Abstract
Background
Mitophagy and ferroptosis occur in intracerebral haemorrhage (ICH) but our understanding of mitophagy and ferroptosis-related genes remains incomplete.
Aim
This study aims to identify shared ICH genes for both processes.
Methods
ICH differentially expressed mitophagy and ferroptosis-related genes (DEMFRGs) were sourced from the GEO database and literature. Enrichment analysis elucidated functions. Hub genes were selected via STRING, MCODE, and MCC algorithms in Cytoscape. miRNAs targeting hubs were predicted using miRWalk 3.0, forming a miRNA-hub gene network. Immune microenvironment variances were assessed with MCP and TIMER. Potential small molecules for ICH were forecasted via CMap database.
Results
64 DEMFRGs and ten hub genes potentially involved in various processes like ferroptosis, TNF signalling pathway, MAPK signalling pathway, and NF-kappa B signalling pathway were discovered. Several miRNAs were identified as shared targets of hub genes. The ICH group showed increased infiltration of monocytic lineage and myeloid dendritic cells compared to the Healthy group. Ten potential small molecule drugs (e.g. Zebularine, TWS-119, CG-930) were predicted via CMap.
Conclusion
Several shared genes between mitophagy and ferroptosis potentially drive ICH progression via TNF, MAPK, and NF-kappa B pathways. These results offer valuable insights for further exploring the connection between mitophagy, ferroptosis, and ICH.
1. Introduction
Intracerebral haemorrhage (ICH) is a severe cerebrovascular disease that occurs when blood vessels rupture or become damaged within the brain, resulting in blood flow into brain tissue or ventricles and posing a serious threat to the patient’s health and life (Li et al. Citation2023). Clinical manifestations of ICH typically include sudden severe headaches, vomiting, and neurological dysfunction (Chen and Wang Citation2022; Zhou et al. Citation2022). Risk factors for ICH include hypertension, amyloidosis, vasculitis, drug abuse, coagulation disorders, and genetic factors (Zhou et al. Citation2022). Although various treatment methods such as surgery, drug therapy, and clinical monitoring have contributed to improving the survival of ICH patients (Bhatia et al. Citation2018; Gil-Garcia et al. Citation2022; Yu et al. Citation2023), ICH still exhibits high disability and mortality rates (Zheng et al. Citation2016). Currently, globally, there are approximately 24.6 cases of ICH per 100,000 people per year, and this trend is on the rise (Andrews et al. Citation2012; Ziai and Carhuapoma Citation2018; Bao et al. Citation2020). Therefore, a comprehensive investigation into the complex pathogenesis of ICH is crucial for improving the survival outcomes of ICH patients.
Previous studies have found that inflammation and immunity play a crucial role in the pathogenesis of ICH (Teng et al. Citation2009). After cerebral haemorrhage, immune cells such as neutrophils are the first to be recruited and enter the brain tissue from the blood to form inflammatory infiltration while secreting extracellular traps to participate in the inflammatory response and brain injury after ICH (Liu et al. Citation2023). In addition, some cytokines and cell surface molecules, such as transforming growth factor beta (TGF-β) and interleukin 10 (IL-10), and other characteristics of accumulation can regulate inflammation, and relieve inflammation and brain injury (Taylor et al. Citation2017; Ziai et al. Citation2021). Therefore, an in-depth understanding of the relationship between cerebral haemorrhage and immunity may be helpful to study the mechanism of ICH brain injury.
Dysfunctional or damaged mitochondria can lead to serious consequences and even cell death (Li, Gao, et al. Citation2022). Therefore, maintaining mitochondrial homeostasis is essential for cell function. Mitochondrial autophagy is a special autophagy mode that can selectively degrade damaged mitochondria and maintain mitochondrial homeostasis (Onishi et al. Citation2021). In addition, previous studies have found that mitochondrial autophagy is particularly important for cardiovascular homeostasis in health and disease (Bravo-San Pedro et al. Citation2017). For example, Chen et al. (Citation2024) found that mitochondrial autophagy and mitochondrial dysfunction are closely related to the pathophysiology of ICH. On the one hand, mitochondrial dysfunction steadily increases with the progression of ICH, and on the other hand, enhancing mitochondrial autophagy will help reduce secondary brain damage caused by mitochondrial dysfunction after ICH. In addition, it has been found that inhibition of mitochondrial autophagy can regulate the immune response of ICH and enhance its inflammatory response, thus aggravating ICH injury (Zheng et al. Citation2021). Thus, targeting mitochondrial autophagy is expected to be an effective and promising therapeutic strategy for ICH.
Ferroptosis, a newly discovered form of cell death, has been shown to play an important role in various diseases, such as cancer, neurodegeneration, and ischaemic organ injury (Li et al. Citation2020; Liang et al. Citation2022). Recent pathological studies of ICH have found that ferroptosis and iron metabolism play a crucial role in the progression of ICH (Yang et al. Citation2021). For example, Sun et al. (Citation2022) found that excessive iron released from haemoglobin after ICH induces Fenton reactions and ferroptosis, leading to brain death. Inhibition of ferroptosis after ICH holds promise for the treatment of ICH and improvement of prognosis. Similarly, Zhou et al. (Citation2023) discovered that inhibition of oxidative stress and ferroptosis significantly improves the prognosis of ICH. Although there is some controversy regarding the relationship between mitochondria and ferroptosis, numerous studies suggest a complex interplay between ferroptosis and mitochondria as well as mitophagy (Gan, Citation2021). For example, Gao et al. (Citation2019) demonstrated that mitochondria play a crucial role in cysteine deprivation-induced ferroptosis, as cells depleted of mitochondria (via mitophagy) exhibit significantly reduced ferroptosis. Similarly, Lin et al. (Citation2023) found that mitophagy prevents cisplatin-induced acute kidney injury by reducing ferroptosis in renal tubular epithelial cells. Currently, the relationship between mitophagy, ferroptosis, and ICH remains unknown and requires further research for a comprehensive understanding of their mechanisms and interactions.
In this study, we collected ICH-related genes, mitophagy-related genes (MRGs), and ferroptosis-related genes (FRGs) from public databases. Through Venn analysis, we identified the shared disease genes that respond to both mitophagy and ferroptosis in ICH. Subsequently, multiple hub genes were identified through the PPI network and Cytoscape software algorithms, and an interaction network between miRNAs and hub genes was constructed. Additionally, we explored the immune landscape differences between the Healthy group and the ICH group and predicted potential small-molecule drugs. Overall, this study aimed to investigate the relationship between mitophagy, ferroptosis, and ICH, providing insights for identifying potential therapeutic targets for ICH.
2. Materials and methods
2.1. Acquisition of public data
Eleven microarray datasets from the GSE24265 dataset were downloaded from the GEO (https://www.ncbi.nlm.nih.gov/) database and used as the training set for ICH. The dataset included samples from peri-hematoma tissue (n = 4), and corresponding contralateral white and gray matter tissue (n = 7). The GSE149317 dataset was downloaded from the GEO database and used as the validation set for ICH. MRGs were integrated from multiple publications (Zhuo et al. Citation2021; Dai et al. Citation2022; Chen et al. Citation2023; Tang et al. Citation2023). FRGs were obtained from the FerrDB (http://www.zhounan.org/ferrdb/legacy/operations/download.html).
2.2. Differential gene enrichment analysis
The limma R package was used to perform differential expression analysis on the training set samples, with a threshold of P-value <.05 and |log(FC)| > 1 to identify differentially expressed genes (DEGs) related to ICH. Subsequently, the intersection of DEGs, MRGs, and FRGs was taken, and the genes in the intersection were considered as differentially expressed mitophagy and ferroptosis-related genes (DEMFRGs) that responded to both mitophagy and ferroptosis in ICH. The DEMFRGs were subjected to GO and KEGG enrichment analyses using the clusterProfiler and enrichplotR packages to elucidate their potential functions.
2.3. Identification of hub genes and analysis of diagnostic performance
The DEMFRGs were input into the STRING database (https://cn.string-db.org/) to construct the PPI network of DEMFRGs with a confidence score of 0.4. Based on the results of the PPI network, the top 10 genes were calculated by implementing the MCC method into Cytoscape (3.9.1) plugins, MCODE (default parameters), and CytoHubba, to identify hub genes. The expression data of hub genes in the GSE149317 dataset were visualised using the ggplot2 R package. The ROC curve of the hub genes was generated and the area under the curve (AUC) was calculated using the pROC R package based on the data from the GSE24265 dataset to evaluate the diagnostic performance of the hub genes, which was further validated using the GSE149317 dataset.
2.4. Prediction of miRNA interaction network with hub genes
To explore potential miRNAs that targeted hub genes we utilised MiRDB of the miRWalk Database 3.0 (http://mirwalk.umm.uni-heidelberg.de/), microT of DIANA Tools (https://diana.e-ce.uth.gr/tools), and miRcode database (http://www.mircode.org/index.php) to carry out the forecast of microRNAs. Finally, the predicted results of the three databases are combined. The gene-miRNA network diagram was then constructed using Cytoscape software.
2.5. Immunoinfiltration analysis
To explore the differences in the immune microenvironment between ICH patients and normal samples, ssGSEA, CIBERSORT, MCP algorithm and xCell algorithm of the IOBR package were successively used for immune analysis.
2.6. Prediction of small molecule compounds targeting ICH
The CMap database (https://clue.io/) is a predictive small molecule and gene expression database that can be used to predict potential drugs that may reverse or induce the biological function of these genes by gene expression characteristics, and then screen for small molecule compounds that may play a role in specific diseases. In this study, the top 150 genes up-regulated in DEGs were imported into the CMap database, and the DEG list was compared with the reference dataset of the database using CMap. According to the enrichment of DEGs in the reference gene expression profile, a correlation score (−100 ∼ 100) was obtained. Based on previous research methods, we selected drugs with negative scores, that is, potential drugs that may reverse the biological function of these genes, as the final candidate drugs (Zhu et al. Citation2021).
3. Results
3.1. Identification and enrichment analysis of DEGs
A total of 449 DEGs were identified through differential analysis, including 318 upregulated DEGs and 131 downregulated DEGs (). Venn analysis revealed 64 DEMFRGs (). The 64 DEMFRGs were mainly enriched in GO terms, such as leukocyte migration, myeloid leukocyte migration, cytokine production involved in immune response, wound healing, regulation of angiogenesis, phospholipase inhibitor activity, lipase inhibitor activity, protease binding, cytokine activity, integrin binding, and phosphatidylinositol phosphate binding (). Additionally, the DEMFRGs were enriched in KEGG pathways such as the NOD-like receptor signalling pathway, ferroptosis, IL-17 signalling pathway, HIF-1 signalling pathway, TNF signalling pathway, MAPK signalling pathway, and NF-kappa B signalling pathway ().
3.2. Identification and analysis of hub genes in DEMFRGs
By constructing the PPI network of DEMFRGs, 63 DEMFRGs and 187 potential interacting pairs were identified (). Furthermore, using the MCODE algorithm and MCC algorithm, 10 hub genes (ITGAX, IL1B, ANXA5, HMOX1, FN1, CCL2, CXCL12, STAT3, IL6, and CAV1) were identified (). The heatmap and boxplot of gene expression showed that these 10 hub genes were generally highly expressed in the ICH group (). The correlation heatmap revealed strong correlations among the 10 hub genes (). Additionally, ROC curves for the 10 hub genes were plotted using the GSE24265 dataset and the GSE149317 dataset. The results demonstrated that the ROC values of ITGAX, IL1B, ANXA5, HMOX1, FN1, CCL2, CXCL12, STAT3, IL6, and CAV1 were all greater than 0.75 in both datasets (). This finding suggested that the 10 identified hub genes had good diagnostic performance.
Figure 2. (A) PPI network of DEMFRGs. (B) PPI network of the 10 hub genes identified by the MCODE algorithm. (C) PPI network of the 10 hub genes identified by the MCC algorithm. (D) Heatmap of gene expression for the 10 hub genes in the Healthy group and ICH group. (E) Boxplot of gene expression for the 10 hub genes in the Healthy group and ICH group. *p < .05; **p < .01; ***p < .001. (F) Correlation heatmap of the 10 hub genes.
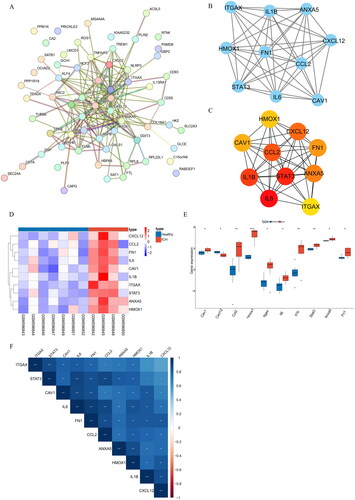
3.3. Construction of miRNA-hub gene interaction network
Based on predictions from three databases (miRWalk, DIANA Tools, and miRcode), we obtained a total of 1099 miRNAs (Supplementary Table 1). The results of the miRNA-hub gene interaction network showed that the 10 hub genes were not only regulated by multiple miRNAs but also had complex interactions among hub genes ().
3.4. ICH immune landscape assessment
To explore the differences between the Healthy group and ICH group in the immune landscape, ssGSEA, CIBERSORT, and the MCP and xCell algorithms of the IOBR package were used to analyse the differences in immune microenvironment between normal samples and ICH samples of the GSE24265 dataset, respectively. The results of ssGSEA showed that compared with the Healthy group, infiltration levels of aDCs, pDCs, Th2 cells, TIL, Treg, APC co-stimulation, CCR, HLA, inflammation-promoting, Parainflammation, T cell co-inhibition, Type I IFN response in ICH group were higher (). The analysis using CIBERSORT showed low infiltration of B cells naïve in the ICH group (). The MCP results showed that compared with the Healthy group, the infiltration levels of monocytic, myeloid dendritic cells, endothelial cells, and fibroblasts in the ICH group were higher (). The xCell analysis showed that endothelial cells, ly endothelial cells, mv endothelial cells, immune score, and microenvironment score were highly infiltrated in the ICH group (). These analyses showed that the ICH group had a higher level of immunity than the Healthy and ICH groups.
Figure 5. (A–B): Differential analysis of immune microenvironment based on ssGSEA algorithm. (C): Differential analysis of immune microenvironment based on CIBERSORT algorithm. (D): Differential analysis of immune microenvironment based on MCP algorithm. (E): Differential analysis of immune microenvironment based on xCell algorithm. *p < .05; **p < .01; ns: p > .05.
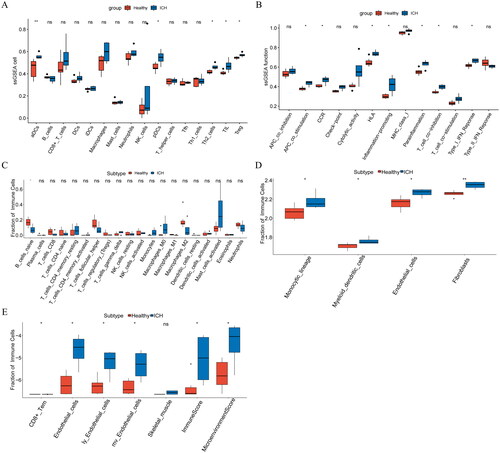
3.5. Prediction of ICH-targeted small molecule drugs using the CMap database
Through the CMap database, we predicted a total of 10 potential small molecule drugs for ICH, namely: Zebularine, TWS-119, CG-930, OM-137, tipifarnib, warfarin, SB-202190, Y-27632, PD-98059, and rilmenidine (). These small molecular compounds may exert their effects by inhibiting the activity of DNA methyltransferase, glycogen synthase kinase, JNK, Aurora kinase, Farnesyltransferase, Vitamin K, p38 MAPK, Rho-associated kinase, MEK, and activating adrenergic receptors ().
Table 1. ICH-targeted small molecule drugs predicted through cMAP database.
4. Discussion
ICH is a subtype of stroke with high incidence, disability rate, and mortality rate (Garg and Biller Citation2019; Ren et al. Citation2020). Studies have found that both mitophagy and ferroptosis can influence the progression of ICH to varying degrees (Li et al. Citation2021; Youn et al. Citation2021; Sun et al. Citation2022). However, the relationship between mitophagy and ferroptosis in ICH is currently unknown. Therefore, this study conducted an exploration of the shared gene set between ferroptosis and mitophagy in ICH, aiming to further elucidate the relationship between these processes and provide insights into potential therapeutic targets for ICH.
Through differential expression analysis and Venn analysis, we identified a total of 64 shared genes related to ferroptosis and mitophagy in ICH. The results of enrichment analysis showed that these DEMFRGs were mainly enriched in KEGG pathways such as the TNF signalling pathway, MAPK signalling pathway, and NF-kappa B signalling pathway. Tumour necrosis factor (TNF) is a key regulatory factor in the immune system and plays a dual role in promoting neurodegeneration and tissue regeneration in neurodegenerative diseases. Activation of the TNF signalling pathway can promote inflammation propagation and oxidative stress (Fischer et al. Citation2015). Liu et al. (Citation2021) found in their study on ICH that the activation of the TNF signalling pathway may play an important role in ferroptosis following ICH stroke. Mitogen-activated protein kinases (MAPKs) and nuclear factor-kappa B (NF-κB) are key factors in inflammatory signalling pathways and have a role in promoting and regulating inflammation (Haftcheshmeh et al. Citation2022). Wang, Jiao, et al. (Citation2022) found in their research that reducing the expression levels of MAPK and NF-κB under Ulinastatin (UTI) therapy may help alleviate necrotic apoptosis and neuroinflammation, thus mitigating early brain injury following ICH. Taken together, these findings suggest that genes related to mitophagy and ferroptosis may mediate the progression of ICH by regulating these signalling pathways.
By constructing the PPI network of DEMFRGs and selecting hub genes, we identified 10 hub genes (ITGAX, IL1B, ANXA5, HMOX1, FN1, CCL2, CXCL12, STAT3, IL6, and CAV1) that were generally upregulated in the ICH group. Integrin αX (ITGAX) is a member of the integrin family and is typically involved in cell-extracellular matrix interactions. It not only participates in dendritic cell angiogenesis but also mediates the occurrence of various diseases such as atherosclerosis, kidney disease, and tumors (Calvin et al. Citation1988; Wang et al. Citation2019, Citation2023; Sui et al. Citation2021). However, the role of ITGAX in ICH has not been discovered yet. Interleukin-1β (IL-1β) is a pro-inflammatory cytokine that significantly increases in the 6–72 h after ICH and serves as an important reference for ICH prevention and intervention (Zhang et al. Citation2019; Varljen et al. Citation2020). Previous studies have found that IL-1β is closely related to mitochondrial autophagy and ferroptosis. Regulation of IL-1β-induced signalling pathways can regulate the occurrence of mitochondrial autophagy (Li, Jiang, et al. Citation2022). Besides, IL-1β production can contribute to the occurrence of ferroptosis (Mo et al. Citation2021). ANXA5 is an important annexin with anti-inflammatory, anticoagulant, and anti-apoptotic effects (Burgmaier et al. Citation2014). Although the relationship between ANXA5 and ICH has not been established, previous research has found a significant role in regulating myogenesis mediated by mitophagy (Baechler et al. Citation2019). Haem oxygenase-1 (HMOX1) is a stress-inducible enzyme that catalyses the degradation of haem into carbon monoxide, iron, and biliverdin. Lack of HMOX1 can exacerbate inflammation after subarachnoid haemorrhage (LeBlanc et al. Citation2016; Deng et al. Citation2021; Dunn et al. Citation2021). Ni et al. (Citation2023) found that upregulating the expression of HMOX1 can promote the accumulation of ferrous ions and induce the occurrence of ferroptosis. In addition, early mitochondrial dysfunction and HMOX1 overactivation synergically trigger the occurrence of mitochondrial autophagy (Meyer et al. Citation2018). Fibronectin 1 (FN1) is a polymorphic gene, and previous studies have found a seven-fold increase in genetic susceptibility to intraventricular haemorrhage in premature infants with FN1 gene polymorphisms (Szpecht et al. Citation2020). IL6, CCL2, and CXCL12 are important pro-inflammatory cytokines and chemokines. Their excessive secretion and activation in ICH can effectively promote the differentiation of microglia and the activation of macrophages, stimulating the inflammatory response in ICH patients (Wang, Bian, et al. Citation2022). Signal transducer and activator of transcription 3 (STAT3) is a transcription factor that regulates various biological processes, including proliferation, metastasis, angiogenesis, immune response, and chemoresistance (Dong et al. Citation2021). Previous studies have shown that mitochondrial translocation of STAT3 inhibits oxidative stress-induced autophagy and may effectively protect mitochondria from mitochondrial autophagy degradation (You et al. Citation2015). Meanwhile, targeted regulation of STAT3 can inhibit the proliferation of cancer cells by regulating mitochondrial autophagy (Jiang et al. Citation2022). A study by Ouyang et al. (Citation2022) showed that STAT3 is a potential regulator of ferroptosis. Zhuang et al. (Citation2023) found that inhibiting the activation of STAT3 can alleviate microglia-induced neuroinflammation after ICH. Caveolin-1 (CAV1) is a key protein in caveolae. Studies in ICH have found that downregulation of CAV1 attenuates cell apoptosis in rats, promotes neuronal survival in brain tissue, and improves ICH-induced neurological dysfunction, while overexpression of CAV1 in ICH rats leads to increased cell apoptosis and neuronal loss, significantly worsening neurological dysfunction (Cao et al. Citation2023). In addition, most studies have shown that CAV1 can be used as a marker to adjust ferroptosis and its related life activities, and regulate mitochondrial autophagy (Yao et al. Citation2021; Lu et al. Citation2022; Yang et al. Citation2022; Li and Hu Citation2023). Combined with these findings, most of the hub genes identified in this study are associated with mitochondrial autophagy, ferroptosis, or ICH. Therefore, combining these analyses, we speculated that these hub genes may directly or indirectly influence mitochondrial autophagy or ferroptosis, which in turn influenced ICH disease progression. Furthermore, ROC analysis revealed that the AUC values of these hub genes were greater than 0.75 in both datasets, indicating good diagnostic performance of the 10 hub genes.
When ICH damages the brain, the immune system becomes overactive, leading to systemic inflammatory responses and immune regulation disorders, and also significantly affecting brain damage (Li and Chen Citation2023). Therefore, it is important to explore the molecular mechanism of post-ICH neuroinflammation. Neuroinflammation is mediated mainly by activation of resident immune cells, recruitment of peripheral lymphocytes, and production of other pro-inflammatory secondary messengers such as cytokines, chemokines, ROS, and mitochondrial autophagy (Vezzani et al. Citation2020). Autophagy and inflammation are thought to be the main culprits in the progression of brain injury. Therefore, the regulation of inflammation in ICH-induced secondary brain injury by autophagy may be an effective treatment for ICH-related diseases (Fu et al. Citation2022). At present, in the relevant studies of rubrospinal cord neurons after spinal cord injury (SCI), it has been found that blocking the autophagy flux (inhibiting the synthesis of AP (LC-3-positive autophagosomes)) can reduce the injury and promote the self-functional recovery of injured neurons (Bisicchia et al. Citation2017). In addition, ICH not only affects the central nervous system in a complex manner but is also regulated by multiple immune systems such as inflammatory mediators and immune effector cells (Iadecola and Anrather Citation2011; Li and Chen Citation2023). Therefore, in addition to exploring the relationship between autophagy related genes and ICH, this study also explored the immune levels in ICH. Monocytic lineage and myeloid dendritic cells are highly infiltrated immune cell types identified in the ICH group in this study. It has been shown that acute ICH rapidly activates inflammation due to inadequate perfusion, during which monocytes extensively infiltrate the brain parenchyma and mediate the loss of blood-brain barrier integrity caused by inflammation (Keep et al. Citation2014; Ritzel et al. Citation2015; Stephens et al. Citation2023). Dendritic cells (DCs), similar to monocytes, migrate to the injured brain region after ICH and participate in local inflammation (Ludewig et al. Citation2016; Stephens et al. Citation2023). The study by Mathias Gelderblom et al. (Gelderblom et al. Citation2012) found that stroke mice receiving neuroprotective treatment show reduced infiltration of dendritic cells and lymphocytes in the ischaemic hemisphere. Taken together, these findings suggest that targeting these immune cells may help alleviate the inflammatory response in ICH.
Zebularine, TWS-119, and PD-98059 are potential small-molecule drugs predicted based on the CMap database in this study. 1-beta-D-Ribofuranosyl-2(1H)-pyrimidinone (Zebularine) is a second-generation highly stable hydrophilic DNA methylation inhibitor that induces replication-dependent double-strand breaks and often acts as a stable antitumor agent. Priority target cancer cells (Orta et al. Citation2017; Marquez et al. Citation2005). 4, 6-disubstituted pyrrolopyrimidine (TWS119), a specific inhibitor of glycogen synthase kinase (GSK)-3β, increases protein levels of β-catenin and activates the Wnt signalling pathway in the ischaemic brain, and has beneficial effects on immunoinflammatory regulation and blood-brain barrier protection (Sabatino et al. Citation2016; Wang et al. Citation2016; Chen et al. Citation2018). At present, researchers have not found the targeted therapeutic effect of Zebularine and TWS-119 on ICH. However, it has been found that TWS119 may reduce tissue plasminogen activator (rtPA) -induced bleeding transformation by activating the Wnt/β-catenin pathway and reduce blood–brain barrier breakdown in ischaemic stroke (Wang et al. Citation2016). Similarly, TWS119 was found to promote neurological recovery after experimental stroke (Song et al. Citation2019). MAPK is an important signal of vascular proliferation and contraction factor, PD-98059 as a MAPK kinase inhibitor can significantly reduce the haemolysis-induced contraction and is, therefore, considered to be a potential candidate for therapeutic agents in the treatment of cerebral vasospasm (Tibbs et al. Citation2000; Zubkov et al. Citation2000). At present, studies on the efficacy of these drug candidates in ICH treatment are not yet known. However, based on the prediction of the CMap database and the existing literature, we can speculate that these small molecule compounds may play a role mainly by regulating the activity of DNA methyltransferase, glycogen synthase kinase, p38 MAPK, Rho-associated kinase, and MEK, and activating adrenergic receptors. However, as this study has not yet conducted experimental studies on the efficacy of these small molecule drugs in ICH treatment, more experimental investigations of drug effectiveness are still needed.
In conclusion, this study systematically explored the potential role of genes shared by ferroptosis and mitochondrial autophagy in ICH and predicted some hub genes with good diagnostic performance. In addition, the immune landscape of the Healthy and ICH groups was explored, and some potential small molecule drugs were predicted. The results of this study have certain significance for the preliminary disclosure of the relationship between mitochondrial autophagy and ferroptosis in ICH. However, some research deficiencies should not be ignored. For example, our findings are limited to the bioinformatics level, and there is still a lack of relevant experimental verification. More studies will be conducted in the future to further verify the findings.
Ethics approval and consent to participate
Not applicable.
Author contribution
YW conceived of the study, and participated in its design and interpretation and helped to draft the manuscript. RFW participated in the design and interpretation of the data. JZZ helped to draft the manuscript. LC performed the statistical analysis and revised the manuscript critically. All the authors read and approved the final manuscript.
Supplemental Material
Download MS Excel (100.5 KB)Disclosure statement
No potential conflict of interest was reported by the author(s).
Data availability statement
The data and materials in the current study are available from the corresponding author on reasonable request.
Additional information
Funding
References
- Andrews CM, Jauch EC, Hemphill JC, 3rd, Smith WS, Weingart SD. 2012. Emergency neurological life support: intracerebral hemorrhage. Neurocrit Care. 17 Suppl 1(S1):S37–S46. doi: 10.1007/s12028-012-9757-2.
- Baechler BL, Bloemberg D, Quadrilatero J. 2019. Mitophagy regulates mitochondrial network signaling, oxidative stress, and apoptosis during myoblast differentiation. Autophagy. 15(9):1606–1619. doi: 10.1080/15548627.2019.1591672.
- Bao WD, Zhou XT, Zhou LT, Wang F, Yin X, Lu Y, Zhu LQ, Liu D. 2020. Targeting miR-124/Ferroportin signaling ameliorated neuronal cell death through inhibiting apoptosis and ferroptosis in aged intracerebral hemorrhage murine model. Aging Cell. 19(11):e13235. doi: 10.1111/acel.13235.
- Bhatia K, Hepburn M, Ziu E, Siddiq F, Qureshi AI. 2018. Modern approaches to evacuating intracerebral hemorrhage. Curr Cardiol Rep. 20(12):132. doi: 10.1007/s11886-018-1078-4.
- Bisicchia E, Latini L, Cavallucci V, Sasso V, Nicolin V, Molinari M, D’Amelio M, Viscomi MT. 2017. Autophagy inhibition favors survival of rubrospinal neurons after spinal cord hemisection. Mol Neurobiol. 54(7):4896–4907. doi: 10.1007/s12035-016-0031-z.
- Bravo-San Pedro JM, Kroemer G, Galluzzi L. 2017. Autophagy and Mitophagy in Cardiovascular Disease. Circ Res. 120(11):1812–1824. doi: 10.1161/CIRCRESAHA.117.311082.
- Burgmaier M, Schutters K, Willems B, van der Vorst EP, Kusters D, Chatrou M, Norling L, Biessen EA, Cleutjens J, Perretti M, et al. 2014. AnxA5 reduces plaque inflammation of advanced atherosclerotic lesions in apoE(-/-) mice. J Cell Mol Med. 18(10):2117–2124. doi: 10.1111/jcmm.12374.
- Calvin S, Corrigan J, Weinstein L, Jeter M. 1988. Factor VIII: von Willebrand factor patterns in the plasma of patients with pre-eclampsia. Am J Perinatol. 5(1):29–32. doi: 10.1055/s-2007-999648.
- Cao D, Li B, Cao C, Zhang J, Li X, Li H, Yu Z, Shen H, Ye M. 2023. Caveolin-1 aggravates neurological deficits by activating neuroinflammation following experimental intracerebral hemorrhage in rats. Exp Neurol. 368:114508. doi: 10.1016/j.expneurol.2023.114508.
- Chen RY, Li DW, Xie H, Liu XW, Zhuang SY, Wu HY, Wu JJ, Sun N, Qu JW, Miao JY, et al. 2023. Gene signature and prediction model of the mitophagy-associated immune microenvironment in renal ischemia-reperfusion injury. Front Immunol. 14:1117297. doi: 10.3389/fimmu.2023.1117297.
- Chen Y, Tang W, Huang X, An Y, Li J, Yuan S, Shan H, Zhang M. 2024. Mitophagy in intracerebral hemorrhage: a new target for therapeutic intervention. Neural Regen Res. 19(2):316–323. doi: 10.4103/1673-5374.379019.
- Chen SP, Wang SJ. 2022. Pathophysiology of reversible cerebral vasoconstriction syndrome. J Biomed Sci. 29(1):72. doi: 10.1186/s12929-022-00857-4.
- Chen YQ, Zheng L, Aldarouish M, Zhou ZH, Pan N, Liu JQ, Chen FX, Wang LX. 2018. Wnt pathway activator TWS119 enhances the proliferation and cytolytic activity of human gammadeltaT cells against colon cancer. Exp Cell Res. 362(1):63–71. doi: 10.1016/j.yexcr.2017.11.003.
- Dai D, Liu L, Guo Y, Shui Y, Wei Q. 2022. A comprehensive analysis of the effects of key mitophagy genes on the progression and prognosis of lung adenocarcinoma. Cancers (Basel). 15(1):15. doi: 10.3390/cancers15010057.
- Deng X, Liang C, Qian L, Zhang Q. 2021. miR-24 targets HMOX1 to regulate inflammation and neurofunction in rats with cerebral vasospasm after subarachnoid hemorrhage. Am J Transl Res. 13(3):1064–1074.
- Dong J, Cheng XD, Zhang WD, Qin JJ. 2021. Recent update on development of small-molecule STAT3 inhibitors for cancer therapy: from phosphorylation inhibition to protein degradation. J Med Chem. 64(13):8884–8915. doi: 10.1021/acs.jmedchem.1c00629.
- Dunn LL, Kong SMY, Tumanov S, Chen W, Cantley J, Ayer A, Maghzal GJ, Midwinter RG, Chan KH, Ng MKC, et al. 2021. Hmox1 (Heme Oxygenase-1) protects against ischemia-mediated injury via stabilization of HIF-1alpha (hypoxia-inducible factor-1alpha). Arterioscler Thromb Vasc Biol. 41(1):317–330.
- Fischer R, Maier O. 2015. Interrelation of oxidative stress and inflammation in neurodegenerative disease: role of TNF. Oxid Med Cell Longev. 2015:610813–610818. doi: 10.1155/2015/610813.
- Fu K, Xu W, Lenahan C, Mo Y, Wen J, Deng T, Huang Q, Guo F, Mo L, Yan J. 2022. Autophagy regulates inflammation in intracerebral hemorrhage: enemy or friend? Front Cell Neurosci. 16:1036313. doi: 10.3389/fncel.2022.1036313.
- Gan B. 2021. Mitochondrial regulation of ferroptosis. J Cell Biol. 220(9). doi: 10.1083/jcb.202105043.
- Gao M, Yi J, Zhu J, Minikes AM, Monian P, Thompson CB, Jiang X. 2019. Role of mitochondria in ferroptosis. Mol Cell. 73(2):354–363 e3. doi: 10.1016/j.molcel.2018.10.042.
- Garg R, Biller J. 2019. Recent advances in spontaneous intracerebral hemorrhage. F1000Res. 8:8. doi: 10.12688/f1000research.16357.1.
- Gelderblom M, Leypoldt F, Lewerenz J, Birkenmayer G, Orozco D, Ludewig P, Thundyil J, Arumugam TV, Gerloff C, Tolosa E, et al. 2012. The flavonoid fisetin attenuates postischemic immune cell infiltration, activation and infarct size after transient cerebral middle artery occlusion in mice. J Cereb Blood Flow Metab. 32(5):835–843. doi: 10.1038/jcbfm.2011.189.
- Gil-Garcia CA, Flores-Alvarez E, Cebrian-Garcia R, Mendoza-Lopez AC, Gonzalez-Hermosillo LM, Garcia-Blanco MD, Roldan-Valadez E. 2022. Essential topics about the imaging diagnosis and treatment of hemorrhagic stroke: A Comprehensive Review of the 2022 AHA Guidelines. Curr Probl Cardiol. 47(11):101328. doi: 10.1016/j.cpcardiol.2022.101328.
- Haftcheshmeh SM, Abedi M, Mashayekhi K, Mousavi MJ, Navashenaq JG, Mohammadi A, Momtazi-Borojeni AA. 2022. Berberine as a natural modulator of inflammatory signaling pathways in the immune system: focus on NF-kappaB, JAK/STAT, and MAPK signaling pathways. Phytother Res. 36(3):1216–1230. doi: 10.1002/ptr.7407.
- Iadecola C, Anrather J. 2011. The immunology of stroke: from mechanisms to translation. Nat Med. 17(7):796–808. doi: 10.1038/nm.2399.
- Jiang T, Peng L, Wang Q, Huang B, Peng D, Men L, Jiang Y, Zhu M, Wang M, Lin L, et al. 2022. Cirsiliol regulates mitophagy in colon cancer cells via STAT3 signaling. Cancer Cell Int. 22(1):304. doi: 10.1186/s12935-022-02732-6.
- Keep RF, Zhou N, Xiang J, Andjelkovic AV, Hua Y, Xi G. 2014. Vascular disruption and blood-brain barrier dysfunction in intracerebral hemorrhage. Fluids Barriers Cns. 11(1):18. doi: 10.1186/2045-8118-11-18.
- LeBlanc RH, 3rd, Chen R, Selim MH, Hanafy KA. 2016. Heme oxygenase-1-mediated neuroprotection in subarachnoid hemorrhage via intracerebroventricular deferoxamine. J Neuroinflammation. 13(1):244. doi: 10.1186/s12974-016-0709-1.
- Liang D, Minikes AM, Jiang X. 2022. Ferroptosis at the intersection of lipid metabolism and cellular signaling. Mol Cell. 82(12):2215–2227. doi: 10.1016/j.molcel.2022.03.022.
- Li J, Cao F, Yin HL, Huang ZJ, Lin ZT, Mao N, Sun B, Wang G. 2020. Ferroptosis: past, present and future. Cell Death Dis. 11(2):88. doi: 10.1038/s41419-020-2298-2.
- Li X, Chen G. 2023. CNS-peripheral immune interactions in hemorrhagic stroke. J Cereb Blood Flow Metab. 43(2):185–197. doi: 10.1177/0271678X221145089.
- Li A, Gao M, Liu B, Qin Y, Chen L, Liu H, Wu H, Gong G. 2022. Mitochondrial autophagy: molecular mechanisms and implications for cardiovascular disease. Cell Death Dis. 13(5):444. doi: 10.1038/s41419-022-04906-6.
- Li L, Hu F. 2023. Mitophagy in tumor: foe or friend? Endokrynol Pol. 74(5):511–519. doi: 10.5603/ep.95652.
- Li J, Jiang M, Yu Z, Xiong C, Pan J, Cai Z, Xu N, Zhou X, Huang Y, Yang Z. 2022. Artemisinin relieves osteoarthritis by activating mitochondrial autophagy through reducing TNFSF11 expression and inhibiting PI3K/AKT/mTOR signaling in cartilage. Cell Mol Biol Lett. 27(1):62. doi: 10.1186/s11658-022-00365-1.
- Li J, Wu X, He Y, Wu S, Guo E, Feng Y, Yang J, Li J. 2021. PINK1 antagonize intracerebral hemorrhage by promoting mitochondrial autophagy. Ann Clin Transl Neurol. 8(10):1951–1960. doi: 10.1002/acn3.51425.
- Li Y, Tao C, An N, Liu H, Liu Z, Zhang H, Sun Y, Xing Y, Gao Y. 2023. Revisiting the role of the complement system in intracerebral hemorrhage and therapeutic prospects. Int Immunopharmacol. 123:110744. doi: 10.1016/j.intimp.2023.110744.
- Lin Q, Li S, Jin H, Cai H, Zhu X, Yang Y, Wu J, Qi C, Shao X, Li J, et al. 2023. Mitophagy alleviates cisplatin-induced renal tubular epithelial cell ferroptosis through ROS/HO-1/GPX4 axis. Int J Biol Sci. 19(4):1192–1210. doi: 10.7150/ijbs.80775.
- Liu J, Zhang S, Jing Y, Zou W. 2023. Neutrophil extracellular traps in intracerebral hemorrhage: implications for pathogenesis and therapeutic targets. Metab Brain Dis. 38(8):2505–2520. doi: 10.1007/s11011-023-01268-6.
- Liu T, Li X, Cui Y, Meng P, Zeng G, Wang Y, Wang Q. 2021. Bioinformatics analysis identifies potential ferroptosis key genes in the pathogenesis of intracerebral hemorrhage. Front Neurosci. 15:661663. doi: 10.3389/fnins.2021.661663.
- Lu T, Zhang Z, Pan X, Zhang J, Wang X, Wang M, Li H, Yan M, Chen W. 2022. Caveolin-1 promotes cancer progression via inhibiting ferroptosis in head and neck squamous cell carcinoma. J Oral Pathol Med. 51(1):52–62. doi: 10.1111/jop.13267.
- Ludewig P, Gallizioli M, Urra X, Behr S, Brait VH, Gelderblom M, Magnus T, Planas AM. 2016. Dendritic cells in brain diseases. Biochim Biophys Acta. 1862(3):352–367. doi: 10.1016/j.bbadis.2015.11.003.
- Marquez VE, Barchi JJ, Jr.; Kelley JA, Rao KV, Agbaria R, Ben-Kasus T, Cheng JC, Yoo CB, Jones PA. 2005. Zebularine: a unique molecule for an epigenetically based strategy in cancer chemotherapy. The magic of its chemistry and biology. Nucleosides Nucleotides Nucleic Acids. 24(5-7):305–318. doi: 10.1081/ncn-200059765.
- Meyer N, Zielke S, Michaelis JB, Linder B, Warnsmann V, Rakel S, Osiewacz HD, Fulda S, Mittelbronn M, Münch C, et al. 2018. AT 101 induces early mitochondrial dysfunction and HMOX1 (heme oxygenase 1) to trigger mitophagic cell death in glioma cells. Autophagy. 14(10):1693–1709., doi: 10.1080/15548627.2018.1476812.
- Mo Z, Xu P, Li H. 2021. Stigmasterol alleviates interleukin-1beta-induced chondrocyte injury by down-regulatingsterol regulatory element binding transcription factor 2 to regulate ferroptosis. Bioengineered. 12(2):9332–9340. doi: 10.1080/21655979.2021.2000742.
- Ni M, Zhou J, Zhu Z, Xu Q, Yin Z, Wang Y, Zheng Z, Zhao H. 2023. Shikonin and cisplatin synergistically overcome cisplatin resistance of ovarian cancer by inducing ferroptosis via upregulation of HMOX1 to promote Fe(2+) accumulation. Phytomedicine. 112:154701. doi: 10.1016/j.phymed.2023.154701.
- Onishi M, Yamano K, Sato M, Matsuda N, Okamoto K. 2021. Molecular mechanisms and physiological functions of mitophagy. Embo J. 40(3):e104705. doi: 10.15252/embj.2020104705.
- Orta ML, Pastor N, Burgos-Morón E, Domínguez I, Calderón-Montaño JM, Huertas Castaño C, López-Lázaro M, Helleday T, Mateos S. 2017. Zebularine induces replication-dependent double-strand breaks which are preferentially repaired by homologous recombination. DNA Repair (Amst)). 57:116–124. doi: 10.1016/j.dnarep.2017.07.002.
- Ouyang S, Li H, Lou L, Huang Q, Zhang Z, Mo J, Li M, Lu J, Zhu K, Chu Y, et al. 2022. Inhibition of STAT3-ferroptosis negative regulatory axis suppresses tumor growth and alleviates chemoresistance in gastric cancer. Redox Biol. 52:102317. doi: 10.1016/j.redox.2022.102317.
- Ren H, Han R, Chen X, Liu X, Wan J, Wang L, Yang X, Wang J. 2020. Potential therapeutic targets for intracerebral hemorrhage-associated inflammation: an update. J Cereb Blood Flow Metab. 40(9):1752–1768. doi: 10.1177/0271678X20923551.
- Ritzel RM, Patel AR, Grenier JM, Crapser J, Verma R, Jellison ER, McCullough LD. 2015. Functional differences between microglia and monocytes after ischemic stroke. J Neuroinflammation. 12(1):106. doi: 10.1186/s12974-015-0329-1.
- Sabatino M, Hu J, Sommariva M, Gautam S, Fellowes V, Hocker JD, Dougherty S, Qin H, Klebanoff CA, Fry TJ, et al. 2016. Generation of clinical-grade CD19-specific CAR-modified CD8+ memory stem cells for the treatment of human B-cell malignancies. Blood. 128(4):519–528. doi: 10.1182/blood-2015-11-683847.
- Song D, Zhang X, Chen J, Liu X, Xue J, Zhang L, Lan X. 2019. Wnt canonical pathway activator TWS119 drives microglial anti-inflammatory activation and facilitates neurological recovery following experimental stroke. J Neuroinflammation. 16(1):256. doi: 10.1186/s12974-019-1660-8.
- Stephens R, Grainger JR, Smith CJ, Allan SM. 2023. Systemic innate myeloid responses to acute ischaemic and haemorrhagic stroke. Semin Immunopathol. 45(3):281–294. doi: 10.1007/s00281-022-00968-y.
- Sui Y, Lu K, Fu L. 2021. Prediction and analysis of novel key genes ITGAX, LAPTM5, SERPINE1 in clear cell renal cell carcinoma through bioinformatics analysis. PeerJ. 9:e11272. doi: 10.7717/peerj.11272.
- Sun Y, Li Q, Guo H, He Q. 2022. Ferroptosis and iron metabolism after intracerebral hemorrhage. Cells. 12(1):90. doi: 10.3390/cells12010090.
- Szpecht D, Al-Saad SR, Karbowski LM, Kosik K, Kurzawińska G, Szymankiewicz M, Drews K, Seremak-Mrozikiewicz A. 2020. Role of fibronectin-1 polymorphism genes with the pathogenesis of intraventricular hemorrhage in preterm infants. Childs Nerv Syst. 36(8):1729–1736. doi: 10.1007/s00381-020-04598-3.
- Tang Y, Guo H, Chen L, Wang X, Chen Q, Gou L, Liu X, Wang X. 2023. Development and validation of a prognostic model for mitophagy-related genes in colon adenocarcinoma: a study based on TCGA and GEO databases. PLoS One. 18(4):e0284089. doi: 10.1371/journal.pone.0284089.
- Taylor RA, Chang CF, Goods BA, Hammond MD, Mac Grory B, Ai Y, Steinschneider AF, Renfroe SC, Askenase MH, McCullough LD, et al. 2017. TGF-beta1 modulates microglial phenotype and promotes recovery after intracerebral hemorrhage. J Clin Invest. 127(1):280–292. doi: 10.1172/JCI88647.
- Teng W, Wang L, Xue W, Guan C. 2009. Activation of TLR4-mediated NFkappaB signaling in hemorrhagic brain in rats. Mediators Inflamm. 2009:473276–473276. doi: 10.1155/2009/473276.
- Tibbs R, Zubkov A, Aoki K, Meguro T, Badr A, Parent A, Zhang J. 2000. Effects of mitogen-activated protein kinase inhibitors on cerebral vasospasm in a double-hemorrhage model in dogs. J Neurosurg. 93(6):1041–1047. doi: 10.3171/jns.2000.93.6.1041.
- Varljen T, Sekulovic G, Rakic O, Maksimovic N, Jekic B, Novakovic I, Damnjanovic T. 2020. Genetic variant rs16944 in IL1B gene is a risk factor for early-onset sepsis susceptibility and outcome in preterm infants. Inflamm Res. 69(2):155–157. doi: 10.1007/s00011-019-01301-4.
- Vezzani B, Carinci M, Patergnani S, Pasquin MP, Guarino A, Aziz N, Pinton P, Simonato M, Giorgi C. 2020. The dichotomous role of inflammation in the CNS: a mitochondrial point of view. Biomolecules. 10(10):1437. doi: 10.3390/biom10101437.
- Wang J, Bian L, Du Y, Wang D, Jiang R, Lu J, Zhao X. 2022. The roles of chemokines following intracerebral hemorrhage in animal models and humans. Front Mol Neurosci. 15:1091498. doi: 10.3389/fnmol.2022.1091498.
- Wang X, Guan Z, Tang W, Wang X, Xu C, Shan E, Wang W, Gao Y. 2023. PAX5/ITGAX contributed to the progression of atherosclerosis by regulation of B differentiation via TNF-alpha signaling pathway. DNA Cell Biol. 42(2):97–104. doi: 10.1089/dna.2022.0461.
- Wang L, Jiao W, Wu J, Zhang J, Tang M, Chen Y. 2022. Ulinastatin alleviates early brain injury after intracerebral hemorrhage by inhibiting necroptosis and neuroinflammation via MAPK/NF-kappaB signaling pathway. Acta Cir Bras. 37(3):e370301. doi: 10.1590/acb370301.
- Wang W, Li M, Wang Y, Li Q, Deng G, Wan J, Yang Q, Chen Q, Wang J. 2016. GSK-3beta inhibitor TWS119 attenuates rtPA-induced hemorrhagic transformation and activates the Wnt/beta-catenin signaling pathway after acute ischemic stroke in rats. Mol Neurobiol. 53(10):7028–7036. doi: 10.1007/s12035-015-9607-2.
- Wang J, Yang L, Liang F, Chen Y, Yang G. 2019. Integrin alpha x stimulates cancer angiogenesis through PI3K/Akt signaling-mediated VEGFR2/VEGF-A overexpression in blood vessel endothelial cells. J Cell Biochem. 120(2):1807–1818. doi: 10.1002/jcb.27480.
- Yang C, Han M, Li R, Zhou L, Zhang Y, Duan L, Su S, Li M, Wang Q, Chen T, et al. 2021. Curcumin nanoparticles inhibiting ferroptosis for the enhanced treatment of intracerebral hemorrhage. Int J Nanomed. 16:8049–8065. doi: 10.2147/IJN.S334965.
- Yang Y, Hong S, Lu Y, Wang Q, Wang S, Xun Y. 2022. CAV1 alleviated CaOx stones formation via suppressing autophagy-dependent ferroptosis. PeerJ. 10:e14033. doi: 10.7717/peerj.14033.
- Yao J, Zhang Y, Li M, Sun Z, Liu T, Zhao M, Li Z. 2021. Single-cell RNA-Seq reveals the promoting role of ferroptosis tendency during lung adenocarcinoma EMT progression. Front Cell Dev Biol. 9:822315. doi: 10.3389/fcell.2021.822315.
- You L, Wang Z, Li H, Shou J, Jing Z, Xie J, Sui X, Pan H, Han W. 2015. The role of STAT3 in autophagy. Autophagy. 11(5):729–739. doi: 10.1080/15548627.2015.1017192.
- Youn DH, Kim Y, Kim BJ, Jeong MS, Lee J, Rhim JK, Kim HC, Jeon JP. 2021. Mitochondrial dysfunction associated with autophagy and mitophagy in cerebrospinal fluid cells of patients with delayed cerebral ischemia following subarachnoid hemorrhage. Sci Rep. 11(1):16512. doi: 10.1038/s41598-021-96092-2.
- Yu W, Liu B, Zhou L, Gong E, Che C, Zhou J, Zhang Z, Liu J, Shi J. 2023. The development of drug delivery systems for efficient intracranial hemorrhage therapy. Adv Healthc Mater. 12(12):e2203141.
- Zhang XW, Wu Y, Wang DK, Jin X, Li CH. 2019. Expression changes of inflammatory cytokines TNF-alpha, IL-1beta and HO-1 in hematoma surrounding brain areas after intracerebral hemorrhage. J Biol Regul Homeost Agents. 33(5):1359–1367. doi: 10.23812/19-150-A.
- Zheng H, Chen C, Zhang J, Hu Z. 2016. Mechanism and therapy of brain edema after intracerebral hemorrhage. Cerebrovasc Dis. 42(3-4):155–169. doi: 10.1159/000445170.
- Zheng S, Jian D, Gan H, Wang L, Zhao J, Zhai X. 2021. FUNDC1 inhibits NLRP3-mediated inflammation after intracerebral hemorrhage by promoting mitophagy in mice. Neurosci Lett. 756:135967. doi: 10.1016/j.neulet.2021.135967.
- Zhou JF, Xiong Y, Kang X, Pan Z, Zhu Q, Goldbrunner R, Stavrinou L, Lin S, Hu W, Zheng F, et al. 2022. Application of stem cells and exosomes in the treatment of intracerebral hemorrhage: an update. Stem Cell Res Ther. 13(1):281. doi: 10.1186/s13287-022-02965-2.
- Zhou ZX, Cui Q, Zhang YM, Yang JX, Xiang WJ, Tian N, Jiang YL, Chen ML, Yang B, Li QH, et al. 2023. Withaferin A inhibits ferroptosis and protects against intracerebral hemorrhage. Neural Regen Res. 18(6):1308–1315. doi: 10.4103/1673-5374.355822.
- Zhu K, Xiaoqiang L, Deng W, Wang G, Fu B. 2021. Development and validation of a novel lipid metabolism-related gene prognostic signature and candidate drugs for patients with bladder cancer. Lipids Health Dis. 20(1):146. doi: 10.1186/s12944-021-01554-1.
- Zhuang J, Cao Y, Guo G, Li M, Zhang T, He D, Chen J, Zhang K, Zhang Z. 2023. Inhibition of BACE1 attenuates microglia-induced neuroinflammation after intracerebral hemorrhage by suppressing STAT3 activation. Aging (Albany NY)). 15(15):7709–7726. doi: 10.18632/aging.204935.
- Zhuo Z, Lin H, Liang J, Ma P, Li J, Huang L, Chen L, Yang H, Bai Y, Sha W. 2021. Mitophagy-related gene signature for prediction prognosis, immune scenery, mutation, and chemotherapy response in pancreatic cancer. Front Cell Dev Biol. 9:802528. doi: 10.3389/fcell.2021.802528.
- Ziai WC, Carhuapoma JR. 2018. Intracerebral hemorrhage. Continuum (Minneap Minn)). 24(6):1603–1622. doi: 10.1212/CON.0000000000000672.
- Ziai WC, Parry-Jones AR, Thompson CB, Sansing LH, Mullen MT, Murthy SB, Mould A, Nekoovaght-Tak S, Hanley DF. 2021. Early inflammatory cytokine expression in cerebrospinal fluid of patients with spontaneous intraventricular hemorrhage. Biomolecules. 11(8):1123. doi: 10.3390/biom11081123.
- Zubkov AY, Ogihara K, Patllola A, Parent AD, Zhang J. 2000. Mitogen-activated protein kinase plays an important role in hemolysate-induced contraction in rabbit basilar artery. Acta Neurochir Suppl. 76:217–221. doi: 10.1007/978-3-7091-6346-7_44.