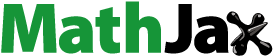
Abstract
The objective of this study was to analyze the deposition patterns of aerosolized bacteria, fungi, and fluorescent particles with a lab-made, multiple round nozzle, single-stage inertial impactor. The cutoff diameter (d50), experimental d50, and Reynolds number of the impactor were 0.39 µm, 0.80 µm, and 2825, respectively. The impactor was elaborated based on a classical impactor design criterion. The deposition characteristics, namely the size, shape, and collection efficiency of aerosols, differed significantly for impaction surfaces like smooth silicon and nanostructured silicon (i.e., black silicon) and various sizes of particles. Our experiment results, based on the image analysis method, suggest that the impaction characteristics of aerosols are subject to a rebound and re-entrainment (i.e., bounce effect) phenomenon that is tuned by the size of the particles, regardless of their origin. This finding leads us to distinguish the collection of global (classical) and local efficiencies, based on where the particles are deposited on the impaction plate. The particles in the primary impaction zone, located underneath the nozzle to which a Micro-Electro-Mechanical System (MEMS) is aligned, are considered to evaluate the local collection efficiency. We estimated the local collection efficiency from the collection on a smooth silicon surface as 17.9 ± 1.8% for fluorescent particles with an aerodynamic diameter (dae) of 0.92 µm and <1% for particles with dae of 2.07, 2.53, and 3.83 µm in size. The reduced number of particles in the primary impaction zone as their size increases is evidence of the bounce effect. This observation is also confirmed for the first time with the capturing of bioaerosols. For the elimination of the bounce effect and localization of the deposit in the primary zone, this empirical study proposes the solution of tailoring the smooth silicon surface with sharp pillar nanostructures (e.g., black silicon, BSi). Therefore, we show that a BSi-functionalized MEMS mass sensor–integrated impactor is suitable for the detection of micron-sized solid particles, such as Aspergillus niger spores (dae of 3.04 µm).
Copyright © 2021 American Association for Aerosol Research
EDITOR:
1. Introduction
The widely known problem with the inertial impactors is that solid airborne particles bounce off the impaction plate and create a spread of the captured particles on the plate. This particle bounce phenomenon results in a distortion of the size and mass concentration measurements, and it is usually minimized by coating the plate with adhesive materials.
This issue is nontrivial in the new generation of miniaturized inertial impactors to which Micro-Electro-Mechanical System (MEMS) mass sensors are coupled. The aim of the integration is to provide real-time, highly sensitive mass concentration measurements of aerosols (Soysal et al. Citation2017). The integration of a small-scale MEMS mass sensor as a micro impaction plate requires a perfect alignment between the device and the nozzle to avoid compromising the efficiency of the collection and to provide unbiased size and mass concentration measurements. Thus, the deposition characteristics of the captured aerosols due to the bounce effect, such as their size, shape, and collection efficiency, are of importance for small-scale miniature systems. The deposition properties of aerosols in classical systems are well documented in the scientific literature. In particular, the deposit shapes, such as halos, rings, and disks (i.e., the primary deposits), come to the forefront as the most encountered ones (). For example, (May Citation1975) stated that the reason for halo-shaped deposition patterns for micron size test particles (d50 of 3.5–13 µm) is due to the hydrodynamic or Magnus effects when the Reynolds number is higher than 2000 or when the jet velocities exceed 60 m/s at a low Reynolds number.
Willeke and Pavlik Citation1979 reported that the ring- and halo-shaped deposits are due to vortex formations of test particles (d50 of ∼2.6 µm) at Reynolds number of 3140 (Oodo et al. Citation1981) documented that the halo shape forms when Reynolds number is higher than 2000 for micron-sized test particles (dae of 1.10 µm and 2.02 µm). In the same study, when the impaction plate was coated with silicone grease, no halo was observed even at high Reynolds numbers. The reason for the halo was therefore correlated with the rebound and re-entrainment of the particles.
Another study (Sethi and John Citation1993) reported that the ring-shaped deposit forms when the Stokes number is 0.47 with test particles of 3.0 µm dae. An increase in the Stokes number led to the formation only of primary deposits with diameters that could be twice as small as the outer diameter of the ring deposits. (Michaud et al. Citation1999) observed halo-shaped deposits in the lower stages of their impactor and d50 changes from (2.0 ± 0.1) µm to (0.07 ± 0.01) µm, and they stated that the halo was produced by jet turbulence. (Rocklage et al. Citation2013) documented that the impact of micron-sized test particles (dae of 1.3–6.3 µm) resulted in a halo-shaped deposit only at low Reynolds numbers and disappeared at Reynolds numbers above 1000.
The reason for halo-shaped deposits was also explained by sedimentation of the large particles, which formed ring deposits closer to the primary deposit due to an increased effect of gravity on large particles. Recently, (Fredericks and Saylor Citation2018) investigated the ring-shaped impact patterns of test particles (dae of 3–15 µm) in a customized impactor and reported that the occurrence of the ring-shaped deposits was related to the inertia of the particles. Hence, the internal diameter and thickness of the rings were shown to be a function of the particle diameter. Finally, (García-Ruiz et al. Citation2019) associated the halo formation of test particles (dae of 1.5–6.0 µm) with inertia and gravity. In their study, liquid particles were used to eliminate the bounce and re-suspension effects.
In a MEMS-based impactor system, the efficient capturing of particles requires that the deposit formed on the surface under the nozzle be smaller than the device. This implies that the deposition should occur only in the primary impact zone. Therefore, the occurrence of the halo- and ring-shaped deposits in miniaturized systems can be a bottleneck. To date, this problem has not received attention in relevant studies (Soysal et al. 2017).
The goal of this research is to build a silicon-based MEMS-integrated impactor detection system for real-time mass concentration measurements. This study is the first to show that the impaction of aerosols on a smooth silicon surface is significantly influenced by the bounce effect. Hence, the particle size was changed to tune this effect. Ultimately, the particle size–dependent bounce effect led to a local collection efficiency, which defines the correct capturing efficiency for a microsensor. To minimize the bounce effect and localize the deposit under the nozzle, we propose to impact micron-sized particles onto sharp nanopillars. Our experimental results showed that the pillars broke the particles into fragments by impaling them, leading to a well-defined primary impaction spot. In this study, the effect of the sharp pillars was studied for different sizes and types of aerosols and bioaerosols.
2. Materials and methods
2.1. Design and elaboration of the impactor
Since the first proposal of the concept of the inertial cascade impaction by (May Citation1945), impactors have been widely studied, used, and characterized for over 50 years. In this study, a single-stage multiple nozzle inertial impactor was designed, and it is described in detail in the online supplementary information (SI). Briefly, the impactor consists of a nozzle plate with 10 round nozzles with tapered inlets positioned circularly. The impaction chamber has a 1000 µm separation between the nozzles and the impaction plate (holder). Silicon and black silicon micro impaction plates are placed in the chamber to simulate the silicon-based MEMS mass sensor-integrated impaction conditions. Its calculated d50 is 0.39 µm and the Re is kept below 3000. Each of the 10 nozzles delivers 1 Liter per Minute (LPM) to their corresponding 10 surfaces (i.e., the 10 MEMS sensors for multi-detection). The active surface area of the sensors is 1 mm2, for reasons described elsewhere (Soysal et al., Citation2020). The silicon impaction plates (thickness of 500 ± 25 µm) were diced from p-type silicon wafers (Addison Engineering, Inc. San Jose, CA). This new-generation impactor enables the sampling of aerosols and bioaerosols at 10 LPM without damaging the MEMS sensors due to the divided flow. It also allows the simultaneous investigation of 10 different impaction spots.
2.2. Black silicon as an impaction plate
Current microfabrication technology is capable of fabricating specific particle attachment zones on the impaction surface by processing the silicon surface (). In this article, BSi was exploited as an impaction plate with the aim of reducing the bounce effect and of localizing the captured particles to the primary impaction spot. BSi consists of nanopillars with diameters of a few nm and length of ∼3 µm and was fabricated using an O2–SF6 plasma-based (∼−110 °C) cryogenic deep-reactive ion etching (DRIE) method. A detailed description can be found elsewhere (Nguyen et al. Citation2012).
2.3. Monodisperse airborne particle generation
The instrumental setup for the generation of airborne particles, both for aerosol and bioaerosols, contains several systems. All aspects of the instruments are illustrated and detailed in the SI. Briefly, a vibrating orifice aerosol generator (VOAG model 3450, TSI) was used to generate monodisperse solid fluorescent particles with a mean median aerodynamic diameter ( of (0.92 ± 0.02) µm, (2.07 ± 0.03) µm, (2.53 ± 0.05) µm, and (3.83 ± 0.01) µm (see SI S2.3.1). Staphylococcus epidermidis and Pseudomonas fluorescens bacteria were aerosolized with a collision nebulizer (BGI, MA, USA). The (
values for P. fluorescens and S. epidermidis were (0.66 ± 0.01) µm and (0.70 ± 0.02) µm, respectively (see in SI S2.3.2). Lastly, a (
of (3.04 ± 0.05) µm was obtained for Aspergillus niger spores aerosolized with a dry generation method (see in SI S2.3.3). All types of generated particles were sampled with the impactor mentioned above on silicon and BSi surfaces, and their size distribution measured with an aerodynamic particle size spectrometer (APS, TSI model 3321) (see SI S2.3.4).
3. Results
3.1. Characterization of the impactor
The details of the experimental setup and the measurements showing the global collection efficiency curve of the impactor are available in SI S3.1. The empirical cutoff diameter of the impactor was 0.80 µm, while the global collection efficiency of the impactor was (62.8 ± 3.2)% for particles with a dae of 1 µm. The poor adhesion properties of this flat non-treated surface enabled us to investigate the bounce mechanism. Likewise, an impactor with this global collection efficiency is appropriate for airborne particle sampling.
3.2. Deposition characteristics of the particles on Si and BSi
The impaction of monodisperse particles onto Si and BSi surfaces showed different deposition characteristics, which allowed us to distinguish local collection efficiency from global collection efficiency. Our image analysis method revealed that the deposition patterns of aerosol and bioaerosols were in good agreement with each other. The patterns (i.e., the halo-shaped, ring-shaped, or primary deposits) were in tune with the size of the captured particles (see ). Therefore, this result strongly indicates that all the particles are forcefully subjected to the bounce-effect phenomenon, regardless of their different surface properties.
3.2.1. Deposition characteristics of monodisperse fluorescent particles on Si and BSi
The impaction on the smooth Si showed that the number of particles in the primary impaction zone decreased as the size increased from a dae of 0.92 µm to micron sizes. As a result, the shape of the deposits gradually changed from the halo to the ring. For the nanostructured BSi, the particles with dae of 0.92 µm size formed a halo. Still, the impaction of 2.07 µm, 2.53 µm, and 3.83 µm particles resulted in the formation only of the primary impaction zone, not a ring shape, as opposed to the impaction of these particles on Si (more details available in SI S3.2.1). These primary impaction zones on BSi are shown in the SEM images in . The destroyed particles are due to their impaction on the sharp nanostructured pillars. Thus, the impaction of 2.07 µm, 2.53 µm, and 3.83 µm particles onto the pillars breaks them and results in a localized circular deposition pattern (diameter <500µm) (see ).
Figure 3. SEM of the deposition characteristics of fluorescent particles of different sizes on Si and BSi surfaces.
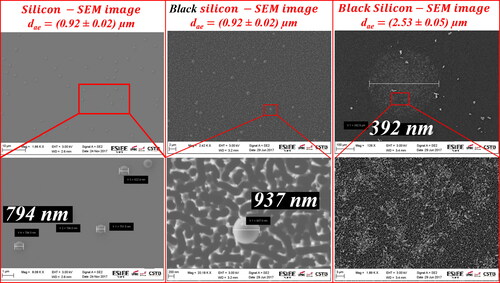
The local collection efficiency of the particles on the smooth Si was estimated from the primary impaction zone under the nozzle, from a 0.75 mm2 area, as (17.9 ± 1.8)% for dae of 0.92 µm particles. For the particles with dae of 2.07, 2.53, and 3.83 µm, the local collection efficiency results in an efficiency of <1% (see ). The local collection efficiency was estimated from the 0.75 mm2 area because the active area of the microsensor is 1 mm2, for the reasons explained elsewhere (Soysal et al. Citation2020). The local collection efficiency cannot be estimated by counting the particles on the primary impaction zone of BSi due to the destroyed particles.
3.2.2. Deposition characteristics of aerosolized bacteria on Si and BSi
Our various experiments revealed that the bacteria are localized and concentrated in the primary deposition area (diameter <500 µm) and are encircled by a dispersed ring that constitutes a halo-shaped deposit on the Si and BSi surfaces. Note that the aggregation of bacterial cells on Si and BSi makes them difficult to count, and the collection efficiency could not be estimated in this case.
3.2.3. Deposition characteristics of aerosolized fungi on Si and BSi
When the Aspergillus niger spores (dae of 3.04 µm) were sampled on the smooth Si surface, fragments of the fungi were observed in the primary impaction zone, unlike the case for the dae of 2.07 µm, 2.53 µm, and 3.83 µm fluorescent particles that formed a particle-free area under the nozzle and led to the ring shape deposit. However, spores were found outside the primary impaction zone, as they would bounce off the central impaction zone, and these spores might leave some fragments or escape from the impaction and be dispersed on the surface until the edge by sedimentation. shows the impaction of Aspergillus niger spores on the BSi surface. As is evident, the sharp pillars lead to a localized circular deposition pattern (diameter <500 µm) by breaking the spores into fragments and, most probably, by impaling them. The spores were captured in the primary impaction zone of the BSi surface, unlike the spores that were not found in the central impaction zone on the Si surface.
4. Discussion
Particles are well known to rebound when they strike flat or non-treated impaction surfaces. They may also hit other previously collected particles, detaching those from the surface and forcing them to follow the airstream in a phenomenon referred to as re-entrainment (see ). In both cases, the particles can bounce off either from the primary impaction zone or from the impaction plate. A simplified theory of the mechanism was adapted from (Paw U Citation1983; Dahneke Citation1971) and is shown in . When a particle that is accelerated along the nozzle throat exits the nozzle, its initial kinetic energy comprises the particle mass and its initial velocity and can be represented as The particle then falls into the initial particle-surface potential energy well (
by getting closer to the surface. If the kinetic energy of the particle before impact (
is sufficient to overcome the energy loss due to the collision
then the particle can escape the surface with the rebound energy of
Otherwise, the particle will be captured by the surface.
originates from the following: elastic and plastic deformation, internal friction, radiation of acoustic waves (such as shears, compressive and Rayleigh surface waves), and flexural work (for the thin and flexible surfaces) (Dahneke Citation1971). The final kinetic energy of the particle remains as
by climbing the final potential energy well, which reaches
The potential energy wells are the function of the adhesion force field between the particle and the surface, which is created by Van der Waals forces.
Figure 6. Schematic illustrations of rebound and re-entrainment theory. (Adapted from Paw U Citation1983.)
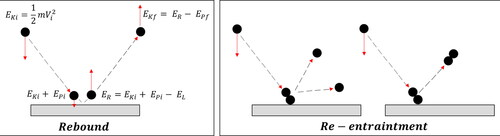
The energy dissipation that originated from the compressed air layer squeezed between the particle and the surface is suggested to be negligible, either in a relative vacuum or in the air (Paw U Citation1983). Additionally, the incoming particles may collide with a previously collected particle on the surface (see ). After this collision, the impacted particle can be detached from the surface. Then, both particles can either rebound separately (in the case of large particles, due to their high aerodynamic drag force) or become attached. The latter occurs by the rolling, sliding, or direct liftoff of the particles. Finally, the detached particle follows the air stream. Thus, the particles practice re-entrainment. Re-entrainment can also occur due to the turbulence created by the high velocity near the surface, which may overcome the adhesion force (Ibrahim et al. Citation2003; Ziskind et al. Citation1995). Both rebound and re-entrainment are interactive mechanisms; thus, assessing them separately is difficult. Therefore, both mechanisms are usually referred to as the bounce effect.
In this study, as summarized in , increasing the size of the fluorescent particles dramatically decreases the number of collected particles in the primary impaction zone because the large particles bounced off the primary zone and formed the ring deposit. Although a small number of large fluorescent particles (e.g., dae of 2.53 µm in ) were also collected in the primary zone, they can be subjected to re-entrainment by the following large particles due to the high drag force. The increase in the particle size leads to an increase in the initial kinetic energy, which is more likely to overcome the energy loss due to the collision,
Table 1. A summary of the deposition characteristics of the particles.
In the case of Aspergillus niger, with dae of 3.04 µm, was also sufficient to overcome
however, due to the different surface morphology and adhesion properties of the spores compared to the fluorescent particles, only fragments of the Aspergillus niger particles were found in the primary impaction zone as a result of the bounce.
The decrease in the fluorescent particle size down to sub-micron levels (e.g., dae of 0.92 µm) leads to a dramatic increase in the number of collected particles in the primary impaction zone (see ). Thus, fluorescent particles with dae of 0.92 µm exhibited a relatively uniform deposition pattern in the primary impaction zone. This observation is consistent with the impaction results for the sub-micron sized aerosolized bacteria, Pseudomonas fluorescens (dae of 0.66 µm) and Staphylococcus epidermidis (dae of 0.70 µm), despite the differences in particle properties.
The decrease in the particle size leads to a decrease in the which is presumably insufficient for the small particle to tackle the
Thus, unlike the other micron-sized particles, the sub-micron particles did not escape as efficiently from the
which is characterized by Van der Waals forces. One point to mention is that, statistically, the fluorescent particles with dae of 0.92 µm and both bacteria were deposited everywhere on the collection surface but at different concentrations, which enabled us to distinguish the deposit shape. When the re-entrainment mechanism plays a dominant role over the rebound mechanism due to the competition between the
of the incoming particle and Van der Waals force subjected to previously attached particles, the halo is formed on the smooth silicon. In addition, some portion of these particles can escape from the impaction or can rebound, but they eventually sediment and contribute to the halo.
Conversely, the sharp pillars of the BSi surface jeopardized the bounce effect by destroying the fluorescent particles with dae of (2.07 ± 0.03) µm, (2.53 ± 0.05) µm, and (3.83 ± 0.01) µm and the Aspergillus niger spores with dae of 3.04 µm. In those cases, the destroyed particles only concentrated on the primary impaction zone, while the rebound mechanism was avoided entirely by the BSi. All the other particles with dae smaller than the abovementioned sizes formed halo-shaped deposit patterns on the BSi due to their insufficient
5. Conclusion
No simple, low-cost, portable, easily integrated (i.e., into daily use electronics for ready-to-use pocket sensors), and real-time aerosol mass concentration measurement systems yet exist that can detect airborne bacteria, fungi, and pollution. The new-generation aerosol mass concentration systems based on MEMS-integrated impactor systems will provide tremendous advantages, such as simultaneous and real-time detection of different types of aerosols by functionalized micro impaction surfaces. This study is the first to show a novel way of sampling based on the concept that a standard impactor will enable capturing of aerosols of different origins to provide essential information, such as mass concentration and size distribution, in real time (Algre et al. Citation2020). Therefore, this study constitutes a basis for exploring the deposition characteristics of different aerosols for real-time and highly sensitive mass concentration measurements of different species in the air. The capturing of solid aerosols on microsensors by the impaction method is strongly influenced by the bounce-effect phenomenon, which ultimately may result in inaccurate mass concentration and size measurements. Thus, the deposition characteristics of captured aerosols can lead to detrimental consequences for small-scale miniature systems. In the present study, various sizes and origins of aerosols were impacted on the smooth Si and BSi micro impaction plates with a lab-made single-stage multi-nozzle impactor to explore the deposition characteristics. The size, shape, and collection efficiency of the deposits of various sizes of aerosols differed significantly for smooth Si and BSi surfaces. Note, however, that the experiments were carried out under laboratory conditions where the ambient effects controlled, and the particle charge was neglected. Based on results obtained with the image analysis method, we did not observe any difference in the deposition characteristics of different aerosols of very similar sizes on the same impaction plate. This article suggests that the impaction characteristics of aerosols are highly subject to the bounce-effect phenomenon, which can be altered by the size of impacted particles. As a result, we distinguished the global and local collection efficiencies based on where the particles were deposited on the impaction plate. The particles in the primary impaction zone were taken into account to evaluate the local collection efficiency, which was determined as (17.9 ± 1.8)% for fluorescent particles with dae of 0.92 µm and as (<1%) for particles with dae of 2.07, 2.53, and 3.83 µm size. These micron-sized particles formed a ring shape deposit, with a nearly particle-free area under the nozzle, on a smooth Si surface. Thus, particles with a dae of 2.07, 2.53, and 3.83 µm size exhibited a significantly low local efficiency. The reduced collection efficiency in the primary impaction zone as the particle size increases is evidence of the bounce effect. This observation was also confirmed with aerosolized bacteria with sub-micron sizes of (0.66 ± 0.01) µm and (0.70 ± 0.02) µm and for the micron-sized (dae of 3.04 µm) fungus particles. Notably, based on numerous experiments, the sub-micron range can now be extended down to 0.6 µm or even below, but excluding nanoparticles (<100 µm), to obtain the same pattern characteristics. The sharp nanopillar-decorated Si surface enabled us to capture micron-sized particles under the nozzle by impaling them. Therefore, we propose to utilize nanostructured silicon MEMS mass sensors as an impaction plate to reduce the bounce effect. This creates deposits that are localized in the primary zone in the shape of a disk rather than a ring. Although porous substrates may lead to the penetration of the impinging jet, which ultimately would result in a non-ideal collection efficiency curves, more investigation on this aspect is needed. Nevertheless, the present findings show that an impactor integrated with a BSi-functionalized MEMS mass sensor is an appropriate choice for the detection of micron-sized solid particles, such as Aspergillus niger spores (dae of 3.04 µm).
Supplemental Material
Download MS Word (7.2 MB)Acknowledgments
The authors would like to thank Dr. Brice Berthelot for discussions, Mr. Anil Hami Yucel for proofreading the article and Mr. Sebastien Ritoux, Ms. Isabelle Lacaze, and Mr. Florian Huet for their technical assistance in bioaerosol generation.
Additional information
Funding
References
- Algre, E., U. Soysal, F. Marty, C. Motzkus, E. Robine, B. Berthelot, and E. Gehin. 2020. Capteur de particules fines avec microbalances en cascade. WO2020044000A1.
- Dahneke, B. 1971. The capture of aerosol particles by surfaces. J. Colloid Interface Sci. 37(2):342–53. doi:https://doi.org/10.1016/0021-9797(71)90302-X.
- Fredericks, S., and J. R. Saylor. 2018. Ring-shaped deposition patterns in small nozzle-to-plate distance impactors. Aerosol Sci. Technol. 52(1):30–7. doi:https://doi.org/10.1080/02786826.2017.1377829.
- García-Ruiz, E., F. J. Romay, J. A. García, J. F. Cambra, L. Alonso, and J. A. Legarreta. 2019. Effect of nozzle spacing in the formation of primary and secondary deposits in multi-nozzle inertial impactors part I: Experimental study. J. Aerosol Sci. 136:61–81. doi:https://doi.org/10.1016/j.jaerosci.2019.06.008.
- Ibrahim, A. H., P. F. Dunn, and R. M. Brach. 2003. Microparticle detachment from surfaces exposed to turbulent air flow: Controlled experiments and modeling. J. Aerosol Sci. 34(6):765–82. doi:https://doi.org/10.1016/S0021-8502(03)00031-4.
- May, K. R. 1945. The cascade impactor: An instrument for sampling coarse aerosols. J. Sci. Instrum. 22(10):187–95. doi:https://doi.org/10.1088/0950-7671/22/10/303.
- May, K. R. 1975. Aerosol impaction jets. J. Aerosol Sci. 6(6):403–11. doi:https://doi.org/10.1016/0021-8502(75)90056-7.
- Michaud, D., P. Picard, and M. Baril. 1999. A versatile flat-deposit impactor-type aerosol collector part 2: Calibration and quantitative study. Aerosol Sci. Technol. 31(5):338–49. doi:https://doi.org/10.1080/027868299304066.
- Nguyen, K. N., D. Abi-Saab, P. Basset, E. Richalot, M. Malak, N. Pavy, F. Flourens, F. Marty, D. Angelescu, Y. Leprince-Wang, et al. 2012. Study of black silicon obtained by cryogenic plasma etching: approach to achieve the hot spot of a thermoelectric energy harvester. Microsyst. Technol. 18(11):1807–14. doi:https://doi.org/10.1007/s00542-012-1486-0.
- Oodo, T., Y. Takashima, and M. Hanzawa. 1981. An experimental study of adhesion of particles with a round nozzle impactor. J. Chem. Eng. Jpn. 14(1):76–8. doi:https://doi.org/10.1252/jcej.14.76.
- Paw U, K. T. 1983. The rebound of particles from natural surfaces. J. Colloid Interface Sci. 93(2):442–52. doi:https://doi.org/10.1016/0021-9797(83)90428-9.
- Rocklage, J. M., V. A. Marple, and B. A. Olson. 2013. Study of secondary deposits in multiple round nozzle impactors. Aerosol Sci. Technol. 47(10):1144–51. doi:https://doi.org/10.1080/02786826.2013.823641.
- Sethi, V., and W. John. 1993. Particle impaction patterns from a circular jet. Aerosol Sci. Technol. 18(1):1–10. doi:https://doi.org/10.1080/02786829308959580.
- Soysal, U., E. Géhin, E. Algré, B. Berthelot, G. Da, and E. Robine. 2017. Aerosol mass concentration measurements: Recent advancements of real-time nano/micro systems. J. Aerosol Sci. 114:42–54. doi:https://doi.org/10.1016/j.jaerosci.2017.09.008.
- Soysal, U., F. Marty, E. Géhin, C. Motzkus, and E. Algré. 2020. Fabrication, electrical characterization and sub-ng mass resolution of sub-µm air-gap bulk mode MEMS mass sensors for the detection of airborne particles. Microelectron. Eng. 221:111190. doi:https://doi.org/10.1016/j.mee.2019.111190.
- Willeke, K., and R. E. Pavlik. 1979. Secondary effects in particle-size classification by opposing jets. J. Aerosol Sci. 10(1):1–10. doi:https://doi.org/10.1016/0021-8502(79)90130-7.
- Ziskind, G., M. Fichman, and C. Gutfinger. 1995. Resuspension of particulates from surfaces to turbulent flows—Review and analysis. J. Aerosol Sci. 26(4):613–44. doi:https://doi.org/10.1016/0021-8502(94)00139-P.