that the fluorescein introduced to the serum-free medium on the carrier particles was 100% detectable by fluorescence spectroscopy.
To obtain a measure of the bioavailability of TNF-α from intact wetted particles, were dosed into medium in wells containing serum free medium, but not cells. Starting mixtures were nebulized for 25 minutes at the nuET. This step was followed by an incubation period of 2 h, after which the serum-free medium was either transferred directly into another well containing A549 cells, or was filtered (0.2 µm pore size) to remove particles larger than 0.2 µm from the serum-free medium. The filtrates were then transferred to different wells containing a culture of A549 cells. Following an 18-h incubation period, all of these cultures were harvested and processed for ICAM-1 expression.
Electron microscopy
Samples of that were generated and transmitted through the spray chamber were directed at an individual well of a six-well plate, where each well contained 2 mL of distilled deionized water. Particles that impacted into the water were subsequently collected by aspiration into a plastic vial. Aliquots of these suspensions were applied to silicon wafers and the samples were dried in preparation for electron microscopy imaging (FEI/Aspex, model Explorer; FEI, model Helios).
Immunocytochemistry assay for ICAM-1
Intercellular adhesion molecule (ICAM)-1 is an epithelial cell membrane protein whose function is to facilitate the migration of leukocytes from the blood stream into underlying tissue, and its expression is up-regulated by cytokines, including TNF-α and interleukin (IL)-6 (Scheller et al. Citation2011; van de Stolpe and van der Saag Citation1996). To detect changes in ICAM-1 expression, immunofluorescence microscopy was used. Upon removal of supernatant, cells were rinsed three times with 2 mL of 37°C 1X phosphate buffed saline (PBS) (Thermo Fisher Scientific, OXBR0014G, Burlington, Ontario, Canada). The cells were fixed with 4% (w/v) paraformaldehyde (Polysciences Inc., 00380-1, Warminster, Pennsylvania, USA) in 1X PBS for 20 minutes at 37°C. After fixation, cells were rinsed three times with 2 mL of 1X PBS at 37°C then serum-free protein block (DaKo, X0909, Via Real Carpinteria, California, USA) was added for 20 minutes to reduce nonspecific staining. Following removal of the protein block primary antibody solution, 2 µg mL−1 mouse anti-human monoclonal antibody (Invitrogen, 07-5403) in 1% (w/v) bovine serum albumin (Sigma-Aldrich, A7030) in 1X PBS), was added and cells were incubated for 1 hour. The samples were rinsed three times with 2 mL 1X PBS, with the second rinse 5 min before removal. The secondary antibody solution (20 µg ml−1 fluorescently labeled goat anti-mouse antibody [Invitrogen, A-11003] in 1% [w/v] BSA in 1X PBS]) was added and kept in the dark for 30 min. The samples were rinsed three times with 2 mL 1X PBS, with the second rinse 1 hour before removal. The samples stored in 2 mL 1X PBS, at 4°C, and in the dark until analyzed. The 18-h time period used was a typical incubation period following a dose necessary to observe maximum stimulated ICAM-1 up-regulation.
Fluorescent microscopy and image analysis
An inverted microscope (Motic, model AE31 Trinocular, Richmond, British Columbia, USA) equipped with an Epi-Fluorescence illuminator (Motic, AE30/31 EPI), filter blocks (Motic, MUB-I and MG-I) and a mounted camera (Motic, Moticam 3000) were used to capture bright-field images and fluorescent emission profiles at 515 nm and 590 nm of A549 of cell cultures exposed to particles. A 10X objective lens was used for each image and the area of each image was calibrated to 0.32 mm2. A bright-field image and both fluorescent emission profiles (515 nm and 590 nm) were recorded at a single random location within each well after the ICAM-1 assay, and then repeated at two other different random locations within each well.
Images recorded via bright-field settings indicated cell distribution, and emission profiles at 515 nm and 590 nm revealed particles and ICAM-1 expression, respectively. Representative combined figures of the three images, assembled using Adobe Photoshop (Adobe Systems, Mountain View, California, USA) are presented. The ICAM-1 images were analyzed as corrected integrated densities and measured via ImageJ software (National Institute of Mental Health, Bethesda, Maryland, USA). The majority of the cells expressed ICAM-1, and as the cultures were grown to near confluence, the entire image was selected as the highlighted region and the background was measured at the four corners of the image where cells where absent. The corrected integrated densities of samples were then processed relative to negative and positive controls normalized to 0 and 1, respectively. To count the total number of particles per image and determine particle size distribution, fluorescent images recorded at 515 nm emission were run through an in-house script developed within a multi-purpose software program (National Instruments, v8.5). All images were reviewed manually, and objects counted incorrectly by the software were manually removed from the data set. All data were either tabulated and/or plotted using Excel (Microsoft Corporation, Seattle, WA, USA) and/or Igor Pro (Wavemetrics, Lake Oswego, Oregon, USA) software.
IL-6 and IL-8 quantitation using enzyme linked immunosorbant assay (ELISA)
Cell supernatants were collected after the 18-h incubation period, stored at 4°C, and were analyzed within two weeks of collection ELISA kits (Peprotech, 900-M16 and 900-M18, Rocky Hill, Connecticut, USA) were used to quantitate IL-6 and IL-8 concentrations in cell culture supernatants. The kits were used as per the protocols provided by the supplier. Dilution factors of 10X and 50X were applied to the 10 ng ml−1 TNF-α A549 controls, as well as all co-culture samples and controls for IL-6 and IL-8, respectively. This dilution step ensured that signal responses from samples were bracketed within the respective calibration curves. Derived values were then corrected based on their corresponding dilution factor.
Statistical analysis
Analysis of data for soluble concentration of fluorescein and TNF-α was determined using method of external standards and least squares regression analysis. Data reported in the results sections were reported as means ± standard deviation (1σ) and analyzed with Student's t-tests for comparison. Significance was assigned to those with a p-value <0.05 and reported as <0.01 when obtained.
Results and discussion
Particle delivery (impaction and wetting) into serum-free media
With the chamber at room temperature, silica particles that exited the spray chamber and impacted into the serum-free medium were observed to not remain intact, both at the air-liquid interface as well as those that had sank through the medium and were in contact with cells. In addition, these particles were also directed to impact onto the dry surface of a microscope slide, and when viewed using bright field optical microscopy, approximately 40% had visibly wetted the surface (). Based on these observations, the particles that passed through the spray chamber at room temperature, and aggregated and agglomerated depending on the individual particle's quantity of water retained (Sokolov et al. Citation2015).
Figure 2. Images of . Particles observed using bright field optical microscopy after collection on a microscope slide with the spray chamber maintained at (a) room temperature and (b) nonuniform elevated temperature. Images obtained by electron microscopy with the spray chamber at the elevated temperature with (c) a large population of particles, in which the particles appeared as (d) closed and (e) not fully closed.
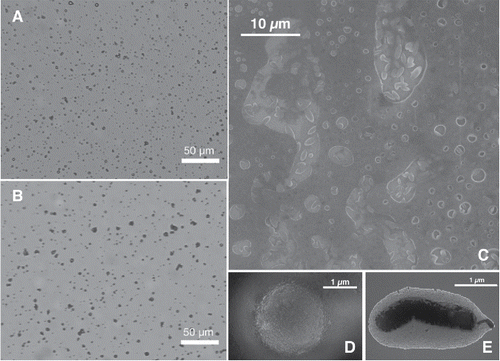
To increase particle intactness in aqueous solutions, the spray chamber was heated to a nonuniform elevated temperature (nuET; ). Silica particles were observed to remain intact upon impact onto the air-liquid interface of the serum-free growth media. However, an estimated 1% of those particles wetted and sank to reach the cell culture surface 2 h. after exposure. Polyethylene glycol (PEG) was introduced to the starting mixtures used to prepare silica particles with the assumptions that particle wettability would increase and lung cell responses would not be affected. For the silica particles that also contained PEG, 57 ± 6% (n = 3) of the particles that impacted into the growth medium were observed to wet and pass below the air-liquid interface 2 h. after exposure. Of the wetted particles, an estimated 95% of those sank to the bottom of the well and contacted the cell surface. The increased particle count on the cell surface was attributed to the increased wettability and/or increased mass of the particles. PEG was henceforth a component present in all subsequent silica particle types.
that exited the spray chamber were also directed at a well of a six-well plate that contained distilled deionized water. These samples were then aspirated into a plastic vial. Aliquots of these samples were dispensed onto silicon wafers and dried prior to characterization by electron microscopy (). Many particles are observable in panel C. The particles can be categorized as spherical or nonspherical. Representative images of these particles at higher magnification are in and , respectively, where these shapes can be further categorized as closed or nonclosed. This terminology is made with reference to the soluble compounds, also present in the starting solutions used to create
and the likelihood that soluble species could be retained within a closed particle. Based on these observations, the particles exiting the spray chamber held at nonuniform elevated temperature had aggregated.
The overall particle deposition and wetting efficiency, relative to the starting material applied to the nebulizer, was calculated as 2.0 ± 0.4%. However, the images shown in indicate that approximately 50% of the particles are spherical and closed, meaning fluorescein retained within those particles would not be accessible to optical (visible) light, and not included in the fluorescence measurement. Thus the overall deposition efficiency could be a maximum of 4%. These estimates of particle delivery to a well of a six-well plate were used to predict exposure times during the dosing of particles onto cell cultures.
Of the particles that reached the cell surface (), 90% of the particles were within 1 to 5 µm, with a total mean and total median particle size of 3.4 µm and 3.3 µm, respectively. The particle size histograms suggest particles <∼1 µm impacted into the growth medium with low efficiency (). The overall similarity in particle size distribution and numbers of particles observed after 2 and 18 h incubation () indicate that the majority of the particles were fixed upon settling suggesting particle-cell interactions that were lasting.
Particle-cell interactions and cellular responses
Representative images of particles residing on cells are shown in . The particles were observed dispersed across the cell culture 2 hours after particle deposition (). Care was taken to minimize disturbance of the growth medium while moving the six-well plate from the laminar flow hood where particle deposition occurred to the incubator, else particles were observed to deposit in the center of the well. After 18 hours, and post immunocytochemistry processing, the particles on the cell culture were in similar numbers relative to the 2 hour time point, but were frequently observed in clusters of 2 to 5 particles (). The clustering of particles was likely the result of the cells pooling internalized particles. In all of the images collected when using either or
, there was no observable evidence of particle breakdown in the medium or within the cells. The negative control culture displayed negligible ICAM-1 signal (590 nm emission) and images recorded were analogous to that shown in . For the positive control, 100 ng mL−1 of TNF-α, ICAM-1 exhibited stronger 590 nm emission relative to that shown in .
Figure 4. Merged images captured with bright-field microscopy and fluorescent microscopy set to an emission wavelength of 515 nm for an A549 cell culture dosed with (objects that appear small, circular, and white) after (i) 2-hour incubation and (ii) 18-hour incubation and immunocytochemistry work-up. Image (ii) also combines a fluorescent microscopy image at an emission wavelength of 590 nm to illustrate ICAM-1 expression by cells (regions that appear light in gray-scale false color) versus no ICAM-1 expression (regions that appear dark in gray-scale false-color). Subset boxes a, b and c, as well as d, e and f are selected regions of (i), and (ii), respectively. Circles show particle aggregates.

Upon determination of the impaction and wetting efficiency, A549 cultures were dosed with one of two particles types, either or
, and the total particle dose was changed by varying exposure times (1 to 30 min). These exposure periods resulted in particle-cell ratios spanning 0.1 to 2.5. Following immunohistochemical work-up, the images of fluorescence emission from the cultures were used to generate the data plotted in . The relative expression of ICAM-1 in the A549 cultures was not significantly different from the negative control for the range of the average number of
per cell accessed. At doses of
greater than that depicted in , particle clustering in the absence of cells was observed, meaning the size distribution changed in the medium. For that reason, doses at levels of
having particle-to-cell ratios >3, though accessible, were deemed semi-quantitative, and not processed further. Cultures dosed with
up-regulated ICAM-1 expression in A549 cells (). The method of least squares was used to determine the slope ± uncertainty for each data set in . The results for the two particle types (
and
) at room temperature () were 0.009 ± 0.005 and 0.11 ± 0.04, respectively, and at the elevated temperature () were 0.02 ± 0.02 and 0.24 ± 0.03, respectively. For both sets of experiments, the slopes of the regression lines between the two particle types were statistically significantly different (p < 0.01). In addition, the slopes between the
for the two chamber temperatures, i.e. room vs. elevated, were different (p < 0.01).
Figure 5. Normalized ICAM-1 expression after 18 h incubation of A549 cells as a function of dose of either or
with the spray chamber at (a) room temperature and at the (b) nonuniform elevated temperature. The negative control (no dose) and positive control (100 ng mL−1 soluble TNF-α) were normalized to 0 and 1, respectively. Each data point corresponded to a single sample (n = 1). The x- and y-axis propagated uncertainties arose from the no. of images (k = 3) captured per sample at each setting. The number of cells also contributed to the x-axis uncertainty, which was derived from the count of a single culture via a hemacytometer across multiple experiments (n = 4).
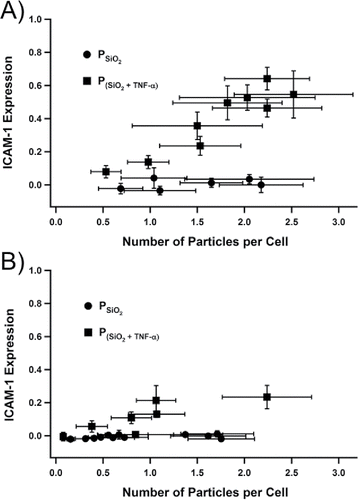
To account for the cytokine response measured following dose by , the liquid-phase interaction between compounds in the starting mixtures was assessed. The resultant ICAM-1 expression levels are listed in . Presented individually to TNF-α in solution/suspension, silica, PEG, and FluoSpheres® increasingly interacted, respectively, with TNF-α, which resulted in corresponding attenuation of ICAM-1 expression. PEG plus FluoSpheres® interacted with TNF-α to the extent that ICAM-1 expression was not different from the negative control (e.g., absent of dose), whereas silica plus PEG resulted in the TNF-α effecting similar ICAM-1 expression as the positive control (e.g., TNF-α only). PEG interacted with silica and FluoSpheres®, to minimize the latters' interactions with TNF-α. Using the information presented in , it was deemed that essentially all of the TNF-α present was bioavailable in the
generated with the spray chamber at room temperature. Note that in nebulizing the starting solutions, and having passed through the spray chamber at the nuET, the resultant particles were dry and aggregated. This observation suggested these compounds interacted nonreversibly under these conditions.
Table 1. ICAM-1 expression in A549 cultures, presented as fold change relative to the negative control, for 18 h incubation periods with the soluble and/or dispersed reagents used in the starting mixtures to create silica particle types.
To further investigate the measured cytokine response following dosage with , the bioavailable TNF-α from
was estimated, as described in the Experimental section. In parallel, A549 cells were incubated with known concentrations of soluble TNF-α to generate a range of ICAM-1 responses that bracketed the sample ICAM-1 responses induced by
. The data indicated that an estimated 10 ± 3% of the TNF-α was active and bioavailable. The remaining 90% of TNF-α, was nonactive via heat denaturation during the brief passage through the elevated temperature spray chamber. An additional possibility for the reduced efficacy of the TNF-α that it was physically bound to the silica particle aggregate.
The data presented in illustrate that for the condition of delivering up to ∼2.5 particles per cell, the expression of ICAM-1 by A549 cells in response to doses of was not different from the negative control. However, silica has been reported to induce cell apoptosis and cellular expression using A549 monocultures. For instance, Wottrich and co-workers measured the relative lethality of a standard, micron-size, quartz particle type, DQ12, relative to amorphous SiO2 nanoparticles of 60 and 100 nm diameter (Wottrich et al. Citation2004). DQ12 particles were more cytotoxic compared to both amorphous SiO2 nanoparticles. The measured lethality for DQ12 particles was 2, 8, 22, and 37% for A549 cells at doses of 10, 50, 100, and 200 µg ml−1, respectively. These values correspond to equivalent surface coverage doses of ∼6.1, 30.3, 60.6 and 121 µm cm−2, respectively. In a study by Singh and co-workers, a 16 µg cm−2 dose of DQ12 did not up-regulate IL-8 in A549 cultures, whereas a higher dose of 80 µg cm−2 caused increased expression (Singh et al. Citation2007). Lin and co-workers used amorphous SiO2 nanoparticles with median diameters of 15 and 46 nm, and standard micron-size quartz particle type, Min-U-Sil 5 (Lin et al. Citation2006). The cell viability of A549 cells exposed to Min-U-Sil 5 particles, at concentrations of 10, 50, and 100 µg mL−1 was 95.1, 85.2, and 81.0%, respectively. The Min-U-Sil 5 concentrations of 10, 50 and 100 µg mL−1 corresponded to an estimated cell surface coverage of 5.2, 26, and 52 µg cm−2 respectively. Hetland and co-workers used amorphous SiO2 nanoparticles and size fractionated quartz particles from another standard quartz particle type, Norquartz-45, at mean diameters of 10, 2, and 0.5 µm (Hetland et al. Citation2001). Statistical significance was not reported, but the expression for the 2 µm quartz particles deviated from the control at an estimated 30 µg cm−2 and 10 µg cm−2 for IL-6 and IL-8, respectively. At the highest dose of 100 µg cm−2, IL-6 and IL-8 expression increased 5.5- and 3.1-fold relative to the control, respectively.
In this work, total particle deposition efficiency was measured as 2.0 ± 0.4%, which corresponded to a maximum estimated dose (i.e. 30 min. exposure period) of 56 ± 10 µg, 28 ± 5 µg mL−1, or 6 ± 1 µg cm−2. The experimental apparatus and methodology used in this report was different from the aforementioned studies in two ways. The dosage of micron-size amorphous silica nanoparticle aggregates (this work) versus amorphous nanoparticles or micron-size crystalline particles, and that the dosage values in this work were at the lower end of mass coverage relative to the referenced studies. As described above, a mass coverage of 6.1 µg cm−2 in the Wottrich study and 5.2 µg cm−2 in the Lin study did not significantly decrease cell viability. Although the required silica concentration to induce ICAM-1 response from A549 monocultures is lacking in literature, other pro-inflammatory proteins, namely IL-6 and IL-8, have been explored extensively. As shown in the studies by Hetland and Singh, it appeared these cytokines were not up-regulated until a dose of approximately 10 to 30 µg cm−2 was applied onto cell cultures. It is therefore not surprising that differential expression for both IL-6 and IL-8 from A549 cells in this work was not observed upon the delivery of 5.9 µg cm−2 (). In using the methodology reported herein, obtaining a surface coverage higher than 5.9 µg cm−2 could be achieved, but aggregation of particles, even in the absence of cell interactivity, increased the difficulty, and error, in obtaining an accurate particle count.
Figure 6. Data for cytokine expression from two experiments, each utilizing a different cell culture composition, involving the deposition of silica particles, . (a) IL-6 and (b) IL-8 expression after 18-hour incubation of A549 cell cultures or A549 + THP-1* co-cultures grown to a 15:1 ratio and dosed with silica particles.
as described in the experimental section. Starting solution uptake rate was 15 µL min−1,
exposure time to each cell culture was 10 min, with particle-to-cell ratios of 1.90 ± 0.08 and 0.99 ± 0.26 for the monocultures and the co-cultures, respectively, and 10 ng mL−1 TNF-α represents the positive control in which the TNF-α was introduced in soluble form. Single and double number signs (# and ##) indicate samples statistically different (p < 0.01) than the control cultures for A459 cells and A549 + THP-1* cells, respectively; where n = 3 for control cultures and TNF-α samples, and n = 6 for silica samples.
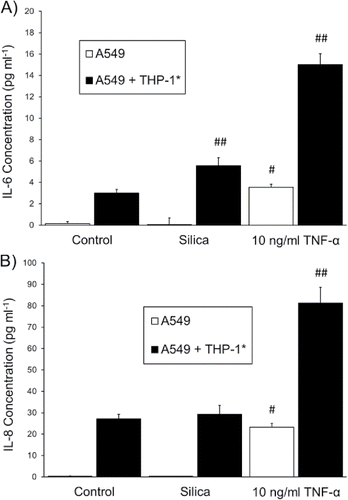
The limited A549 response following incubation with prompted the use of co-cultures as a means to increase cellular response and method sensitivity while maintaining an accurate particle count. Co-cultures of A549 and THP-1* cells were prepared, and to expand the readout of cellular response assessment, cytokines IL-6 and IL-8 were included in assessing culture response () following dosage and incubation with
. The positive control for both culture types in was soluble TNF-α. IL-6 levels were significantly greater from co-cultures exposed to
, whereas the expression of IL-8 was not distinguishable from the negative control. ICAM-1 response from the co-cultures was assessed, but it was found that ICAM-1 was elevated to the extent that a measurable difference between the negative and positive controls was too small to be quantitatively useful for these experiments.
Conclusions
A particle generation apparatus and associated methodology were developed and used to perform multi-compositional particle dose–response studies using human lung mono- and co-cultures. The apparatus readily affords particle doses at the level of 0.1 to 2.5 particles cell−1 over an exposure time spanning 1 to 10 min, but up to 30 min was demonstrated. The potential utility of the method was illustrated with a multi-component particle type. This particle type incorporated the compound TNF-α directly into the particle, and as such, provided a method control. Particles generated with the spray chamber at room temperature did not remain intact in the growth medium and the cellular response as a function of the calculated dose delivered as
indicated that all TNF-α delivered was bioavailable. Conversely, for particles that were generated with the spray chamber at elevated temperature, which remained intact in the growth medium, ∼90% of the TNF-α delivered to the cell culture was denatured, and additionally, possibly bound to particles.
Acknowledgments
We are thankful to Dr. Geoff Coleman for discussion and nebulizer donation, Meinhard Inc. CO, USA, I.-H. Sherry Lu and Haley Mitchell for preliminary work, and 4D labs for electron microscopy.
References
- Adamson, I. Y., Prieditis, H., and Vincent, R. (1999). Pulmonary Toxicity of an Atmospheric Particulate Sample is due to the Soluble Fraction. Toxicol. Appl. Pharmacol., 157:43–50.
- Akhtar, U. S., Rastogi, N., McWhinney, R. D., Urch, B., Chow, C.-W., Evans, G. J., and Scott, J. A. (2014). The Combined Effects of Physicochemical Properties of Size-Fractionated Ambient Particulate Matter on in Vitro Toxicity in Human A549 Lung Epithelial Cells. Toxicol. Rep., 1:145–156.
- Alfaro-Moreno, E., Ponce-de-Leon, S., Osornio-Vargas, A. R., Garcia-Cuellar, C., Martinez, L., and Rosas, I. (2007). Potential Toxic Effects Associated to Metals and Endotoxin Present in PM10: An Ancillary Study Using Multivariate Analysis. Inhal.Toxicol., 19(Suppl. 1):49–53.
- Bakand, S., Winder, C., Khalil, C., and Hayes, A. (2005). Toxicity Assessment of Industrial Chemicals and Airborne Contaminants: Transition from in Vivo to in Vitro Test Methods: A Review. Inhal.Toxicol., 17:775–787.
- Berube, K., Aufderheide, M., Breheney, D. et al. (2009). In Vitro Models of Inhalation Toxicity and Disease. ATLA, 37:89–141.
- Bitterle, E., Karg, E., Schroeppel, A., Kreyling, W. G., Tippe, A., Ferron, G. A., Schmid, O., Maier, K. L., and Hofer, T. (2006). Dose-Response Exposure of A549 Epithelial Cells at the Air-Liquid Interface to Airborne Ultrafine Carbonaceous Particles. Chemosphere, 65:1784–1790.
- Brobell, D., Troller, S., Dziurowitz, N., Plitzko, S., Linsel, G., Asbach, C., Azong-Wara, N., Fissan, H., and Schmidt-Ott, A. (2013). A Thermal Precipitator for the Deposition of Airborne Nanoparticles Onto Living Cells-Rationale and Development. J. Aersol Sci., 63:75–86.
- Brodie, E. L., DeSantis, T. Z., Moberg Parker, J. P., Zubietta, I. X., Piceno, Y. M., and Andersen, G. L. (2007). Urban Aerosols Harbor Diverse and Dynamic Bacterial Populations. Proc. Natl. Acad. Sci., USA, 104:299–304.
- Boumans, P. W. J. M. (1987). Inductively Coupled Plasma Emission Spectroscopy. Part II: Applications and Fundamentals, Volume 2, John Wiley and Sons, New York, p. 6743257.
- Clague, A. D. H., Donnet, J. B., Wang, T. K., and Peng, J. C. M. (1999). A Comparison of Diesel Engine Soot with Carbon Black. Carbon, 37:1553–1565.
- Dagher, Z., Garcon, G., Gosset, P., Ledoux, F., Surpateanu, G., Courcot, D., Aboukais, A., Puskaric, E., and Shirali, P. (2005). Pro-Inflammatory Effects of Dunkerque City Air Pollution Particulate Matter 2.5 in Human Epithelial Lung Cells (L132) in Culture. J. Appl. Toxicol., 25:166–175.
- Elder, A., Gelein, R., Finkelstein, J., Phipps, R., Frampton, M., Utell, M., Kittelson, D. B., Watts, W. F., Hopke, P., Jeong, C.-H., Kim, E., Liu, W., Zhao, W., Vincent, R., Kumarathasan, P., and Oberdorster, G. (2004). On-Road Exposure to Aerosols 2. Exposures of Aged Compromised Rats. Inhal. Toxicol., 16:41–53.
- Farina, F., Sancini, G., Battaglia, C., Tinaglia, V., Mantecca, P., Camatini, M., and Palestini, P. (2013). Milano Summer Particulate Matter (PM10) Triggers Lung Inflammation and Extra Pulmonary Adverse Events in Mice. PloS One, 8:e56636.
- Ghio, A. J., Stonehuerner, J., Dailey, L. A., and Carter, J. D. (1999). Metals Associated with Both the Water-Soluble and Insoluble Fractions of an Ambient Air Pollution Particle Catalyze an Oxidative Stress. Inhal. Toxicol., 11:37–49.
- Grass, R. N., Limbach, L. K., Athanassiou, E. K., and Stark, W. J. (2010). Exposure of Aerosols and Nanoparticle Dispersions to in Vitro Cell Cultures: A Review on the Dose Relevance of Size, Mass, Surface and Concentration. J. Aerosol Sci., 41:1123–1142.
- Haddrell, A. E., Ishii, H., van Eeden, S. F., and Agnes, G. R. (2005). Apparatus for Preparing Mimics of Suspended Particles in the Troposphere and Their Controlled Deposition Onto Individual Lung Cells in Culture with Measurement of Downstream Biological Response. Anal. Chem., 77:3623–3628.
- Hetland, R. B., Schwarze, P. E., Johansen, B. V., Myran, T., Uthus, N., and Refsnes, M. (2001). Silica-Induced Cytokine Release from A549 Cells: Importance of Surface Area Versus Size. Hum. Exp. Toxicol., 20:46–55.
- IARC Working Group on the Evaluation of Carcinogenic Risks to Humans Silica, some Silicates, Coal Dust, and Para-Aramid Fibrils (n.d.). Monogr. Eval. Carcinog. Risks Hum., 68:1–475.
- Imrich, A., Ning, Y. Y., and Kobzik, L. (2000). Insoluble Components of Concentrated Air Particles Mediate Alveolar Macrophage Responses in Vitro. Toxicol. Appl. Pharmacol., 167:140–150.
- Jalava, P., Salonen, R. O., Halinen, A. I., Sillanpaa, M., Sadell, E., and Hirvonen, M.-R. (2005). Effects of Sample Preparation on Chemistry, Cytotoxicity, and Inflammatory Responses Induced by Air Particulate Matter. Inhal.Toxicol., 17:107–117.
- Kaan, P. M., and Hegele, R. G. (2003). Interaction Between Respiratory Syncytial Virus and Particulate Matter in Guinea Pig Alveolar Macrophages. Am. J. Respir. Cell Mol. Biol., 28:697–704.
- Lee, S. Y., Gradon, L., Janeczko, S., Iskandar, F., and Okuyama, K. (2016). Formation of Highly Ordered Nanosctructures by Drying Micrometer Colloidal Droplets. ACS Nano, 4:4717–4724.
- Lenz, A. G., Karg, E., Lentner, B., Dittrich, V., Brandenberger, C., Rothen-Rutishauser, B., Schulz, H., Ferron, G. A., and Schmid, O. (2009). A Dose-Controlled System for Air-Liquid Interface Cell Exposure and Application to Zinc Oxide Nanoparticles. Part. Fibre Toxicol., 6:1–17.
- Leung, C. C., Ignatius, T. S. Y., and Chen, W. (2012). Silicosis. Lancet, 379:2008–2018.
- Lin, W., Huang, Y. W., Zhou, X. D., and Ma, Y. (2006). In Vitro Toxicity of Silica Nanoparticles in Human Lung Cancer Cells. Toxicol. Appl. Pharmacol., 217:252–259.
- Longerich, H. P. (1993). Automated Two-Speed Peristaltic Pump Controller for Reduced Sample Wash-out Time and Analysis of Small Sample Volumes. J. Anal. Atomic Spectrom., 8:371–374.
- Mauderley, J. L., and Samet, J. M. (2009). Is There Evidence for Synergy Among Air Pollutants in Causing Health Effects? Environ. Health Perspect., 117:1–6.
- Motl, N. E., Mann, A. K. P., and Skrabalak, S. E. (2013). Aerosol-Assisted Synthesis and Assembly of Nanoscale Building Blocks. J. Mater. Chem. A., 1:5193–5202.
- Naimabadi, A., Ghadiri, A., Idani, E., Babaei, A. A., Alavi, N., Shirmardi, M., Khodadadi, A., Marzouni, M. B., Ankali, K. A., Rouhizadeh, A., and Goudarzi, G. (2016). Chemical Composition of PM and Its in Vitro Toxicological Impacts on Lung Cells During the Middle Eastern Dust (MED) Storms in Ahvaz, Iran. Environ. Pollut., 211:316–324.
- Occupational Safety and Health Administration (2016). Occupational exposure to respirable crystalline silica. 81-FR-16285.
- Puledda, S., Paoletti, L., and Ferdinandi, M. (1999). Airborne Quartz Concentration in An Urban Site. Environ. Pollut., 104:441–448.
- Pulskamp, K., Diabate, S., and Krug, H. F. (2007). Carbon Nanotubes Show no Sign of Acute Toxicity But Induce Intracellular Reactive Oxygen Species in Dependence on Contaminants. Toxicol. Lett., 168:58–74.
- Sánchez, R., Todolí, J. L., Lienemann, C.-P., and Mermet, J.-M. (2010). Air-Segmented, 5-μL Flow Injection Associated with a 200 °C Heated Chamber to Minimize Plasma Loading Limitations and Difference of Behaviour Between Alkanes, Aromatic Compounds and Petroleum Products in Inductively Coupled Plasma Atomic Emission Spectrometry. J. Anal. Atomic Spectrom., 25:1888–1894.
- Scheller, J., Chalaris, A., Schmidt-Arras, D., and Rose-John, S. (2011). The Pro-and Anti-Inflammatory Properties of the Cytokine Interleukin-6. Biochim.Biophys.Acta., 1813:878–888.
- Schulz, C., Farkas, L., Wolf, K., Kratzel, K., Eissner, G., and Pfeifer, M. (2002). Differences in LPS-Induced Activation of Bronchial Epithelial Cells (BEAS-2B) and Type II-Like Pneumocytes (A-549). Scand. J. Immunol., 56:294–302.
- Shiraki, R., and Holmen, B. A. (2002). Airborne Respirable Silica near a Sand and Gravel Facility in Central California: XRD and Elemental Analysis To Distinguish Source and Background Quartz. Environ. Sci. Technol., 36:4956–4961.
- Sillanpää, M., Geller, M. D., Phuleria, H. C., and Sioutas, C. (2008). High Collection Efficiency Electrostatic Precipitator for in Vitro Cell Exposure to Concentrated Ambient Particulate Matter (PM). J. Aerosol Sci., 39:335–347.
- Singh, S., Shi, T., Duffin, R., Albrecht, C., van Berlo, D., Hohr, D., Fubini, B., Martra, G., Fenoglio, I., Borm, P. J., and Schins, R. P. (2007). Endocytosis, Oxidative Stress and IL-8 Expression in Human Lung Epithelial Cells Upon Treatment with Fine and Ultrafine TiO2: Role of the Specific Surface Area and of Surface Methylation of the Particles. Toxicol. Appl. Pharmacol., 222:141–151.
- Sokolov, S. V., Tschulik, K., Batchelor-McAuley, C., Jurkschat, K., and Compton, R. G. (2015). Reversible or not? Distinguishing Agglomeration and Aggregation at the Nanoscale. Anal. Chem., 87:10033–10039.
- Soukup, J. M., Ghio, A. J., and Becker, S. (2000). Soluble Components of Utah Valley Particulate Pollution Alter Alveolar Macrophage Function in Vivo and in Vitro. Inhal.Toxicol., 12:401–414.
- Suwa, T., Hogg, J. C., Quinlan, K. B., Ohgami, A., Vincent, R., and van Eeden, S. F. (2002). Particulate Air Pollution Induces Progression of Atherosclerosis. J. Am. Coll. Cardiol., 39:935–942.
- Tang, J. W. (2009). The Effect of Environmental Parameters in the Survival of Airborne Infectious Agents. J. R. Soc. Interface., 6:S737–S746.
- Tao, F., and Kobzik, L. (2002). Lung Macrophage-Epithelial Cell Interactions Amplify Particle-Mediated Cytokine Release. Am. J. Respir. Cell Mol. Biol., 26:499–505.
- U.S. EPA (1996). Health Effects of Inhaled Crystalline and Amorphous Silica. U.S. Environmental Protection Agency, Office of Research and Development, National Center For Environmental Assessment, Research Triangle Park Office, Research Triangle Park, NC, EPA/600/R-95/115.
- van de Stolpe, A., and van der Saag, P. T. (1996). Intercellular adhesion molecule-1. J. Mol. Med., 74:13–33.
- Velali, E., Papachristou, E., Pantazaki, A., Choli-Papadopoulou, T., Planou, S., Kouras, A., Manoli, E., Besis, A., Voutsa, D., and Samara, C. (2016). Redox Activity and in Vitro Bioactivity of the Water-Soluble Fraction of Urban Particulate Matter in Relation to Particle Size and Chemical Composition. Environ. Pollut., 208:774–786.
- Watson, A. Y., and Valberg, P. A. (2001). Carbon Black and Soot: Two Different Substances. J. Am. Ind. Hy. Assoc., 62:218–228.
- Wottrich, R., Diabate, S., and Krug, H. F. (2004). Biological Effects of Ultrafine Model Particles in Human Macrophages and Epithelial Cells in Mono-and Co-Culture. Int. J. Hyg. Environ. Health, 207:353–361.
- Zosky, G. R., Iosifidis, T., Perks, K., Ditcham, W. G. F., Devadason, S. G., Siah, S. S., Devine, B., Maley, F., and Cook, A. (2014). The Concentration of Iron in Real-World Geogenic PM10 Is Associated with Increased Inflammation and Deficits in Lung Function in Mice. PloS one, 9:1–9.