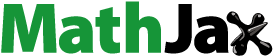
Abstract
Aerosol physicochemical mixing state, defined as the chemical, phase, and internal structure of a population of aerosols, is complex and under-characterized regarding its role in the atmosphere. The compositional and microphysical properties of the aerosol particle strongly impact its atmospheric processes, including water uptake and ice nucleation (IN) activities. However, the relationship between IN activity and particle microphysics remains underdeveloped, as the aerosol particle IN activity, composition, morphology, and size are highly intercorrelated. In this article, we demonstrate the efficacy of a microfluidic static well trap device for testing both IN activity and effloresced residual particle morphology in the same droplet. We link cationic composition with both IN activity and residual particle morphology in droplets containing sodium chloride, calcium chloride, and the biological ice nucleating particle (INP) Snomax. Finally, we show a decoupling between IN activity and residual particle morphology within the same droplet for the chemical systems studied here.
Editor:
1. Introduction
While aerosols have been studied since the nineteenth century (Hidy Citation2019), many of their impacts on human health and climate are still under-characterized on a mechanistic level, in part due to the compositional complexity of the individual particles. On short spatial and temporal scales, studying the link between chemical composition and the generation (Lindsley et al. Citation2012), transport (Ahlawat et al. Citation2022), and deposition efficiency (Legh-Land et al. Citation2021) of respiratory aerosols remains a key challenge. On larger spatial and temporal scales, systems of aerosols in the atmosphere, known as atmospheric aerosols, influence global climate and the energy balance of the earth (Szopa et al. Citation2021). Atmospheric aerosol particles are compositionally complex, and can be comprised of water, organics, soot, dust, salt, and biological components (Hinds Citation1999). For example, inorganic salts can be introduced over the ocean, where breaking waves generate sea spray aerosols (SSA) high in sodium chloride, calcium chloride, and other salts. Biological matter in aerosols such as pollen (Steiner et al. Citation2015), bacteria and fungi (Beall et al. Citation2021), and viruses (Reche et al. Citation2018) can vary seasonally and with geographic location (Burrows et al. Citation2009). Once suspended in the atmosphere, the particle composition and microphysical properties can be further altered with aging and changes of ambient conditions.
Aerosol mixing state, defined as the distribution of physical and chemical properties of particles within a suspended aerosol population, is an important predictor of atmospheric interaction parameters in aerosol particles. This broad definition of mixing state can be split into two categories: the more well studied chemical mixing state, defined as the distribution of chemical species across individual particles within an aerosol population, and the less well understood physicochemical mixing state (Riemer et al. Citation2019). Defined by Ault and Axson, physicochemical mixing state refers to the phase and internal structure of the particles in an aerosol population, providing both chemical and spatial information (Ault and Axson Citation2017). Chemical and physicochemical mixing states are impacted by the initial chemical composition of the aerosol droplet, as well as by atmospheric aging processes in which the droplet undergoes chemical reactions, phase changes, and evaporation. Both chemical and physicochemical mixing states change the optical properties of aerosol particles directly (Yao et al. Citation2022). They impact the ability of particles to act as cloud condensation nuclei (CCN), commonly referred to as CCN activity (Stevens and Dastoor Citation2019; Vu et al. Citation2019). Chemical mixing state impacts the ice nucleating (IN) activity of individual particles, where the particles which can nucleate ice within clouds are referred to as ice nucleating particles (INP). Differences in the amount and type of INP can change the overall albedo of clouds by causing portions of the cloud to freeze, leading to greater optical extinction through the cloud (Vergara-Temprado et al. Citation2018).
Partially frozen clouds, commonly referred to as mixed-phased clouds, are known to be common over the Arctic and the Southern Ocean, greatly influencing the radiative budget in these areas (Korolev et al. Citation2017). Global climate models are still not fully able to represent the contribution of mixed-phase clouds to overall climate (Mccoy et al. Citation2016). INP enable the heterogeneous freezing that can lead to mixed phase clouds, but uncertainties remain in their contribution to global climate (Bellouin et al. Citation2020) partially because different aerosol compositions create a complex set of ice nucleation pathways that are not fully characterized (Knopf, Alpert, and Wang Citation2018). Continuing efforts to establish connections between ice nucleation activity and the aerosol particles chemical and physical properties are needed, as it is important for the predictive power of global climate models (Riemer et al. Citation2019).
In the puzzle of physical and chemical properties that contribute to the physicochemical mixing state of an aerosol population, particle morphology is one important piece. Particle morphology in the atmosphere can be a spectrum of shapes and phases, with some particles being nearly spherical, and others having highly disordered fractal shapes or multiple phases. As aqueous droplets evaporate, they form dry residual particles, whose shapes and sizes may be dependent on composition (Hasenkopf et al. Citation2016), ion type (Ott and Freedman Citation2021), drying rate (Altaf and Freedman Citation2017), aging (Kaluarachchi et al. Citation2022), Peclet number (Wang et al. Citation2021), and confinement (Kohler, Pierre-Louis, and Dysthe Citation2022). Residual particles of different morphologies behave differently in the atmosphere, and the link between particle morphology and IN activity is still currently being investigated. IN activity has been shown to be a function of composition (Demott et al. Citation2003; Peterson and Tyler Citation2003; Baustian et al. Citation2012), particle size (Reicher et al. Citation2019), and surface chemistry (Zhang et al. Citation2020). However, the relationship between IN activity and particle morphology is less clear. In biomass burning aerosol, particle source can change the morphology and IN activity of the resulting aerosol (Mahrt et al. Citation2023), and atmospheric aging removes organic surface coatings on biomass burning aerosol, enhancing their IN activity (Jahl et al. Citation2021). Some studies in inorganic (Gao et al. Citation2022) and urban (Hasenkopf et al. Citation2016) aerosols have suggested IN ability is linked to particle morphology. Advanced methods are needed to aid in relating IN activity and residual particle morphology for individual particles of a given size and composition.
Single particle measurement techniques are important tools for studying physicochemical properties of particles of varying composition, shape, and size. Krieger, Marcolli, and Reid reviewed common methods of isolating single aerosol or model-aerosol particles for chemical analysis, and subsequent measurement of important aerosol properties like hygroscopicity and particle morphology (Krieger, Marcolli, and Reid Citation2012). The authors identify ways in which single particles are isolated, such as with electrodynamic balances (EDBs) and optical tweezers. Atmospheric parameters like hygroscopicity, hygroscopic growth, morphology, and phase separation can then be observed by measuring mass changes and observing phase domains with optical microscopy. For the study of INP, immersion freezing and continuous flow diffusion chambers (CFDC) have been used to explore the IN activity of multicomponent aerosol droplets from marine (Demott et al. Citation2016) and overland (Suski et al. Citation2018) environments. Immersion freezing can be combined with Raman spectroscopy for chemical analysis (Mael, Busse, and Grassian Citation2019).
Microfluidic methods have emerged comparatively recently in the field of atmospheric aerosol science, as a complementary approach to single droplet work. A review into current microfluidic methods for studying droplets, including those of atmospheric relevance, has been given by Roy, Liu, and Dutcher (Citation2021). Briefly, fluid flows can be used to determine the changing optical, thermodynamic, and rheological properties of a droplet through time, leading to information about events like freezing and phase separation. Examples of flow-through devices for ice nucleation include the MINCZ (Isenrich et al. Citation2022) and the flow-through devices used by the Murray group (Tarn et al. Citation2021) and the Dutcher group (Roy, House, et al. Citation2021). Alternatively, trapped droplets can be observed with an optical microscope under changing temperature and/or relative humidity (RH), and information about the number and type of phase transitions, as well as effloresced particle morphology, can be linked to droplet composition. Examples of static devices for ice nucleation include the WISDOM (Reicher, Segev, and Rudich Citation2018) and the static well arrays used by the Sullivan group (Brubaker et al. Citation2020) and the Dutcher group (Nandy et al. Citation2019). Microfluidic techniques can be combined with Raman spectroscopy for chemical analysis, and microfluidic devices can be temperature controlled to study low temperature phase changes and freezing (Roy et al. Citation2020; Roy, House, and Dutcher Citation2021). Because of the ability to combine temperature and RH control in a single microfluidic device, microfluidic platforms offer unique opportunities for elucidating the relationship between IN activity and residual particle morphology.
In their article, Roy et al. (Citation2021) utilized a static well trap microfluidic device to collect a freezing assay of ∼150 nanoliter-volume seawater and sea surface microlayer (SSML) samples. These same droplets were then dried, and information about the size and shape of the residual particles was obtained using optical microscopy. Information about particle composition was further explored using Raman spectroscopy, as well as heat and chemical treatments. The authors concluded that residual particle morphology and IN activity in the same aerosol particle were potentially related, with particles resulting in aggregate or amorphous appearing to have a slightly warmer IN spectrum than those with a crystalline morphology. The study was performed with seawater-based samples, with a range of ion types present.
In this article, we seek to explore this possible relationship between particle morphology and IN activity using the Roy et al. microfluidic method for coupled freezing and efflorescence experiments on the same droplets, but now utilizing a model aqueous aerosol system comprised of known amounts and ratios of inorganic salt and organic material. In Section 2, we discuss the method adapted from Roy et al. for freezing and residual particle morphology testing in a microfluidic well device and outline the compositional ratios of salts and organics used in our model system. In Section 3, we show the efficacy of our device in capturing complex biological IN behavior in Snomax bacteria and demonstrate the important role of the Ca2+ cation in both freezing behavior and residual particle morphology. In Section 4, we discuss the interaction between the Ca2+ cation and the biological particles in our model system and explain how our results suggest decoupling of IN activity and residual particle morphology in the same droplet.
2. Methods
To fabricate the microfluidic devices used in all freezing and drying experiments, a “master mold” was first created using standard photolithography techniques, as detailed in previous works (Stan et al. Citation2009; Narayanan Citation2011; Narayan et al. Citation2020; Roy et al. Citation2020). The mold is used to pattern polydimethylsiloxane (PDMS) with the device features. The PDMS device is fixed to a PDMS-coated glass slide as a backing. Details on photolithography techniques and device fabrication are provided in the online Supplemental Information. The device design is based on similar static well trap devices, such as those in Nandy et al. (Citation2019) and Roy, Liu, and Dutcher (Citation2021). The large well array design for IN studies is based on a similar design by Brubaker et al. (Citation2020) as shown in , with modifications to the channel dimensions and the number of inlet and outlet ports to make loading droplets of the desired working fluids more reliable and efficient, as shown in . As shown in , the well diameter is 450 µm; it should be noted that volume and confinement can play an important role in both freezing (Alba-Simionesco et al. Citation2006; Ginot et al. Citation2020) and efflorescence (Li et al. Citation2018). Volume analysis of droplets from the optical microscopy is described in Section 3.1. To load droplets into the microfluidic device, all channels are first flushed with filtered silicone oil (Whatman PTFE Filter, 0.02 um). Then, a syringe with a 1.2 mm needle is used to fill all channels and wells with the sample to be tested. Finally, filtered silicone oil is again injected into each channel so that the channels are cleared of excess aqueous sample while droplets of sample remain trapped in each well. The array stores 150 droplets total.
Figure 1. Schematic of the microfluidic device showing (a) the view of all wells as well as the inlet and outlet channels, and (b) detail of one well with relevant dimensions.
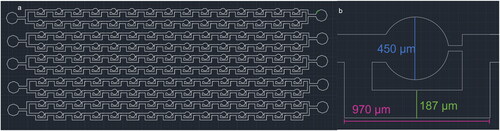
All freezing experiments take place on a temperature-controlled cold stage (LTS 420 with LNP96 cooling pump, Linkam Scientific). A mirror is placed between the cold stage and the device to reflect the image of the device to the reflected light microscope (SZX10, Olympus). Thermal grease (Ceramique 2, Arctic Silver) is used to ensure good thermal contact between the mirror and cold plate. Several drops of silicone oil are placed between the loaded device and the mirror to enhance thermal contact. Thermocouples (K Type Mini-Connector Thermocouple, HUATO Environment Monitoring System and Equipment) are slotted into each thermocouple channel, shown in , after the tips are coated in thermal grease. The device temperature, defined as the average of the four thermocouple temperatures, is calibrated using the known melting points of hydrocarbons, as detailed in the online Supplemental Information. The typical standard deviation of the temperature across the four thermocouples was approximately 1 °C. Analysis of the temperature error is provided in the online Supplemental Information. Pure water and water–salt samples, after correcting for freezing point depression, freeze below −20 °C, with an average pure water freezing temperature (T50) of −30 °C. While above the homogeneous freezing temperature due to possible environmental contaminants in the PDMS or impurities in the water (Polen et al. Citation2018), the background freezing is well below the IN spectra for the aqueous Snomax samples studied here. Finally, a custom-made acrylic box is placed over the device and cold stage, and dry nitrogen gas at a rate of about 5 SLPM is used to purge the atmosphere in the box so that condensation on the device and cold stage surfaces can be avoided during experiments. Condensation remains a challenge in the humid summer months, limiting the number of tests that can be performed in the summer.
Figure 2. (a) Simplified schematic of large well array device showing thermocouple channels (not to scale). (b) Similar design by Brubaker et al. (Citation2020) for a 720 well device (Brubaker et al. Citation2020).
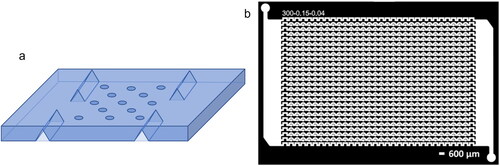
The temperature of the cold stage is dropped to −4 °C and held there for five minutes to ensure that the thermocouples have been inserted properly by checking the measured temperature. Then, the droplets are cooled at a rate of 1 °C per minute until all droplets are frozen. A video is taken of the freezing droplets with a 1200 × 1600 px monochromatic camera (acA1600-60gm, Basler) attached to the microscope. After all droplets are frozen, the device is warmed to room temperature at a rate of 10 °C/min, and all droplets melt. The thawed device is placed in a chamber at <5% RH. The relative humidity gradient in the PDMS between the droplets and the dry air causes water molecules to pervaporate from the droplets through the device into the ambient air. When all the water evaporates from the droplet, salts, bacterial matter, and organic matter are left behind and form crystal structures that differ in shape and optical properties (Nandy and Dutcher Citation2018). The residual crystal or particle left in each well is photographed with a camera (Basler) and the pictures are sorted manually to classify the particle type.
Aqueous samples were prepared using milli-Q water (Millipore). The milli-Q water was filtered with a 0.02 µm PTFE Whatman filter before mixing to minimize impurities from the background water and salt water samples. Background freezing information can be found in the SI. Snomax (Snomax International, Englewood, CO, USA) was stored in freezer at −5 °C until use to prevent aging. NaCl (Fisher Scientific) and CaCl2 (dihydrous, Millipore Sigma) were used to study salts with two different cations. shows the concentrations of salts, and Snomax in each mixture.
Table 1. Ingredients of each mixture.
The 5.25:1 NaCl:CaCl2 molar ratio was chosen because of its relevance to enrichment in SSA, where Ca2+ is seen to be enriched from seawater, as further detailed in Section 4.2. To test the effects of physiochemical degradation on Snomax bacteria, samples were heated in a water bath. Samples labeled “HT 7 min” were heated to 80 °C for 7 min. Samples labeled “HT 10 min” were heated to 80 °C for 10 min. Samples labeled “denatured” were heated to 100 °C for 20 min.
3. Results
3.1. Comparing to literature results for device validation
The first step in carrying out the large well array crystallization experiments was to verify that the device design performed comparably to other similar devices and methods for measuring the freezing behavior of warm ice nucleators like Snomax. To make this comparison, the ice nucleating active site density, or the number of ice nucleating active sites per milligram of Snomax bacteria, abbreviated as ηm, was calculated. To calculate ηm, the fraction of droplets frozen in the device, called the frozen fraction, is first calculated as a function of temperature, as shown in EquationEquation (1)(1)
(1) .
(1)
(1)
where Nf is the number of frozen droplets at a given temperature T, and N is the total number of droplets visible in the viewing window. Temperature from the four thermocouples is averaged across the device. Once frozen fraction as a function of temperature is calculated, ηm can be modeled as a time-independent function of temperature, as shown in EquationEquation (2)
(2)
(2) (Vali Citation1971).
(2)
(2)
where ηm is the cumulative ice nucleating active site density (INAS/mg), V is droplet volume, and Cm is the concentration of the ice nucleating material in the bulk solution, in this case Snomax. Since droplets in the microfluidic device are pressed into a “pancake” shape, volume is approximated as a cylinder with the semicircular border, as shown in EquationEquation (3)
(3)
(3) (Vuong and Anna Citation2012; Nandy and Dutcher Citation2018),
(3)
(3)
where h is the channel height and Ddrop is the diameter of the droplet.
The ice nucleating active site density measured in our device is plotted in , along with values from several other works focusing on Snomax IN activity. In theory, ηm should be consistent across temperature for any INP. In practice, Snomax is known to have varying ηm over a wide range of temperatures. This variation can be caused by age of the Snomax (Polen, Lawlis, and Sullivan Citation2016), storage method (Beall et al. Citation2020), and testing method (Wex et al. Citation2015).
Figure 3. Ice nucleating active site density, or ηm, for our microfluidic device compared to several sets of literature values (Wex et al. Citation2015; Polen, Lawlis, and Sullivan Citation2016; Brubaker et al. Citation2020; Roy, House, et al. Citation2021). Horizontal error bars represent uncertainty in the temperature measurement of our device.
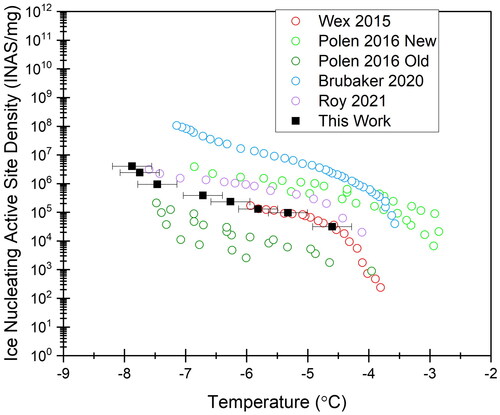
Our freezing curve and INAS density of Snomax bacteria agree with literature values. Snomax has been postulated to contain three classes of ice nucleators; class A nucleates ice above −4.5 °C, class B nucleates ice between −4.5 and −6.5 °C, and class C nucleates ice below −6.5 °C (Turner, Arellano, and Kozloff Citation1990). It has been theorized in recent work on Snomax that class A and C INP dominate, with few class B INPs (Wex et al. Citation2015; Schwidetzky et al. Citation2020). This explains the plateau region of the curves from around −4.5 to −6.5 °C, which our device captures.
3.2. Freezing point depression
The freezing point depression of the saline aqueous mixes, was calculated using Blagden’s Law for ideal dilute solutions, given by EquationEquation (4)
(4)
(4) ,
(4)
(4)
where i is the van’t Hoff factor, or the number of cations per molecule of solute, Kf is the cryoscopic constant of the solvent, and m is the molality of the solution. Using Blagden’s Law, the freezing point depression for each mixture is −3.72 °C for the NaCl containing systems, −5.58 °C for the CaCl2 containing systems, and −4.02 °C for the systems containing a 5.25:1 molar mix of NaCl to CaCl2. The freezing temperatures reported here are adjusted by these values in order to compare across samples. In tests of the saline aqueous mixtures compared to pure water, these constant offset values for freezing point depression accurately matched the freezing curves to within the error bars of our devices.
3.3. Cation dependency across treatments
Frozen fraction versus temperature and cumulative INAS density, K(T), versus temperature are shown for the monovalent NaCl and Snomax mixture, the divalent CaCl2 and Snomax mixture, and the 5.25:1 NaCl:CaCl2 mix in . These curves are corrected for freezing point depression. Heat treatments were applied because they had a predictable effect on the ice nucleating proteins in the Snomax bacteria, flattening the beta helix structures into beta sheets (Roy, House, and Dutcher Citation2021) and inactivating the bacteria’s ice nucleating capabilities. The effect of this flattening could then be compared across cation differences. In addition, we could observe the effect of gradual Snomax denaturation on final effloresced particle morphology. Even after freezing point depression correction, the mixture of 1 M divalent CaCl2 shows a significantly lower freezing activity than the monovalent mixture. The divalent curves are also more “spread” than the monovalent curves, with more warm and more cold freezers. To potentially distinguish slight differences in INAS density more clearly between the fully monovalent mixture and the monovalent–divalent mixture, the derivative form of INAS, K(T), was also calculated for these cases. The results of the derivative calculation are included in the online Supplemental Information. The role of cation enrichment on the IN activity will be discussed in more detail in Section 4.2.
Figure 4. (a) Frozen fraction versus temperature and (b) INAS versus temperature for 1 M NaCl and 0.03 mg/mL Snomax (red circles), 5.25:1 Mix and 0.03 mg/mL Snomax (purple squares), and 1 M CaCl2 and 0.03 mg/mL Snomax (blue triangles) with heat treatments applied. Crosses on plot (a) indicate the background freezing curve of pure water for these samples.
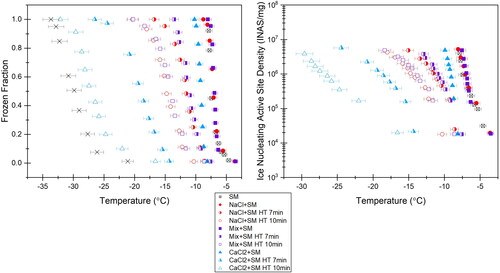
After determining our systems’ freezing behavior observed in the device, the next goal of the experiments was to explore the link, if any, between freezing point and crystal morphology. To do this, it was convenient to create five “bins” to sort different final crystal morphologies into. The categories are shown in . These categories are not discrete: rather, they represent a spectrum of disordered behavior in the final crystal morphologies observed in the wells. Similar methods of binning morphology into broad categories are used into aerosol science communities, particularly for substrate based single particle methods (Roy, House, et al. Citation2021; Kaluarachchi et al. Citation2022; Lei et al. Citation2022).
Figure 5. Example images of each particle category. The categories are as follows: Single crystals (a) with aggregate and (b) without aggregate, (c) fractal, (d) and (e) fully aggregate clusters, and (f) amorphous.
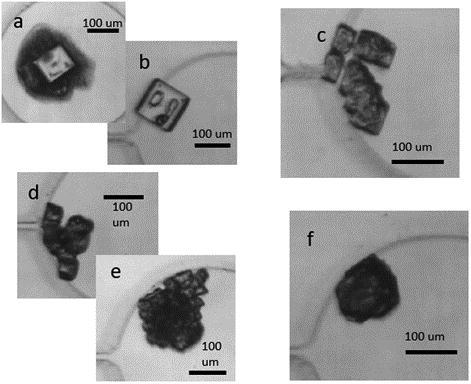
shows the percentage of each particle type for different mixtures of salt and Snomax, as well as across heat treatments. The NaCl mixtures and the divalent–monovalent salt mixtures form very different final particles, with the majority of purely monovalent salt particles being single or fractal, as expected, and no single or fractal particles present in the monovalent divalent mixture. The ‘coated’ crystal type (shown with crosshatches) also emerges with the introduction of the divalent salt, and is not observed in the purely monovalent case. Without Snomax bacteria present (“No SM”), the final residual particles for pure NaCl are more ordered than when Snomax is present. This is true even when the Snomax bacteria is inactivated. The prevalence of the coated particle type may be highest when the Snomax bacteria is partially denatured. However, taking the ± 4% error bars associated with device-to-device variability, there appears to be no correlation between the level of Snomax denaturation and the final particle morphology distribution observed.
Figure 6. Charts showing the fraction of each particle type for (a) 1 M NaCl and (b) 5.25:1 Mix. The ± 4% error bars represent the average deviation in value between two different devices containing the same mixture. Crosshatches in single category in (b) represent aggregate surrounding the single particle, which was found in all single particles in the 5.25:1 Mix.
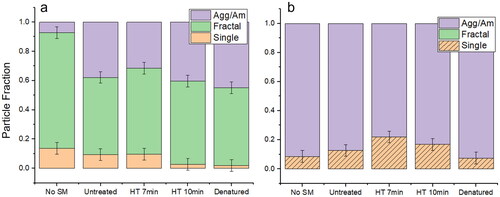
4. Discussion
4.1. Crystal type versus freezing temperature
Overall, there is no dependency observed for the monovalent/divalent mix between final particle type and freezing temperature, as shown in . In the fully monovalent mixture, there is a weak dependency on temperature, where single particle wells appear to freeze at a higher temperature on average than the other wells. However, due to low sampling numbers in the “single” category and sometimes the “aggregate/amorphous” category, these differences are not found to be statistically significant. Melting and then subsequently refreezing after efflorescence and deliquescence in the same wells was carried out for the mixture of interest, 1 M NaCl:SM. The refreezing behavior is discussed in Section 4.3 and the online Supplemental Information.
4.2. Enrichment of Ca2+ and the effects on freezing and particle morphology
Comparing our results to the results of Roy, House, and Dutcher (Citation2021) when linking freezing temperature to efflorescence particle morphology for real seawater samples, Roy et al. attributed the high level of disorder in the effloresced particles to the presence of high levels of organics, namely sialic acid. Our results show that the presence of biological compounds like Snomax bacteria, even in low concentrations, can also have an affect on final particle morphology. Roy et al. may have observed a larger fraction of the coated particle type in their studies—this could be due to the presence of other salts besides NaCl and CaCl2, such as MgCl2.
It is understood that divalent cations are enriched in SSA and SSML (Oppo et al. Citation1999; Jayarathne et al. Citation2016; Mukherjee, Reinfelder, and Gao Citation2020) and that these divalent cations lead to the enrichment of other, organic components in SSA (Schill et al. Citation2018). Ca2+ has been shown to be enriched in SSA, though the reason behind enrichment is not agreed upon. Suggested mechanisms include Ca2+ complexing to carbonate or organics (Salter et al. Citation2016), biogenic CaCO3 such as calcareous phytoplankton fragments (Sievering Citation2004; Hawkins and Russell Citation2010), and shell fragments (Keene et al. Citation2007). A recent study by de Vasquez et al. on the binding of calcium and magnesium ions shows that calcium preferentially binds as a contact ion with carboxylate (Vazquez De Vasquez et al. Citation2021). Our estimate for the enrichment of Ca2+ in our 5.25:1 molar ration Na:Ca mixture is based on a comprehensive study by Bertram et al. which gives recommendations for the mass fractions of salt used in laboratory mimics of SSA (Bertram et al. Citation2018). It is important to note that a key limitation of microfluidic methods is that the size distribution of sea spray and other aerosol is not preserved. Since the homogeneous freezing point changes with droplet volume, this could impact our observed freezing results.
1 M CaCl2 had a much lower freezing efficiency than 1 M NaCl when mixed with equal amounts of Snomax bacteria, as well as a broader spread of freezing temperatures. This trend persisted when the Snomax was heat treated. The lower freezing efficiency could be caused by the gelation of the Snomax bacteria, since Ca2+ is known to gel biological components (Silva et al. Citation2003; Richards et al. Citation2020). However, the 5.25:1 mixture showed no statistical difference in freezing behavior from the mixture containing only NaCl. This suggests that sodium chloride dominates freezing behavior, even in SSA enriched in calcium cations. It is only in the phase change of the particles with decreasing relative humidity that a difference in phase between a monovalent only mixture and monovalent-divalent mixture is observed. This is further confirmed by the results of Roy et al. (Citation2021), where bulk seawater and SSML did not show differences in freezing behavior but showed differences in final particle morphology.
4.3. Bacterial entrainment and halotolerance
It has been observed that bacteria can be entrained within fluid inclusions in salt crystals, both laboratory grown (Adamski, Roberts, and Goldstein Citation2006) and naturally occurring in a marine environment, and that the bacteria can survive and mutate to become more halotolerant (Elabed et al. Citation2019). The survivability of bacteria in high salinity conditions has become an area of great scientific interest as the saline lakes on the planet Mars are studied and modeled (Benison Citation2019; Cesur et al. Citation2022). Many of the visible imperfections in the single and fractal particles in our model mixtures could be fluid inclusions; this could be confirmed in future studies by use of Raman spectroscopy (Haixia and Guoxiang Citation2015).
The Snomax bacteria entrained in the fluid inclusions may be denatured partially or fully because of exposure to high salinity. In a study, Karimi et al. observed that P. syringae strains isolated from Snomax had no IN activity in NaCl solutions above 7% w/w NaCl (Karimi et al. Citation2020). To explore the halotolerance of Snomax bacteria, fully effloresced Snomax-NaCl particles were rehydrated in a 100% humidity environment. There was no correlation observed between the freezing order in the first test, before drying, and the freezing order in the second test, after drying-deliquescence-partial rehydration with the untreated 1 M NaCl:SM mixture. Further discussion of the details of rehydration tests is provided in the online Supplemental Information.
Finally, it is important to contextualize our results within the larger research space of freezing behavior and efflorescence of confined droplets. It is known that confinement plays a role in freezing, both in experiments and in the atmosphere. Experimentally, it has been shown that fluid–fluid and fluid–wall interactions in a confined environment have pronounced effects on freezing, and that the hydrogen bonding present in water causes water confined in pores to freeze at lower temperatures than the bulk (Alba-Simionesco et al. Citation2006). This pore freezing is theorized to occur in the atmosphere in aggregate dusts and other porous particles (Marcolli Citation2014), and is impacted by solute (Ginot et al. Citation2020). However, this behavior is often observed in pores on the nanometer scale, which are much smaller than our microfluidic wells. Confinement is also known to play a role in crystallization (Kohler, Pierre-Louis, and Dysthe Citation2022), and a wide range of microfluidic techniques have been used to study crystallization rate and final particle morphology under confinement (Meldrum and O’Shaughnessy Citation2020). While it may be impossible to rule out confinement effects in our observations in the current study, it should be noted that all final particles compared to each other were under the same degree of confinement.
5. Conclusion
Model marine-origin aerosols were studied using a 150-well microfluidic device in order to establish what link, if any, exists between composition, IN activity, and effloresced particle morphology. Droplets were loaded into the static 450 µm diameter wells, frozen, thawed, and then dried at room temperature until efflorescence of the particle occurred. Particle composition ranged from 1 M NaCl, the totally monovalent case, to 1 M CaCl2, the totally divalent case, with an intervening composition of 5.25:1 NaCl:CaCl2 to represent the most common enrichment of CaCl2 in SSA. The ice nucleating bacteria Snomax was added to represent biogenic INP found in seawater. CaCl2 decreased the IN activity of the droplets, possibly due to gelation of the Snomax bacteria forming aggregates with fewer ice nucleating active sites. The addition of Snomax bacteria affected the final particle morphology distribution of effloresced particles, and stepwise heat treatment of the Snomax, which denatured the ina-Z protein responsible for ice nucleation, did not affect the final particle morphology distribution. In addition to organic aerosol and biological INP, the method reported in this article is useful for studying the IN activity of inorganic particles such as dust, providing a platform for future studies on the effect of RH cycling on IN activity on a single droplet level.
When comparing the average freezing temperature of final particle categories for NaCl in Section 4.1, freezing temperature and final particle morphology appear to be decoupled. This suggests that final particle morphology is more likely rate dependent, volume dependent, and dependent upon the degree of confinement of the initial droplet. This theory is further confirmed by the fact that denaturing the Snomax with heat has no measurable effect on the final effloresced particle morphology distribution as a whole. This behavior warrants further study with varying drying rates, which can be completed in a relative humidity control chamber, but which is outside of the scope of this article. Snomax bacteria appeared to be denatured by the highly saline conditions undergone during the drying process. This has wider implications in the atmosphere in general, suggesting that halotolerant biogenic INP may remain more IN active through RH changes than their non-halotolerant counterparts, and that particle phase change due to RH may permanently affect IN activity by denaturing key proteins in biological INP.
Supplemental Material
Download PDF (402.6 KB)Acknowledgments
The authors thank collaborators from NSF CAICE, especially Kathryn Moore, Paul DeMott, and Thomas Hill for insight on ice nucleation experiments, Dutcher group alumnus Priyatanu Roy for manuscript feedback, and Dutcher group member Iaroslav Makhnenko for device fabrication assistance. The authors are working to make the data for this paper publicly available in the UC San Diego Library data repository system under the following reference: House, Margaret L.; Dutcher, Cari S. (2023). Data from: Microfluidic platform for coupled studies of freezing behavior and final effloresced particle morphology in Snomax® containing aqueous droplets. In Center for Aerosol Impacts on Chemistry of the Environment (CAICE). UC San Diego Library Digital Collections.
Additional information
Funding
References
- Adamski, J. C., J. A. Roberts, and R. H. Goldstein. 2006. Entrapment of bacteria in fluid inclusions in laboratory-grown halite. Astrobiology 6 (4):552–62. doi:10.1089/ast.2006.6.552.
- Ahlawat, A., S. K. Mishra, H. Herrmann, P. Rajeev, T. Gupta, V. Goel, Y. Sun, and A. Wiedensohler. 2022. Impact of chemical properties of human respiratory droplets and aerosol particles on airborne viruses’ viability and indoor transmission. Viruses 14 (7):1497. doi:10.3390/v14071497.
- Alba-Simionesco, C., B. Coasne, G. Dosseh, G. Dudziak, K. E. Gubbins, R. Radhakrishnan, and M. Sliwinska-Bartkowiak. 2006. Effects of confinement on freezing and melting. J. Phys. Condens. Matter 18 (6):R15–R68. doi:10.1088/0953-8984/18/6/R01.
- Altaf, M. B., and M. A. Freedman. 2017. Effect of drying rate on aerosol particle morphology. J. Phys. Chem. Lett. 8 (15):3613–8. doi:10.1021/acs.jpclett.7b01327.
- Ault, A. P., and J. L. Axson. 2017. Atmospheric aerosol chemistry: spectroscopic and microscopic advances. Anal. Chem. 89 (1):430–52. doi:10.1021/acs.analchem.6b04670.
- Baustian, K. J., D. J. Cziczo, M. E. Wise, K. A. Pratt, G. Kulkarni, A. G. Hallar, and M. A. Tolbert. 2012. Importance of aerosol composition, mixing state, and morphology for heterogeneous ice nucleation: A combined field and laboratory approach. J. Geophys. Res. 117 (D06):217. doi:10.1029/2011JD016784.
- Beall, C. M., D. Lucero, T. C. Hill, P. J. Demott, M. D. Stokes, and K. A. Prather. 2020. Best practices for precipitation sample storage for offline studies of ice nucleation in marine and coastal environments. Atmos. Meas. Tech. 13 (12):6473–86. doi:10.5194/amt-13-6473-2020.
- Beall, C. M., J. M. Michaud, M. A. Fish, J. Dinasquet, G. C. Cornwell, M. D. Stokes, M. D. Burkart, T. C. Hill, P. J. Demott, and K. A. Prather. 2021. Cultivable halotolerant ice-nucleating bacteria and fungi in coastal precipitation. Atmos. Chem. Phys. 21 (11):9031–45. doi:10.5194/acp-21-9031-2021.
- Bellouin, N., J. Quaas, E. Gryspeerdt, S. Kinne, P. Stier, D. Watson‐Parris, O. Boucher, K. S. Carslaw, M. Christensen, A. L. Daniau, et al. 2020. Bounding global aerosol radiative forcing of climate change. Rev. Geophys. 58 (1). doi:10.1029/2019RG000660.
- Benison, K. C. 2019. How to search for life in Martian chemical sediments and their fluid and solid inclusions using petrographic and spectroscopic methods. Front. Environ. Sci. 7:108. doi:10.3389/fenvs.2019.00108.
- Bertram, T. H., R. E. Cochran, V. H. Grassian, and E. A. Stone. 2018. Sea spray aerosol chemical composition: Elemental and molecular mimics for laboratory studies of heterogeneous and multiphase reactions. Chem. Soc. Rev. 47 (7):2374–400. doi:10.1039/c7cs00008a.
- Brubaker, T., M. Polen, P. Cheng, V. Ekambaram, J. Somers, S. L. Anna, and R. C. Sullivan. 2020. Development and characterization of a “store and create” microfluidic device to determine the heterogeneous freezing properties of ice nucleating particles. Aerosol Sci. Technol. 54 (1):79–93. doi:10.1080/02786826.2019.1679349.
- Burrows, S. M., W. Elbert, M. G. Lawrence, and U. Pöschl. 2009. Bacteria in the global atmosphere – part 1: Review and synthesis of literature data for different ecosystems. Atmos. Chem. Phys. 9 (23):9263–80. doi:10.5194/acp-9-9263-2009.
- Cesur, R. M., I. M. Ansari, F. Chen, B. C. Clark, and M. A. Schneegurt. 2022. Bacterial growth in brines formed by the deliquescence of salts relevant to cold arid worlds. Astrobiology 22 (1):104–15. doi:10.1089/ast.2020.2336.
- Demott, P. J., D. J. Cziczo, A. J. Prenni, D. M. Murphy, S. M. Kreidenweis, D. S. Thomson, R. Borys, and D. C. Rogers. 2003. Measurements of the concentration and composition of nuclei for cirrus formation. Proc. Natl. Acad. Sci. USA 100 (25):14655–60. doi:10.1073/pnas.2532677100.
- Demott, P. J., T. C. J. Hill, C. S. Mccluskey, K. A. Prather, D. B. Collins, R. C. Sullivan, M. J. Ruppel, R. H. Mason, V. E. Irish, T. Lee, et al. 2016. Sea spray aerosol as a unique source of ice nucleating particles. Proc. Natl. Acad. Sci. USA 113 (21):5797–803. doi:10.1073/pnas.1514034112.
- Elabed, H., E. González-Tortuero, C. Ibacache-Quiroga, A. Bakhrouf, P. Johnston, K. Gaddour, J. Blázquez, and A. Rodríguez-Rojas. 2019. Seawater salt-trapped Pseudomonas aeruginosa survives for years and gets primed for salinity tolerance. BMC Microbiol. 19 (1):142. doi:10.1186/s12866-019-1499-2.
- Gao, K., F. Friebel, C.-W. Zhou, and Z. A. Kanji. 2022. Enhanced soot particle ice nucleation ability induced by aggregate compaction and densification. Atmos. Chem. Phys. 22 (7):4985–5016. doi:10.5194/acp-22-4985-2022.
- Ginot, F., T. Lenavetier, D. Dedovets, and S. Deville. 2020. Solute strongly impacts freezing under confinement. Appl. Phys. Lett. 116 (25):253701. doi:10.1063/5.0008925.
- Haixia, C., and C. Guoxiang. 2015. Determining fluid compositions in the h2o-nacl-cacl2system with cryogenic raman spectroscopy: Application to natural fluid inclusions. Acta Geol. Sin. Engl. Ed. 89 (3):894–901. doi:10.1111/1755-6724.12487.
- Hasenkopf, C. A., D. P. Veghte, G. P. Schill, S. Lodoysamba, M. A. Freedman, and M. A. Tolbert. 2016. Ice nucleation, shape, and composition of aerosol particles in one of the most polluted cities in the world: Ulaanbaatar, mongolia. Atmos. Environ. 139:222–9. doi:10.1016/j.atmosenv.2016.05.037.
- Hawkins, L. N., and L. M. Russell. 2010. Polysaccharides, proteins, and phytoplankton fragments: Four chemically distinct types of marine primary organic aerosol classified by single particle spectromicroscopy. Adv. Meteorol. 2010:1–14. doi:10.1155/2010/612132.
- Hidy, G. M. 2019. Atmospheric aerosols: Some highlights and highlighters, 1950 to 2018. Aerosol. Sci. Eng. 3 (1):1–20. doi:10.1007/s41810-019-00039-0.
- Hinds, W. C. 1999. Aerosol technology: Properties, behavior, and measurement of airborne particles. New York: Wiley.
- Isenrich, F. N., N. Shardt, M. Rösch, J. Nette, S. Stavrakis, C. Marcolli, Z. A. Kanji, A. J. Demello, and U. Lohmann. 2022. The microfluidic ice nuclei counter Zürich (Mincz): a platform for homogeneous and heterogeneous ice nucleation. Atmos. Meas. Tech. 15 (18):5367–81. doi:10.5194/amt-15-5367-2022.
- Jahl, L. G., T. A. Brubaker, M. J. Polen, L. G. Jahn, K. P. Cain, B. B. Bowers, W. D. Fahy, S. Graves, and R. C. Sullivan. 2021. Atmospheric aging enhances the ice nucleation ability of biomass-burning aerosol. Sci. Adv. 7 (9):eabd3440. doi:10.1126/sciadv.abd3440.
- Jayarathne, T., C. M. Sultana, C. Lee, F. Malfatti, J. L. Cox, M. A. Pendergraft, K. A. Moore, F. Azam, A. V. Tivanski, C. D. Cappa, et al. 2016. Enrichment of saccharides and divalent cations in sea spray aerosol during two phytoplankton blooms. Environ. Sci. Technol. 50 (21):11511–20. doi:10.1021/acs.est.6b02988.
- Kaluarachchi, C. P., V. W. Or, Y. Lan, E. S. Hasenecz, D. Kim, C. K. Madawala, G. P. Dorcé, K. J. Mayer, J. S. Sauer, C. Lee, et al. 2022. Effects of atmospheric aging processes on nascent sea spray aerosol physicochemical properties. ACS Earth Space Chem. 6 (11):2732–44. doi:10.1021/acsearthspacechem.2c00258.
- Karimi, B., R. Nosrati, B. S. Fazly Bazzaz, M. Mirpour, M. Malboobi, and P. Owlia. 2020. A comparative evaluation of freezing criteria and molecular characterization of epiphytic ice-nucleating (ice+) and non-ice-nucleating (ice−) Pseudomonas syringae and pseudomonas fluorescens. J. Plant Pathol. 102:169–78. doi:10.1007/s42161-019-00402-7.
- Keene, W. C., H. Maring, J. R. Maben, D. J. Kieber, A. A. P. Pszenny, E. E. Dahl, M. A. Izaguirre, A. J. Davis, M. S. Long, X. Zhou, et al. 2007. Chemical and physical characteristics of nascent aerosols produced by bursting bubbles at a model air-sea interface. J. Geophys. Res. 112 (D21):202. doi:10.1029/2007JD008464.
- Knopf, D. A., P. A. Alpert, and B. Wang. 2018. The role of organic aerosol in atmospheric ice nucleation: a review. ACS Earth Space Chem. 2 (3):168–202. doi:10.1021/acsearthspacechem.7b00120.
- Kohler, F., O. Pierre-Louis, and D. K. Dysthe. 2022. Crystal growth in confinement. Nat. Commun. 13 (1):6990. doi:10.1038/s41467-022-34330-5.
- Korolev, A., G. Mcfarquhar, P. R. Field, C. Franklin, P. Lawson, Z. Wang, E. Williams, S. J. Abel, D. Axisa, S. Borrmann, et al. 2017. Mixed-phase clouds: progress and challenges. Meteorol. Monogr. 58 (1):5.1–.50. doi:10.1175/AMSMONOGRAPHS-D-17-0001.1.
- Krieger, U. K., C. Marcolli, and J. P. Reid. 2012. Exploring the complexity of aerosol particle properties and processes using single particle techniques. Chem. Soc. Rev. 41 (19):6631–62. doi:10.1039/c2cs35082c.
- Legh-Land, V., A. E. Haddrell, D. Lewis, D. Murnane, and J. P. Reid. 2021. Water uptake by evaporating PMDI aerosol prior to inhalation affects both regional and total deposition in the respiratory system. Pharmaceutics 13 (7):941. doi:10.3390/pharmaceutics13070941.
- Lei, Z., Y. Chen, Y. Zhang, M. E. Cooke, I. R. Ledsky, N. C. Armstrong, N. E. Olson, Z. Zhang, A. Gold, J. D. Surratt, et al. 2022. Initial ph governs secondary organic aerosol phase state and morphology after uptake of isoprene epoxydiols (iepox). Environ. Sci. Technol. 56 (15):10596–607. doi:10.1021/acs.est.2c01579.
- Li, L., J. R. Sanchez, F. Kohler, A. Røyne, and D. K. Dysthe. 2018. Microfluidic control of nucleation and growth of caco3. Crystal Growth Design 18 (8):4528–35. doi:10.1021/acs.cgd.8b00508.
- Lindsley, W. G., T. A. Pearce, J. B. Hudnall, K. A. Davis, S. M. Davis, M. A. Fisher, R. Khakoo, J. E. Palmer, K. E. Clark, I. Celik, et al. 2012. Quantity and size distribution of cough-generated aerosol particles produced by influenza patients during and after illness. J. Occup. Environ. Hyg. 9 (7):443–9. doi:10.1080/15459624.2012.684582.
- Mael, L. E., H. Busse, and V. H. Grassian. 2019. Measurements of immersion freezing and heterogeneous chemistry of atmospherically relevant single particles with micro-Raman spectroscopy. Anal. Chem. 91 (17):11138–45. doi:10.1021/acs.analchem.9b01819.
- Mahrt, F., C. Rösch, K. Gao, C. H. Dreimol, M. A. Zawadowicz, and Z. A. Kanji. 2023. Physicochemical properties of charcoal aerosols derived from biomass pyrolysis affect their ice-nucleating abilities at cirrus and mixed-phase cloud conditions. Atmos. Chem. Phys. 23 (2):1285–308. doi:10.5194/acp-23-1285-2023.
- Marcolli, C. 2014. Deposition nucleation viewed as homogeneous or immersion freezing in pores and cavities. Atmos. Chem. Phys. 14 (4):2071–104. doi:10.5194/acp-14-2071-2014.
- Mccoy, D. T., I. Tan, D. L. Hartmann, M. D. Zelinka, and T. Storelvmo. 2016. On the relationships among cloud cover, mixed‐phase partitioning, and planetary albedo in GCMS. J. Adv. Model. Earth Syst. 8 (2):650–68. doi:10.1002/2015MS000589.
- Meldrum, F. C., and C. O’Shaughnessy. 2020. Crystallization in confinement. Adv. Mater. 32 (31):2001068. doi:10.1002/adma.202001068.
- Mukherjee, P., J. R. Reinfelder, and Y. Gao. 2020. Enrichment of calcium in sea spray aerosol in the arctic summer atmosphere. Mar. Chem. 227:103898. doi:10.1016/j.marchem.2020.103898.
- Nandy, L., and C. S. Dutcher. 2018. Phase behavior of ammonium sulfate with organic acid solutions in aqueous aerosol mimics using microfluidic traps. J. Phys. Chem. B 122 (13):3480–90. doi:10.1021/acs.jpcb.7b10655.
- Nandy, L., S. Liu, C. Gunsbury, X. Wang, M. A. Pendergraft, K. A. Prather, and C. S. Dutcher. 2019. Multistep phase transitions in sea surface microlayer droplets and aerosol mimics using microfluidic wells. ACS Earth Space Chem. 3 (7):1260–7. doi:10.1021/acsearthspacechem.9b00121.
- Narayan, S., I. Makhnenko, D. B. Moravec, B. G. Hauser, A. J. Dallas, and C. S. Dutcher. 2020. Insights into the microscale coalescence behavior of surfactant-stabilized droplets using a microfluidic hydrodynamic trap. Langmuir 36 (33):9827–42. doi:10.1021/acs.langmuir.0c01414.
- Narayanan, S. 2011. Gas assisted thin-film evaporation from confined spaces. ProQuest Dissertations and Theses 213.
- Oppo, C., S. Bellandi, N. Degli Innocenti, A. M. Stortini, G. Loglio, E. Schiavuta, and R. Cini. 1999. Surfactant components of marine organic matter as agents for biogeochemical fractionation and pollutant transport via marine aerosols. Mar. Chem. 63 (3–4):235–53. doi:10.1016/s0304-4203(98)00065-6.
- Ott, E.-J E., and M. A. Freedman. 2021. Influence of ions on the size dependent morphology of aerosol particles. ACS Earth Space Chem. 5 (9):2320–8. doi:10.1021/acsearthspacechem.1c00210.
- Peterson, R. E., and B. J. Tyler. 2003. Surface composition of atmospheric aerosol: Individual particle characterization by TOF-SIMS. Appl. Surf. Sci. 203–204:751–6. doi:10.1016/S0169-4332(02)00812-7.
- Polen, M., T. Brubaker, J. Somers, and R. C. Sullivan. 2018. Cleaning up our water: Reducing interferences from nonhomogeneous freezing of “pure” water in droplet freezing assays of ice-nucleating particles. Atmos. Meas. Tech. 11 (9):5315–34. doi:10.5194/amt-11-5315-2018.
- Polen, M., E. Lawlis, and R. C. Sullivan. 2016. The unstable ice nucleation properties of snomax® bacterial particles. JGR. Atmos. 121 (19):11,666–11,678. doi:10.1002/2016JD025251.
- Reche, I., G. D’Orta, N. Mladenov, D. M. Winget, and C. A. Suttle. 2018. Deposition rates of viruses and bacteria above the atmospheric boundary layer. ISME J. 12:1154–62. doi:10.1038/s41396-017-0042-4.
- Reicher, N., C. Budke, L. Eickhoff, S. Raveh-Rubin, I. Kaplan-Ashiri, T. Koop, and Y. Rudich. 2019. Size-dependent ice nucleation by airborne particles during dust events in the eastern Mediterranean. Atmos. Chem. Phys. 19 (17):11143–58. doi:10.5194/acp-19-11143-2019.
- Reicher, N., L. Segev, and Y. Rudich. 2018. The weizmann supercooled droplets observation on a microarray (wisdom) and application for ambient dust. Atmos. Meas. Tech. 11 (1):233–48. doi:10.5194/amt-11-233-2018.
- Richards, D. A.-O., K. A.-O. Trobaugh, J. A.-O. Hajek-Herrera, C. L. Price, C. S. Sheldon, J. F. Davies, and R. A.-O. Davis. 2020. Ion-molecule interactions enable unexpected phase transitions in organic-inorganic aerosol. Sci. Adv. 6 (47):eabb5643.
- Riemer, N., A. P. Ault, M. West, R. L. Craig, and J. H. Curtis. 2019. Aerosol mixing state: Measurements, modeling, and impacts. Rev. Geophys. 57 (2):187–249. doi:10.1029/2018RG000615.
- Roy, P., M. L. House, and C. S. Dutcher. 2021. A microfluidic device for automated high throughput detection of ice nucleation of snomax®. Micromachines 12 (3):296. doi:10.3390/mi12030296.
- Roy, P., S. Liu, and C. S. Dutcher. 2021. Droplet interfacial tensions and phase transitions measured in microfluidic channels. Annu. Rev. Phys. Chem. 72:73–97. doi:10.1146/annurev-physchem-090419-105522.
- Roy, P., L. E. Mael, T. C. J. Hill, L. Mehndiratta, G. Peiker, M. L. House, P. J. Demott, V. H. Grassian, and C. S. Dutcher. 2021. Ice nucleating activity and residual particle morphology of bulk seawater and sea surface microlayer. ACS Earth Space Chem. 5 (8):1916–28. doi:10.1021/acsearthspacechem.1c00175.
- Roy, P., L. E. Mael, I. Makhnenko, R. Martz, V. H. Grassian, and C. S. Dutcher. 2020. Temperature-dependent phase transitions of aqueous aerosol droplet systems in microfluidic traps. ACS Earth Space Chem. 4 (9):1527–39. doi:10.1021/acsearthspacechem.0c00114.
- Salter, M. E., E. Hamacher-Barth, C. Leck, J. Werner, C. M. Johnson, I. Riipinen, E. D. Nilsson, and P. Zieger. 2016. Calcium enrichment in sea spray aerosol particles. Geophys. Res. Lett. 43 (15):8277–85. doi:10.1002/2016GL070275.
- Schill, S., S. Burrows, E. Hasenecz, E. Stone, and T. Bertram. 2018. The impact of divalent cations on the enrichment of soluble saccharides in primary sea spray aerosol. Atmosphere 9 (12):476. doi:10.3390/atmos9120476.
- Schwidetzky, R., A. T. Kunert, M. Bonn, U. Pöschl, H. Ramløv, A. L. Devries, J. Fröhlich-Nowoisky, and K. Meister. 2020. Inhibition of bacterial ice nucleators is not an intrinsic property of antifreeze proteins. J. Phys. Chem. B 124 (24):4889–95. doi:10.1021/acs.jpcb.0c03001.
- Sievering, H. 2004. Aerosol non-sea-salt sulfate in the remote marine boundary layer under clear-sky and normal cloudiness conditions: Ocean-derived biogenic alkalinity enhances sea-salt sulfate production by ozone oxidation. J. Geophys. Res. 109 (D19):317. doi:10.1029/2003JD004315.
- Silva, D. A., A. C. F. Brito, R. C. M. De Paula, J. P. A. Feitosa, and H. C. B. Paula. 2003. Effect of mono and divalent salts on gelation of native, na and deacetylated sterculia striata and sterculia urens polysaccharide gels. Carbohydr. Polym. 54 (2):229–36. doi:10.1016/s0144-8617(03)00163-2.
- Stan, C. A., G. F. Schneider, S. S. Shevkoplyas, M. Hashimoto, M. Ibanescu, B. J. Wiley, and G. M. Whitesides. 2009. A microfluidic apparatus for the study of ice nucleation in supercooled water drops. Lab Chip. 9 (16):2293–305. doi:10.1039/b906198c.
- Steiner, A. L., S. D. Brooks, C. Deng, D. C. O. Thornton, M. W. Pendleton, and V. Bryant. 2015. Pollen as atmospheric cloud condensation nuclei. Geophys. Res. Lett. 42 (9):3596–602. doi:10.1002/2015GL064060.
- Stevens, R., and A. Dastoor. 2019. A review of the representation of aerosol mixing state in atmospheric models. Atmosphere 10 (4):168. doi:10.3390/atmos10040168.
- Suski, K. J., T. C. J. Hill, E. J. T. Levin, A. Miller, P. J. Demott, and S. M. Kreidenweis. 2018. Agricultural harvesting emissions of ice-nucleating particles. Atmos. Chem. Phys. 18 (18):13755–71. doi:10.5194/acp-18-13755-2018.
- Szopa, S., V. Naik, B. Adhikary, P. Artaxo, T. Berntsen, W. D. Collins, S. Fuzzi, L. Gallardo, A. Kiendler-Scharr, Z. Klimont, et al. 2021. Short-lived climate forcers. In Climate Change 2021: The Physical Science Basis. Contribution of Working Group I to the Sixth Assessment Report of the Intergovernmental Panel on Climate Change, 817–922. Cambridge, United Kingdom: Cambridge University Press.
- Tarn, M. D., S. N. F. Sikora, G. C. E. Porter, J.-U. Shim, and B. J. Murray. 2021. Homogeneous freezing of water using microfluidics. Micromachines 12 (2):223. doi:10.3390/mi12020223.
- Turner, M. A., F. Arellano, and L. M. Kozloff. 1990. Three separate classes of bacterial ice nucleation structures. J. Bacteriol. 172 (5):2521–6. doi:10.1128/jb.172.5.2521-2526.1990.
- Vali, G. 1971. Quantitative evaluation of experimental results on the heterogeneous freezing nucleation of supercooled liquids. J. Atmos. Sci. 28 (3):402–9. doi:10.1175/1520-0469(1971)028<0402:qeoera>2.0.co;2.
- Vazquez De Vasquez, M. G., B. A. Wellen Rudd, M. D. Baer, E. E. Beasley, and H. C. Allen. 2021. Role of hydration in magnesium versus calcium ion pairing with carboxylate: solution and the aqueous interface. J. Phys. Chem. B 125 (40):11308–19. doi:10.1021/acs.jpcb.1c06108.
- Vergara-Temprado, J., A. K. Miltenberger, K. Furtado, D. P. Grosvenor, B. J. Shipway, A. A. Hill, J. M. Wilkinson, P. R. Field, B. J. Murray, and K. S. Carslaw. 2018. Strong control of southern ocean cloud reflectivity by ice-nucleating particles. Proc. Natl. Acad. Sci. USA 115 (11):2687–92. doi:10.1073/pnas.1721627115.
- Vu, D., S. Gao, T. Berte, M. Kacarab, Q. Yao, K. Vafai, and A. Asa-Awuku. 2019. External and internal cloud condensation nuclei (ccn) mixtures: controlled laboratory studies of varying mixing states. Atmos. Meas. Tech. 12 (8):4277–89. doi:10.5194/amt-12-4277-2019.
- Vuong, S. M., and S. L. Anna. 2012. Tuning bubbly structures in microchannels. Biomicrofluidics 6 (2):22004–2200418. doi:10.1063/1.3693605.
- Wang, Z., M. Ordoubadi, H. Wang, and R. Vehring. 2021. Morphology and formation of crystalline leucine microparticles from a co-solvent system using multi-orifice monodisperse spray drying. Aerosol Sci. Technol. 55 (8):901–19. doi:10.1080/02786826.2021.1904129.
- Wex, H., S. Augustin-Bauditz, Y. Boose, C. Budke, J. Curtius, K. Diehl, A. Dreyer, F. Frank, S. Hartmann, N. Hiranuma, et al. 2015. Intercomparing different devices for the investigation of ice nucleating particles using snomax® as test substance. Atmos. Chem. Phys. 15 (3):1463–85. doi:10.5194/acp-15-1463-2015.
- Yao, Y., J. H. Curtis, J. Ching, Z. Zheng, and N. Riemer. 2022. Quantifying the effects of mixing state on aerosol optical properties. Atmos. Chem. Phys. 22 (14):9265–82. doi:10.5194/acp-22-9265-2022.
- Zhang, C., Y. Zhang, M. J. Wolf, L. Nichman, C. Shen, T. B. Onasch, L. Chen, and D. J. Cziczo. 2020. The effects of morphology, mobility size, and secondary organic aerosol (soa) material coating on the ice nucleation activity of black carbon in the cirrus regime. Atmos. Chem. Phys. 20 (22):13957–84. doi:10.5194/acp-20-13957-2020.