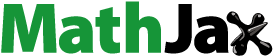
Abstract
Nano-plastics have been found in snow and evidence shows that they can act as nuclei. The aerosol water-uptake ability is an important property that will determine wet deposition, a potential main route for nano-plastics to fall to the ground. In this work, we measure the hygroscopicity of three nano-plastics: low-density polyethylene (LDPE), polyethylene terephthalate (PET) and polyvinyl chloride (PVC), and a fourth compound, cellulose, with in-situ aerosol techniques via a cloud condensation nuclei counter. Cellulose is a high-molecular weight compound and a main component of commonly used paper products. Ultrafine nano-plastic particles in the range of 20 to 200 nm are explored. Nano-plastic materials LDPE, PET, and PVC form droplets more readily than cellulose nanoparticles. The high hygroscopicity of nano-plastics indicates that the nano-plastics are either oligomers or a polymer with properties for water adsorption. Furthermore, a single parameter hygroscopicity, κ, derived from different droplet activation models (Flory Huggins Köhler and Frenkel-Halsey-Hill adsorption theory) are reported. Moreover, the molecular weights of the leached oligomers are predicted by Flory Huggins Köhler theory assuming ideal polymer mixing. The observed intrinsic hygroscopicity is size-dependent, hence the adsorption-based hygroscopicity model could be applied. Nano-plastics can be incorporated into clouds, transported globally, and may also be removed from the atmosphere via wet deposition processes. The analysis of hygroscopicity help estimating the lifetime and transport of nano-plastic particles in the atmosphere.
EDITOR:
1. Introduction
Micro plastic particles identified in aquatic (Andrady Citation2011) and atmospheric systems (Prata Citation2018) have raised concerns. Recent work has explored the biological impacts of these anthropogenic particles on living things. Specifically, Haegerbaeumer et al. reviewed the effects of plastic particles on small aquatic organisms (Haegerbaeumer et al. Citation2019). Micro (and potentially smaller) plastics were found in benthic fauna digestive systems. Furthermore nano and larger plastic debris can affect the food chain, impact creatures health via bioaccumulation (Ma et al. Citation2016; Sökmen et al. Citation2020). In 2018, Prata raised concerns that small hydrophobic airborne nano- particles could cross the blood brain barrier (Prata Citation2018). In the same study, micro-plastics particles were found in the respiratory system of humans resulting in inflammation and cytotoxicity.
In recent studies, micro and nano-plastic particles have been found in remote areas, such as the Antarctic (Munari et al. Citation2017), the Arctic (Cózar et al. Citation2017), New Zealand (Fan et al. Citation2022) and Pyrenees Mountains (Allen et al. Citation2019), suggesting that they have a long and mobile lifetime in the atmosphere. Allen et al. sampled air masses and found that micro plastic particles could travel as far as 95 km (Allen et al. Citation2019). It has been hypothesized that plastic particles entered the atmosphere from aerosolized sea spray (Allen et al. Citation2020; Lehmann et al. Citation2021; Trainic et al. Citation2020), were subsequently transported to cold areas, and then precipitated as either droplets or snow to the earth’s surface. Micro and nano plastics have been found in freshly fallen snow (Bergmann et al. Citation2019; Materić et al. Citation2020; Z. Wang, Saadé, and Ariya Citation2021), thus providing evidence to the theory of long range atmospheric transport. Plastic particles may act as both cloud and ice condensation nuclei (Aeschlimann et al. Citation2022). Wet deposition is a possible route for plastic removal in the atmosphere. However, the mechanisms by which plastics act as warm and cold cloud seeds are not well understood. Furthermore, the intrinsic ability to uptake water (hygroscopicity) of airborne plastic particles will help determine a particle’s fate and transport in atmospheric and aquatic environments.
Micro and nano-plastics can be difficult to characterize, especially when the sizes of plastics are in nanometer size ranges (Ivleva Citation2021). Techniques that have studied nano-plastic particles include Optical Traps (Gillibert et al. Citation2019), Nanoparticle tracking analysis (NTA)(Filipe, Hawe, and Jiskoot Citation2010), and Dynamic Light Scattering (DLS). The aforementioned particle methods can be combined with additional instrumentation to identify the chemical composition, such as ICP-MS (Lai et al. Citation2021), FTIR (Zangmeister et al. Citation2022) or gas chromatography − mass spectrometry (GC-MS). Gillibert et al. used Ramen tweezers to analyze the size and the morphology of nylon and polypropylene small plastics particles in sea water (Gillibert et al. Citation2019). Ter Halle et al. found plastic debris smaller than 1000 nm in North Atlantic subtropical gyre using DLS, but an accurate size distribution was hard to obtain (Ter Halle et al. Citation2017). Notably, these techniques identified plastic particles in aqueous solutions. Additionally, plastic particles can also be aerosolized and counted in air (Zangmeister et al. Citation2022). In 2022, Zangmeister et al. used a differential mobility analyzer (DMA) with a condensation particle counter (CPC) in series and showed a full size distribution of nano-particles leaching from consumer products. Plastic consumer products including the LDPE and nylon were placed in a hot water bath. The nano-plastics in the water were then atomized and a log-normal distributed nanoparticles were classified by their electromobility. They then showed that the nano-plastics can be aerosolized, and that the combination of a DMA with a CPC can be used to effectively detect plastic nano particles and measure the sizes of nano plastics (Hiranuma et al. Citation2011; Mao, Gohil, and Asa-Awuku Citation2022).
In the atmosphere, such nano-plastics may provide a surface for water vapor to condense. Large (∼micron) particles can provide sufficient surface area but are prone to gravitational settling (Williams et al. Citation2002). Whereas nano-scale particles have longer atmospheric lifetimes and their ability to form droplets, act as cloud condensation or ice nuclei is determined by both particle size and chemistry. Recently, Yang et al. found that the submicrometer particles leached from textiles could potentially be oligomers (Yang et al. Citation2024). They showed that the leached particles from textiles dissolved in alcohol, but the polyethylene terephthalate (PET) particles did not. We know little about the fate of these oligomers leaching from the plastics as well (Shi et al. Citation2023). The molecular weight of oligomers are smaller than polymers, and hence oligomers can serve as the cloud condensation nuclei more easily. Moreover, the oligomer in the atmosphere has the potential to further polymerize and become nano-plastics (Kalberer et al. Citation2004).
Recently, Wlasits et al. has shown the cloud condensation probability of PET particles using a nano-DMA (Wlasits, Konrat, and Winkler Citation2023). In this work, we employ widely used aerosol measurement techniques to measure the size distribution and droplet forming ability of nano-plastics. Specifically, a DMA and a CPC is simultaneously connected to a cloud condensation nuclei counter (CCNC) and measures CCN activity of the nano-plastics exposed to constant relative humidity. The hygroscopicity of three plastics: low density polyethylene (LDPE), polyvinyl chloride (PVC), and PET, are measured and estimated. LDPE, PVC and PET contain polymeric carbon chains and all three are considered hydrophobic compounds (Karim et al. Citation2022; Maláč, Altmann, and Zelinger Citation1970; McCall et al. Citation1984). The hygroscopicity of cellulose, a main component of paper products is also measured and reported for comparison. The hygroscopic properties of aerosols determine whether particles have the propensity to precipitate to the surface. Specifically, we apply the Frenkel-Halsey-Hill adsorption theory single parametrization hygroscopicity model (FHH-AT) (Mao, Gohil, and Asa-Awuku Citation2022) and the Flory-Huggins Köhler (FHK) (Mao, Malek, and Asa-Awuku Citation2021) to describe the water-uptake ability of the nano-plastics and cellulose. A single hygroscopicity value is reported for all compounds and the implications of these measurements are then discussed.
2. Materials and methods
2.1. Aerosol measurement
Dry nanoparticles were generated from atomized solutions. 0.3 grams of low density polyethylene (LDPE, Alfa Aesar), polyvinyl chloride (PVC, Sigma Aldrich, 99%+), and cellulose (Sigma Aldrich, 99%+) or 3 g of granular polyethylene terephthalate (PET, Sigma Aldrich, 99%+) were placed into 200 ml of ultra-purified water (UPW) (Millipore® 18MΩ) in separate Pyrex® glass bottles. The bottles were stored under room temperature and placed overnight for the water uptake measurement. The size distribution of nano-plastics leached from the bulk material can be measured by the aerosol techniques, especially a scanning mobility particle sizer (SMPS) (Zangmeister et al. Citation2022). To further identify the existence of the nano-plastics (LDPE, PVC and PET), a transmission electron microscope (TEM) was used. Chlorine in PVC particles were also detected by the Energy-dispersive X-ray spectroscopy (EDXS). The TEM and EDXS results of nano-plastics were in supplementary documents, showing that the leaching of nano-plastics occurs.
A Collison type atomizer then generated wet droplets and the wet particles were dried via a silica dryer. The dry polydisperse ultrafine aerosols are then sampled at 0.8 liter per minute with SMPS (S. C. Wang and Flagan Citation1990). The sheath to aerosol flow rate is then set at a ratio of 10:1. The SMPS consists of an Electrostatic Classifier, Differential Mobility Analyzer (TSI DMA 3080) and Condensation Particle Counter (TSI CPC 3776). The DMA size selects the polydisperse dry aerosols by electrical mobility and produces monodisperse aerosol. The monodisperse aerosol were then split into the CPC and Cloud Condensation Nuclei Counter (TSI CCNC-100) (Roberts and Nenes Citation2005). The CPC samples aerosol at 0.3 liters per minute and the CCNC samples aerosol at 0.5 liters per minute. The total particle concentration (CN) is counted by the CPC and the concentration of particles that form droplets at a selected size and constant supersaturation (CCN) is counted by the CCNC. The ratio of the CCN to CN is computed with Scanning Mobility CCN Analysis Method (SMCA) (Moore, Nenes, and Medina Citation2010) and a critical diameter (Dc) is computed for a constant supersaturation. For a given constant supersaturation, s, and compound, the experiment was repeated four times. By measuring the CCN/CN ratio at several different supersaturations, supersaturation and activation dry diameter (s-Dc) data was obtained. The supersaturation was then varied from 0.3% to 1.5%. (s-Dc) measurements are then required to calculate nanoparticle hygroscopicity.
2.2. The hygroscopicity theory
Aerosol hygroscopicity can be measured and reported via the representation of a single hygroscopicity parameter, κ. Briefly described here, the κ of aerosols found in the atmosphere can vary from 0 to 1.4 (Petters and Kreidenweis Citation2007). Inorganic salts, like ammonium sulfate, are known to be hygroscopic with measured and predicted κ = 0.604 (Kreidenweis and Asa-Awuku Citation2013; Petters and Kreidenweis Citation2007; Tang et al. Citation2019). Wettable but hydrophobic species, such as graphitic soot and high molecular weight hydrophobic polymers, are assumed to have κ = 0. Simple and ideal assumptions are known to reproduce the droplet growth hygroscopicity of water-soluble and inorganic compounds.
Traditional hygroscopicity depends on the molecular weight of the solute:
(1)
(1)
Where is the van’t Hoff coefficient,
is the molecular weight of the solute,
is the molecular weight of the solute,
is the density of the solute, and
is the density of water.
Recently, two models have been developed to calculate the hygroscopicity of polymeric and water-insoluble particles. The FHK-hygroscopicity is aptly applied to water-soluble oligomer or polymer and FHH-AT-hygroscopicity has been applied to water-insoluble solid. FHK uses one fitting parameter and was incorporated into a single-parameter hygroscopicity term that describes the water-uptake of water-soluble aerosol. FHK has been shown to aptly reproduce the hygroscopicity of biodegradable hydrophilic polymeric aerosol, like PEG, and gelatin (Mao, Malek, and Asa-Awuku Citation2021). The FHK-hygroscopicity () was developed by Mao, Malek, and Asa-Awuku (Citation2021) and a simplified equation is described as follow:
(2)
(2)
is the volume fraction of the polymer.
is the reciprocal of the chain segments of the polymer equal to the ratio of the molecular volume of water and the solute.
approaches to zero when for polymer when the molecular weight can be assumed infinite large.
is the Flory-Huggins interaction parameter.
describes the water affinity of the polymer.
if the droplet is an ideal solution when activated. When
is equal to the traditional hygroscopicity, which has the same value of
Additional details of the
can be found in Mao, Malek, and Asa-Awuku (Citation2021).
FHH-AT-hygroscopicity has been applied to water-insoluble polymers of known nano-sizes (Mao, Gohil, and Asa-Awuku Citation2022; Mao, Malek, and Asa-Awuku Citation2021) and black carbonaceous aerosol (Gohil et al. Citation2023). FHH-AT assumes the solute is water-insoluble and that water may adsorb on the particle surface. FHH-AT determines droplet growth using two empirical parameters, AFHH and BFHH (Sorjamaa and Laaksonen Citation2007). The parameter AFHH is related to the interaction of the first layer of water and the particle surface. The BFHH represents the interaction between other layers of water molecules and the particles. Furthermore, it is postulated that AFHH should range from 0.1 to 3.0, and BFHH should be within range of 0.5 to 3.0 for mineral dust aerosols (Kumar et al. Citation2009). The FHH-AT-hygroscopicity () was developed by Mao, Gohil, and Asa-Awuku (Citation2022) and is derived from experimental (
) and theoretical (
) inputs as follows:
(3)
(3)
(4)
(4)
are the dry and wet diameters respectively.
is the surface coverage and describes the layers of water molecules adsorbed on to the dry particle surface.
is the diameter of a single water molecule and equals to 0.275 nm.
and
are compound specific empirical parameters. The two empirical parameters are fitted from the s-Dc data. Furthermore,
is size dependent and decreases with the increasing particle sizes.
It should be noted that numerous CCN studies employ traditional Köhler theory derived hygroscopicity for analysis and prediction (Mao, Gohil, and Asa-Awuku Citation2022; Mao, Malek, and Asa-Awuku Citation2021; Sullivan et al. Citation2009). Köhler theory assumes complete dissolution for non-polymeric compounds. Here, we introduce two more hygroscopicity models: water-uptake due to adsorption and no dissolution with the mechanism, and water-uptake affect by the molecular weight with the
mechanism. Furthermore, the two mechanisms are used to compare theoretical and values derived from observation; good agreement between theoretical and experimentally derived hygroscopicity values will indicate that the model aptly describes the observed droplet growth. In our study, most of the nano-plastic particles are smaller than 100 nm and assumed to be spherical (see Supplementary Material). Particle shape factor can be used to describe the effect of particle shape in hygroscopicity. The shape factor can be measured by a DMA and an aerodynamic aerosol classifier in parallel (Yao et al. Citation2020).
3. Results
3.1. Cloud condensation activity (s-Dc diagram)
Nano-plastics provide a surface for water to condense and can act as cloud condensation nuclei. shows the s-Dc measurements of LDPE, PVC, PET and cellulose. The lines in are predictions from traditional Köhler theory that assume complete dissolution of the aerosol solute in the water droplet. When one assumes a full dissolution of the particle volume, the diameter follows a −1.5 power law dependence (Seinfeld, Pandis, and Noone Citation1998) and the single parameter hygroscopicity is size-independent (Petters and Kreidenweis Citation2008). The red line is the prediction for (NH4)2SO4. The black solid line is for a general polymer with molecular weight near 100,000 g mol−1 and unity density. In , nano-plastic particles (LDPE, PET and PVC) smaller than 100 nm activate and grow into droplets when the supersaturation is over 0.3%. The three plastics are less hygroscopic than ammonium sulfate (the solid red line) but more hygroscopic than the traditional prediction for polymers (the solid black line) (Medronho et al. Citation2012). In , cellulose leaches activate later (at larger sizes under a constant supersaturation) and requires more water vapor to form a droplet than the hydrophobically-classified plastics. The leaches from anthropogenic plastic are more hygroscopic than the natural polymer cellulose when exposed to water vapor.
Figure 1. The CCN activation of PVC (open squares), PET (pink triangles), LDPE (open circles) and cellulose (green triangles). The solid lines are the traditional Köhler theory predictions that assume complete dissolution of the aerosol in the droplet growth mechanism. The solid red line is representative of inorganic ammonium sulfate with (Kreidenweis and Asa-Awuku Citation2013; Petters and Kreidenweis Citation2007). The solid black line (
) is the CCN activity prediction of a polymer with molecular weight of 100,000 g mol−1. The CCN activity of LDPE, PET and PVC is noticeably more hygroscopic than measured cellulose particles or the predicted polymeric material (solid black line).
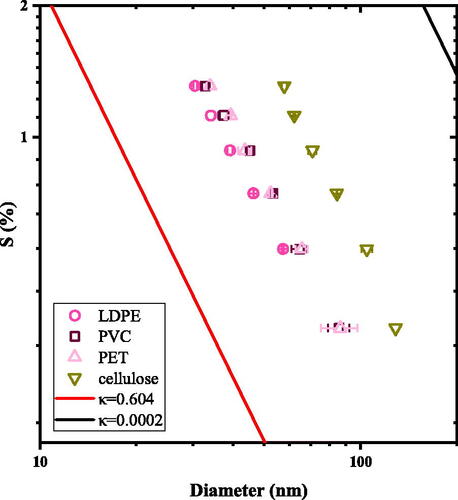
3.2. The hygroscopicity of nano-plastics
Traditional hygroscopicity assumes a thermodynamically ideal solution. The intrinsic hygroscopicity is the ratio of molecular volume of the solute and water molecules (Sullivan et al. Citation2009). Theoretically, the intrinsic hygroscopicity for polymers with an infinitely large molecular weight is zero. shows that the experimental data do not agree with the hygroscopicity prediction of large-molecular weight polymers ∼ 0. Thus these nano-plastics are either water-soluble oligomers or polymeric solid particles but with strong adsorption properties. Different approaches must be utilized. shows the derived using experimental data in . Size dependences are negligible in
for LDPE, PVC and PET. Notice that the theoretical prediction in is
assuming the molecular weight derived from FHK.
will be zero for a polymer with infinity large molecular weight.
Figure 2. The hygroscopicity of LDPE (open circle), PET (pink triangle), PVC (open square) and cellulose (green triangle) derived from S-D measurement using (a) traditional hygroscopicity theory (b) FHK (c) FHH-AT. Symbols are derived from data presented in . Solid lines are respective predictions of hygroscopicity assuming different mechanisms.
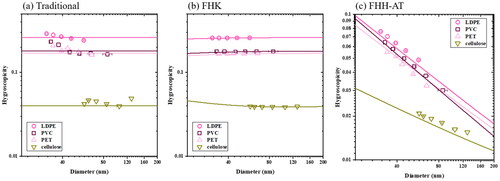
Molecular weight is an important factor for water uptake if these nano-plastics are water-soluble oligomers. However, the molecular weights of these nano-plastics are unknown. Assumptions must be made. To simplify the condition, we assume an ideal mixing in Flory-Huggins theory (Flory Citation1942). The water-polymer affinity can be ignored, which is
becomes a constant independent to the dry diameter which equals to
(see EquationEquation (2)
(2)
(2) ). In we demonstrated the FHK derived molecular weight of LDPE, PET, PVC and cellulose and reported the corresponding
The
agree well with the theoretical prediction (see ). There are 3 ∼ 5 units repeated for the leaches of LDPE, PVC, and cellulose. The derived molecular weight of PET leaches is smaller than a single unit of PET (C10H8O4, 192 g mol−1). PET is a copolymer synthesized from terephthalic acid and ethylene glycol (Biermann et al. Citation2021). The molecular weight of terephthalic acid and ethylene glycol are 166 g mol−1 and 62 g mol−1 respectively. The nano-plastics leaching from PET can potentially be a mixing of its monomers. It should be noted that
of these hydrophobic oligomers may be larger than 0.5. Hence the derived molecular weights are expected to be smaller for the same hygroscopicity. The high hygroscopicity of the hydrophobic plastic compounds could be caused by an adsorption contribution.
Table 1. Particle hygroscopicity and fitting parameters of LDPE, PET, PET and cellulose.
It is hard to tell if the nano-plastic leaches are fully water-soluble oligomers without solid fraction. In Figure S1, we not only found homogeneous spheres but also saw particles aggregation around 100 nm and 200 nm using TEM. In , intrinsic hygroscopicity decreases with size. The size dependence behavior in hygroscopicity implies the CCN can be non-fully dissolution compounds (Gohil et al. Citation2022). As a result, adsorption can still play an important role in water uptake mechanism. values are derived from EquationEquation (3)
(3)
(3) with the s-Dc data in the . shows the FHH-AT-hygroscopicity of LDPE, PET, PVC and cellulose. shows the empirical fitting
and
of LDPE, PET, PVC and cellulose. LDPE, PET, PVC and cellulose are assumed to be completely insoluble in water (Karim et al. Citation2022; Maláč, Altmann, and Zelinger Citation1970; McCall et al. Citation1984; Medronho et al. Citation2012) but water can condense on the particle surface. Among all three plastics, LDPE is more hygroscopic than PVC and PET (). Cellulose is also effectively water-insoluble (Medronho et al. Citation2012). However, cellulose is noticeably less hygroscopic. On average, the overall hygroscopicity is ∼0.05 for the nano-plastic material. also shows that hygroscopicity is size dependent and that measured
and predicted
values agree well. Small particles of a given composition are more likely to be incorporated into clouds than larger plastic particles. Furthermore, the hygroscopicity of micron and larger sized particles approaches zero. The adsorption mechanism shows that size and total surface area play the important roles of water uptake in the nano range.
In , we also provide the reported densities of the LDPE, PVC, PET and cellulose. Considering a spherical particle with the same volume, the difference in solute density and the density of the surrounding air modifies buoyancy forces acting on the particle. Thus, plastic particles like LDPE, PVC and PET with lower density can potentially travel to places further than a cellulose particle with larger solute density. Interestingly, the hygroscopicity of LDPE, PVC and PET are higher than cellulose, as well. In other words, the nano-plastic particles are more likely to be incorporated into clouds (via a wet route) and may also be transported further distances (with more boyouancy). The consideration of particle hygroscopicity and particle density supports theories that plastic particles can transfer to areas far from human activity and can then precipitate in cold environments.
4. Implication and conclusion
In this work, we measure the CCN activity and estimate the hygroscopicity of three anthropogenic nano-particles – LDPE, PVC and PET. The CCN activity and the hygroscopicity are compared with a natural polymer, cellulose. Cellulose is also the main component of paper materials used as a natural alternative to plastic. Traditional Köhler theory predicts a zero hygroscopicity for polymer and water-insoluble particles. However, in this study we show that nano-plastic particles are more hygroscopic than assumed with traditional full dissolution droplet activation theory. The nano-plastic leaches could be oligomers with three to five repeated units or solid particles with strong adsorption behavior. The nano-plastic leaches can also be more hygroscopic than nanocellulose due to their small molecular weight. Oligomers entering the environment could still polymerize (Kalberer et al. Citation2004). Plastics, like other nano-sized aerosol compositions, can potentially travel to the remote cold areas like Antarctic (Munari et al. Citation2017) and Arctic (Cózar et al. Citation2017). This work quantifies the propensity nano-plastics to act as cloud condensation nuclei. Water may readily be taken-up on these particles in saturated environments. We used traditional Köhler theory, FHK and FHH-AT to predict the hygroscopicity of nano-plastics. Plastics are considered hydrophobic but the leaching materials can be considerably hygroscopic at nano-sizes. FHH-AT, FHK and even the ideal hygroscopicity model shows a non-zero hygroscopicity of anthropogenic plastic leaches. The non-zero hygroscopic behavior is evidence that wet deposition is a plausible and significant route of nano-plastics to the earth surface. Furthermore, the hygroscopicity values quantified for nano-plastics by size in this work is important. Size dependence in the intrinsic hygroscopicity indicates a potential adsorption contribution in water uptake mechanism. Nano-plastics can provide an adsorptive core and coated with the secondary organic aerosols (Raincrow, Al-Mashala, and Schnitzler Citation2024) or inorganic salt (Petters et al. Citation2023) and grow under sub-saturated environment. Adsorbed water on particle surfaces will likely provide another route for ice particles immersion freezing mechanisms (Demott et al. Citation2015; Laaksonen et al. Citation2016; B. Wang et al. Citation2012). Wlasits et al. shows that PET particles smaller than 10 nm can readily activated under supersaturated environment as well, which provide evidence of the importance of size in adsorption mechanism (Wlasits, Konrat, and Winkler Citation2023). Our findings reported will be useful in the estimation of lifetime and transport of nano-plastic leaches in the atmosphere and subsequent climatic and health effects.
Author contributions
CNM generated, collected, and analyzed aerosol data and PAS data. KG contributed the theory development and analysis. DR contributed TEM images and analysis. All authors contributed to the writing and preparation of this manuscript.
Supplemental Material
Download MS Word (1.1 MB)Acknowledgments
The authors would like to thank Drs. George Mulholland and James Radney, for their conversations and feedback on ideas presented in this work. The authors acknowledge the financial support from the National Science Foundation (NSF). Any opinions, findings, and conclusions or recommendations expressed in this material are those of the author(s) and do not necessarily reflect the views of the National Science Foundation.
Data availability statement
Data will be made available upon request.
Disclosure statement
No potential conflict of interest was reported by the author(s).
Additional information
Funding
References
- Aeschlimann, M., G. Li, Z. A. Kanji, and D. M. Mitrano. 2022. Potential impacts of atmospheric microplastics and nanoplastics on cloud formation processes. Nat. Geosci. 15 (12):967–75. doi: 10.1038/s41561-022-01051-9.
- Allen, S., D. Allen, K. Moss, G. Le Roux, V. R. Phoenix, and J. E. Sonke. 2020. Examination of the ocean as a source for atmospheric microplastics. PLoS One. 15 (5):e0232746. doi: 10.1371/JOURNAL.PONE.0232746.
- Allen, S., D. Allen, V. R. Phoenix, G. Le Roux, P. Durántez Jiménez, A. Simonneau, S. Binet, and D. Galop. 2019. Atmospheric transport and deposition of microplastics in a remote mountain catchment. Nat. Geosci. 12 (5):339–44. doi: 10.1038/s41561-019-0335-5.
- Andrady, A. L. 2011. Microplastics in the marine environment. Mar. Pollut. Bull. 62 (8):1596–605. doi: 10.1016/j.marpolbul.2011.05.030.
- Bergmann, M., S. Mützel, S. Primpke, M. B. Tekman, J. Trachsel, and G. Gerdts. 2019. White and wonderful? Microplastics prevail in snow from the Alps to the Arctic. Sci. Adv. 5 (8):eaax1157. doi: 10.1126/sciadv.aax1157.
- Biermann, L., E. Brepohl, C. Eichert, M. Paschetag, M. Watts, and S. Scholl. 2021. Development of a continuous PET depolymerization process as a basis for a back-to-monomer recycling method. Green Process. Synth. 10 (1):361–73. doi: 10.1515/GPS-2021-0036/MACHINEREADABLECITATION/RIS.
- Cózar, A., E. Martí, C. M. Duarte, J. García-de-Lomas, E. Van Sebille, T. J. Ballatore, V. M. Eguíluz, J. Ignacio González-Gordillo, M. L. Pedrotti, F. Echevarría, et al. 2017. The Arctic Ocean as a dead end for floating plastics in the North Atlantic branch of the Thermohaline Circulation. Sci. Adv. 3 (4):e1600582. doi: 10.1126/sciadv.1600582.
- Demott, P. J., A. J. Prenni, G. R. McMeeking, R. C. Sullivan, M. D. Petters, Y. Tobo, M. Niemand, O. Möhler, J. R. Snider, Z. Wang, et al. 2015. Integrating laboratory and field data to quantify the immersion freezing ice nucleation activity of mineral dust particles. Atmos. Chem. Phys. 15 (1):393–409. doi: 10.5194/acp-15-393-2015.
- Fan, W., J. A. Salmond, K. N. Dirks, P. C. Sanz, G. M. Miskelly, and J. D. Rindelaub. 2022. Evidence and mass quantification of atmospheric microplastics in a Coastal New Zealand City. Environ. Sci. Technol. 56 (24):17556–68. doi: 10.1021/ACS.EST.2C05850.
- Filipe, V., A. Hawe, and W. Jiskoot. 2010. Critical evaluation of nanoparticle tracking analysis (NTA) by NanoSight for the measurement of nanoparticles and protein aggregates. Pharm. Res. 27 (5):796–810. doi: 10.1007/s11095-010-0073-2.
- Flory, P. J. 1942. The thermodynamics of high polymer solutions. V. Phase equilibria in the ternary system: Polymer 1-polymer 2-solvent. Statistical Mechanics of Cross-Linked Polymer Networks II. Swelling. J. Chem. Phys. 10 (1):51–61. doi: 10.1063/1.1723621.
- Gillibert, R., G. Balakrishnan, Q. Deshoules, M. Tardivel, A. Magazzù, M. G. Donato, O. M. Maragò, M. Lamy de La Chapelle, F. Colas, F. Lagarde, et al. 2019. Raman tweezers for small microplastics and nanoplastics identification in seawater. Environ. Sci. Technol. 53 (15):9003–13. doi: 10.1021/acs.est.9b03105.
- Gohil, K., R. Barrett, D. Rastogi, C.-N. Mao, Q. Yao, and A. Asa-Awuku. 2023. Solubility considerations for cloud condensation nuclei (CCN) activity analysis of pure and mixed black carbon species. Phys. Chem. A. 127 (17):3873–82. doi:10.1021/ACS.JPCA.2C08585.
- Gohil, K., C.-N. Mao, D. Rastogi, C. Peng, M. Tang, and A. Asa-Awuku. 2022. Hybrid water adsorption and solubility partitioning for aerosol hygroscopicity and droplet growth. Atmos. Chem. Phys. 22 (19):12769–87. doi: 10.5194/acp-22-12769-2022.
- Haegerbaeumer, A., M. T. Mueller, H. Fueser, and W. Traunspurger. 2019. Impacts of micro- and nano-sized plastic particles on benthic invertebrates: A literature review and gap analysis. Front. Environ. Sci. 7:17. doi: 10.3389/fenvs.2019.00017.
- Hiranuma, N., M. Kohn, M. S. Pekour, D. A. Nelson, J. E. Shilling, and D. J. Cziczo. 2011. Droplet activation, separation, and compositional analysis: Laboratory studies and atmospheric measurements. Atmos. Meas. Tech. 4 (10):2333–43. doi: 10.5194/amt-4-2333-2011.
- Ivleva, N. P. 2021. Chemical analysis of microplastics and nanoplastics: Challenges, advanced methods, and perspectives. Chem. Rev. 121 (19):11886–936. doi: 10.1021/ACS.CHEMREV.1C00178/ASSET/IMAGES/LARGE/CR1C00178_0015.JPEG.
- Kalberer, M., D. Paulsen, M. Sax, M. Steinbacher, J. Dommen, A. S. H. Prevot, R. Fisseha, E. Weingartner, V. Frankevich, R. Zenobi, et al. 2004. Identification of polymers as major components of atmospheric organic aerosols. Science 303 (5664):1659–62. doi: 10.1126/SCIENCE.1092185.
- Karim, S. S., S. Farrukh, T. Matsuura, M. Ahsan, A. Hussain, S. Shakir, L. F. Chuah, M. Hasan, and A. Bokhari. 2022. Model analysis on effect of temperature on the solubility of recycling of Polyethylene Terephthalate (PET) plastic. Chemosphere 307 (Pt 3):136050. doi: 10.1016/J.CHEMOSPHERE.2022.136050.
- Kreidenweis, S. M., and A. Asa-Awuku. 2013. Aerosol hygroscopicity: Particle water content and its role in atmospheric processes. In Treatise on geochemistry, eds. H. D. Holland and K. K. Turekian, 2nd ed., 331–61. Elsevier Science. doi: 10.1016/B978-0-08-095975-7.00418-6.
- Kumar, P., A. Nenes, and I. N. Sokolik. 2009. Importance of adsorption for CCN activity and hygroscopic properties of mineral dust aerosol. Geophys. Res. Lett. 36 (24):L24804. doi:10.1029/2009GL040827.
- Laaksonen, A., J. Malila, A. Nenes, H. M. Hung, and J. P. Chen. 2016. Surface fractal dimension, water adsorption efficiency and cloud nucleation activity of insoluble aerosol. Sci. Rep. 6 (1):25504. doi: 10.1038/srep25504.
- Lai, Y., L. Dong, Q. Li, P. Li, Z. Hao, S. Yu, and J. Liu. 2021. Counting nanoplastics in environmental waters by single particle inductively coupled plasma mass spectroscopy after cloud-point extraction and in situ labeling of gold nanoparticles. Environ. Sci. Technol. 55 (8):4783–91. doi: 10.1021/ACS.EST.0C06839/SUPPL_FILE/ES0C06839_SI_001.PDF.
- Lehmann, M., L. M. Oehlschlägel, F. P. Häusl, A. Held, and S. Gekle. 2021. Ejection of marine microplastics by raindrops: A computational and experimental study. Micropl&Nanopl. 1 (1):1–19. doi: 10.1186/s43591-021-00018-8.
- Ma, Y., A. Huang, S. Cao, F. Sun, L. Wang, H. Guo, and R. Ji. 2016. Effects of nanoplastics and microplastics on toxicity, bioaccumulation, and environmental fate of phenanthrene in fresh water. Environ. Pollut. 219:166–73. doi: 10.1016/j.envpol.2016.10.061.
- Maláč, J., V. Altmann, and J. Zelinger. 1970. Properties of PVC. II. Properties of PVC compounds with solvents. J. Appl. Polym. Sci. 14 (1):161–73. doi: 10.1002/app.1970.070140116.
- Mao, C.-N., K. Gohil, and A. A. Asa-Awuku. 2022. A single-parameter hygroscopicity model for functionalized insoluble aerosol surfaces. Atmos. Chem. Phys. 22 (19):13219–28. doi: 10.5194/acp-22-13219-2022.
- Mao, C. N., K. A. Malek, and A. Asa-Awuku. 2021. Hygroscopicity and the water-polymer interaction parameter of nano-sized biodegradable hydrophilic substances. Aerosol Sci. Technol. 55 (10):1115–24. doi: 10.1080/02786826.2021.1931012.
- Materić, D., A. Kasper-Giebl, D. Kau, M. Anten, M. Greilinger, E. Ludewig, E. Van Sebille, T. Röckmann, and R. Holzinger. 2020. Micro-and nanoplastics in alpine snow: A new method for chemical identification and (semi)quantification in the nanogram range. Environ. Sci. Technol. 54 (4):2353–9. doi: 10.1021/ACS.EST.9B07540/SUPPL_FILE/ES9B07540_SI_005.ZIP.
- McCall, D. W., D. C. Douglass, L. L. Blyler, G. E. Johnson, L. W. Jelinski, and H. E. Bair. 1984. Solubility and diffusion of water in low-density polyethylene. Macromolecules 17 (9):1644–9. doi: 10.1021/ma00139a001.
- Medronho, B., A. Romano, M. G. Miguel, L. Stigsson, and B. Lindman. 2012. Rationalizing cellulose (in)solubility: Reviewing basic physicochemical aspects and role of hydrophobic interactions. Cellulose 19 (3):581–7. doi: 10.1007/s10570-011-9644-6.
- Moore, R. H., A. Nenes, and J. Medina. 2010. Scanning mobility CCN analysis—a method for fast measurements of size-resolved CCN distributions and activation kinetics. Aerosol. Sci. Technol. 44 (10):861–71. doi: 10.1080/02786826.2010.498715.
- Munari, C., V. Infantini, M. Scoponi, E. Rastelli, C. Corinaldesi, and M. Mistri. 2017. Microplastics in the sediments of Terra Nova Bay (Ross Sea, Antarctica). Mar. Pollut. Bull. 122 (1-2):161–5. doi: 10.1016/j.marpolbul.2017.06.039.
- Petters, M. D., and S. M. Kreidenweis. 2007. A single parameter representation of hygroscopic growth and cloud condensation nucleus activity. Atmos. Chem. Phys. 7 (8):1961–71. doi: 10.5194/acp-7-1961-2007.
- Petters, M. D., and S. M. Kreidenweis. 2008. A single parameter representation of hygroscopic growth and cloud condensation nucleus activity - part 2: Including solubility. Atmos. Chem. Phys. 8 (20):6273–9. doi: 10.5194/ACP-8-6273-2008.
- Petters, S. S., E. R. Kjærgaard, F. Hasager, A. Massling, M. Glasius, and M. Bilde. 2023. Morphology and hygroscopicity of nanoplastics in sea spray. Phys. Chem. Chem. Phys. 25 (47):32430–42. doi: 10.1039/D3CP03793B.
- Prata, J. C. 2018. Airborne microplastics: Consequences to human health? Environ. Pollut. 234:115–26. doi: 10.1016/j.envpol.2017.11.043.
- Raincrow, K. L., H. H. Al-Mashala, and E. G. Schnitzler. 2024. Partitioning of secondary organic aerosol onto nanoplastics leading to hygroscopic partially-engulfed particles. Environ. Sci. Atmos. 4 (1):9–17. doi: 10.1039/D3EA00103B.
- Roberts, G. C., and A. Nenes. 2005. A continuous-flow streamwise thermal-gradient CCN chamber for atmospheric measurements. Aerosol Sci. Technol. 39 (3):206–21. doi: 10.1080/027868290913988.
- Seinfeld, J. H., S. N. Pandis, and K. Noone. 1998. Atmospheric chemistry and physics: From air pollution to climate change. Phys. Today 51 (10):88–90. doi: 10.1063/1.882420.
- Shi, C., M. Wang, Z. Wang, G. Qu, W. Jiang, X. Pan, and M. Fang. 2023. Oligomers from the synthetic polymers: Another potential iceberg of new pollutants. Environ. Health 1 (4):228–35. doi: 10.1021/envhealth.3c00086.
- Sökmen, T. Ö., E. Sulukan, M. Türkoğlu, A. Baran, M. Özkaraca, and S. B. Ceyhun. 2020. Polystyrene nanoplastics (20 nm) are able to bioaccumulate and cause oxidative DNA damages in the brain tissue of zebrafish embryo (Danio rerio). Neurotoxicology 77:51–9. doi: 10.1016/j.neuro.2019.12.010.
- Sorjamaa, R., and A. Laaksonen. 2007. The effect of H2O adsorption on cloud drop activation of insoluble particles: A theoretical framework. Atmos. Chem. Phys. 7 (24):6175–80. doi: 10.5194/ACP-7-6175-2007.
- Sullivan, R. C., M. J. K. Moore, M. D. Petters, S. M. Kreidenweis, G. C. Roberts, and K. A. Prather. 2009. Effect of chemical mixing state on the hygroscopicity and cloud nucleation properties of calcium mineral dust particles. Atmos. Chem. Phys. 9 (10):3303–16. doi:10.5194/acp-9-3303-2009.
- Tang, M., C. K. Chan, Y. J. Li, H. Su, Q. Ma, Z. Wu, G. Zhang, Z. Wang, M. Ge, M. Hu, et al. 2019. A review of experimental techniques for aerosol hygroscopicity studies. Atmos. Chem. Phys. 19 (19):12631–86. doi: 10.5194/acp-19-12631-2019.
- Ter Halle, A., L. Jeanneau, M. Martignac, E. Jardé, B. Pedrono, L. Brach, and J. Gigault. 2017. Nanoplastic in the north Atlantic subtropical gyre. Environ. Sci. Technol. 51 (23):13689–97. doi: 10.1021/acs.est.7b03667.
- Trainic, M., J. M. Flores, I. Pinkas, M. L. Pedrotti, F. Lombard, G. Bourdin, G. Gorsky, E. Boss, Y. Rudich, A. Vardi, et al. 2020. Airborne microplastic particles detected in the remote marine atmosphere. Commun. Earth Environ. 1 (1):1–9. doi: 10.1038/s43247-020-00061-y.
- Wang, B., A. T. Lambe, P. Massoli, T. B. Onasch, P. Davidovits, D. R. Worsnop, and D. A. Knopf. 2012. The deposition ice nucleation and immersion freezing potential of amorphous secondary organic aerosol: Pathways for ice and mixed-phase cloud formation. J. Geophys. Res. 117 (D16):16209. doi: 10.1029/2012JD018063.
- Wang, S. C., and R. C. Flagan. 1990. Scanning electrical mobility spectrometer. Aerosol Sci. Technol. 13 (2):230–40. doi: 10.1080/02786829008959441.
- Wang, Z., N. K. Saadé, and P. A. Ariya. 2021. Advances in ultra-trace analytical capability for micro/nanoplastics and water-soluble polymers in the environment: Fresh falling urban snow. Environ. Pollut. 276:116698. doi: 10.1016/J.ENVPOL.2021.116698.
- Williams, J., M. De Reus, R. Krejci, H. Fischer, and J. Ström. 2002. Application of the variability-size relationship to atmospheric aerosol studies: Estimating aerosol lifetimes and ages. Atmos. Chem. Phys. 2 (2):133–45. doi: 10.5194/acp-2-133-2002.
- Wlasits, P. J., R. Konrat, and P. M. Winkler. 2023. Heterogeneous nucleation of supersaturated water vapor onto sub-10 nm nanoplastic particles. Environ. Sci. Technol. 57 (4):1584–91. doi: 10.1021/ACS.EST.2C07643/ASSET/IMAGES/LARGE/ES2C07643_0007.JPEG.
- Yang, T., Y. Xu, G. Liu, and B. Nowack. 2024. Oligomers are a major fraction of the submicrometre particles released during washing of polyester textiles. Nat. Water 2 (2):151–60. doi: 10.1038/s44221-023-00191-5.
- Yao, Q., A. Asa-Awuku, C. D. Zangmeister, and J. G. Radney. 2020. Comparison of three essential sub-micrometer aerosol measurements: Mass, size and shape. Aerosol Sci. Technol. 54 (10):1197–209. doi: 10.1080/02786826.2020.1763248.
- Zangmeister, C. D., J. G. Radney, K. D. Benkstein, and B. Kalanyan. 2022. Common single-use consumer plastic products release trillions of sub-100 nm nanoparticles per liter into water during normal use. Environ. Sci. Technol. 56 (9):5448–55. doi: 10.1021/ACS.EST.1C06768.