ABSTRACT
Colibacillosis caused by avian pathogenic Escherichia coli (APEC) is the most common bacterial disease in poultry, resulting in significant economic losses. Resistance to fluoroquinolones has been found to be high in APEC worldwide, which has increased concerns about risks to human health as well as poultry production. In the present study, we determined the prevalence, genetic traits, and fitness traits of fluoroquinolone-resistant APEC isolated from chickens in Korea using a total of 286 APEC isolates collected between 2014 and 2017. The APEC isolates were highly resistant to nalidixic acid (86.0%), ampicillin (71.7%), tetracycline (69.6%), and sulfisoxazole (61.2%), and 132 (46.2%) of the isolates were resistant to both enrofloxacin and ciprofloxacin. These fluoroquinolone-resistant isolates showed eight mutation combinations including single- or double-point mutations in the gyrA, parC, or parE genes. The isolates with double mutations (codons 83 and 87) in gyrA and additional mutations in parC and parE showed high-level fluoroquinolone resistance (minimum inhibitory concentrations, 16–128 µg/ml). The isolates fell into four phylogenetic groups, and groups A (47/132, 35.6%) and B1 (47/132, 36.4%) were the most predominant. Nine isolates (6.8%) belonged to group B2 and included major lineages of extraintestinal pathogenic E. coli, sequence type (ST) 95 (n = 3) and ST69 (n = 2). The isolates varied in their virulence-associated gene content, biofilm formation, and intramacrophage survival. Overall, fluoroquinolone-resistant APEC in poultry poses a potential risk to public health and represents a highly diverse group of the resistant bacteria that varied in their genetic and fitness traits.
Introduction
Avian colibacillosis is a common infectious disease in chickens, turkeys, and other avian species of all ages, caused by Escherichia coli, and it has been associated with heavy economic losses to the poultry industry worldwide (Dziva & Stevens, Citation2008; Lutful Kabir, Citation2010). This colibacillosis in poultry is characterized in its acute form by septicaemia and in its subacute form by airsacculitis, pericarditis, and perihepatitis (Dziva & Stevens, Citation2008; Lutful Kabir, Citation2010). E. coli strains that cause avian colibacillosis are termed avian pathogenic E. coli (APEC; Dziva & Stevens, Citation2008; Lutful Kabir, Citation2010). APEC is known to be genetically closely related to extraintestinal pathogenic E. coli (ExPEC) that causes infections in humans, and APEC-contaminated poultry is suspected to be a foodborne reservoir of ExPEC (Ewers et al., Citation2009; Vincent et al., Citation2010; Bergeron et al., Citation2012; Sarowska et al., Citation2019). Therefore, avian colibacillosis has zoonotic potential as well as an important economic impact on poultry production.
Antimicrobial resistance has been frequently recognized in APEC worldwide and, in particular, fluoroquinolone resistance has increased concerns about risks to human health (Literak et al., Citation2013; Nhung et al., Citation2017). It was reported that APEC isolates from Asia, Africa, America, and Europe showed broad ranges of resistance to the fluoroquinolones, ciprofloxacin (0–91%) and enrofloxacin (3–83%), and 91% of the isolates from Iran and Spain were resistant to ciprofloxacin (Nhung et al., Citation2017). APEC isolates from chickens in Korea showed continuous increases in the level of fluoroquinolone resistance during the period 1985–2005, ranging from 26.7% to 92.2% (Kim et al., Citation2007). The epidemiological relationship between poultry consumption and human disease caused by fluoroquinolone-resistant bacteria resulted in the withdrawal of fluoroquinolones from use in poultry in the USA, Australia, and Northern Europe (Cheng et al., Citation2012; Literak et al., Citation2013; Olkkola et al., Citation2016). In many other parts of the world, including Korea, the prudent use of fluoroquinolones in food-producing animals has been recommended in reduced amounts (Agunos et al., Citation2012; Literak et al., Citation2013).
Fluoroquinolone resistance in E. coli requires multiple genetic changes, including mutations that alter topoisomerases, which facilitate chromosome replication and RNA transcription (Marcusson et al., Citation2009; Redgrave et al., Citation2014). These mutations have impacts that are mostly associated with reduced bacterial fitness (Melnyk et al., Citation2015; Bhatnagar & Wong, Citation2019). However, recent studies suggest that increased resistance to fluoroquinolones in E. coli can be selected with the prevalence of specific resistance alleles among clinical isolates even in the absence of the drug selection pressure (Marcusson et al., Citation2009; Bhatnagar & Wong, Citation2019). Reduced or no fitness costs can be driven by selection of low-cost mutations or by accumulation of secondary fitness-compensating mutations that do not decrease resistance (Marcusson et al., Citation2009; Bhatnagar & Wong, Citation2019). Indeed, fluoroquinolone-resistant Campylobacter jejuni has even shown enhanced fitness in the absence of exposure to the drug, partially explaining its high prevalence on a global scale (Luo et al., Citation2005; Redgrave et al., Citation2014). Selection of fluoroquinolone-resistant Campylobacter in poultry has also been reported to occur and to allow the persistence of the resistant bacteria on farms long after removal of the drug selection pressure (Griggs et al., Citation2005; Luo et al., Citation2005).
It has also been reported that (fluoro)quinolone-resistant E. coli may have increased biofilm-forming and intramacrophage survival abilities that enhance the probability of prolonged survival in infected hosts and environments (Miskinyte & Gordo, Citation2013; Oliveira et al., Citation2014; Nesse et al., Citation2020). APEC isolates are known to carry an arsenal of virulence-associated genes, of which some are proposed to be associated with highly pathogenic APEC (Johnson et al., Citation2008; Jeong et al., Citation2012; Cummins et al., Citation2019). Also, E. coli sequence type 131 (ST131), belonging to phylogenetic group B2, is the most frequent sequence type of E. coli in regard to ExPEC, infections and is known to have genetic changes associated with fluoroquinolone resistance (Doumith et al., Citation2012; Cummins et al., Citation2019). In addition, ST1193 has been reported as an emerging clonal lineage of fluoroquinolone-resistant ExPEC, belonging to group B2, in several countries such as Australia, Germany, USA, China, and Korea (Matsui et al., Citation2020). Thus, certain lineages of E. coli may have a greater capacity to cause APEC infections than others (Johnson et al., Citation2008; Cummins et al., Citation2019). Fluoroquinolone resistance of APEC in chickens has been managed by reducing the use of the drug on farms in Korea as described above. The present study was therefore to examine the prevalence and genetic traits (related to fluoroquinolone resistance, virulence, and phylogeny) of fluoroquinolone-resistant APEC and to determine associations between their resistance-related genetic changes and fitness traits without using the drug.
Materials and methods
Bacterial strains
This study included 286 APEC isolates that are archived by the Avian Disease Research Division at Animal and Plant Quarantine Agency (APQA) in Korea. The isolates were collected from clinical cases of chickens diagnosed with avian colibacillosis by APQA between 2014 and 2017. Their sources were the liver (n = 206), joints (n = 14), infraorbital sinus (n = 14), air sacs (n = 7), peritoneum (n = 7), oviduct (n = 6), subcutaneous tissues (n = 6), lungs (n = 5), yolk sac (n = 5), and others (n = 16). The isolates were identified by conventional biochemical tests (Ewing, Citation1986) or VITEK 2 (bioMerieux Inc., Hazelwood, MO, USA). Only one isolate per case was included in this study to maximize independence between isolates.
Antimicrobial susceptibility testing
Antimicrobial resistance patterns were determined by Kirby-Bauer agar diffusion according to the Clinical and Laboratory Standards Institute guidelines (CLSI, Citation2016) using 18 antimicrobials: ampicillin, amoxicillin-clavulanic acid, cephalothin, cefoxitin, ceftiofur, cefepime, apramycin, gentamicin, streptomycin, neomycin, tetracycline, nalidixic acid, enrofloxacin, ciprofloxacin, trimethoprim-sulfamethoxazole, sulfisoxazole, chloramphenicol, and florfenicol. Enrofloxacin and florfenicol were included in the panel because they are frequently used in chickens in Korea. Minimum inhibitory concentrations (MICs) of nalidixic acid, ciprofloxacin, and enrofloxacin were determined by the agar dilution method and were interpreted according to the CLSI guidelines (CLSI, Citation2015; CLSI, Citation2016). MIC data were expressed as the MIC range and the minimum concentrations at which 50% and 90% of isolates were inhibited (MIC50 and MIC90, respectively).
Analysis of quinolone resistance determinants
The quinolone resistance-determining regions (QRDR) of gyrA, gyrB, parC, and parE genes of fluoroquinolone-resistant APEC isolates were amplified by polymerase chain reaction (PCR) as described previously (Everett et al., Citation1996). PCR products were sequenced on both strands (Macrogen, Seoul, Korea). The sequences were aligned and analyzed using CLC Main Workbench software (CLC Bio, Aarhus, Denmark) to detect mutations in the QRDR of the genes as described previously (Everett et al., Citation1996). Plasmid-mediated quinolone resistance (PMQR) genes including qnrA, qnrB, qnrS, qnrC, qnrD, qepA, aac(6´)-Ib-cr, and oqxAB were detected with a PCR assay as described previously (Ciesielczuk et al., Citation2013). Positive PCR products were sequenced and analyzed as described above. PMQR genes were examined by determining the identity of the sequences using the BLAST programme of National Center for Biotechnology Information (Altschul et al., Citation1997).
Phylogenetic analysis and multilocus sequence typing
Phylogenetic groups (A, B1, B2, and D) of all fluoroquinolone-resistant APEC isolates were determined based on the presence of the chuA, yjaA, and TSpE4 by the multiplex PCR assay as described previously (Doumith et al., Citation2012). Multilocus sequence typing (MLST) on isolates belonging to group B2 was performed by amplification and sequencing of seven house keeping genes including adk, fumC, gyrB, icd, mdh, purA, and recA as described previously (Wirth et al., Citation2006). Sequence types were assigned according to the scheme of the E. coli MLST website (http://enterobase.warwick.ac.uk/species/index/ecoli).
Detection of virulence-associated genes
The presence of virulence-associated genes implicated in adhesion (fimC and tsh), iron acquisition (incD, fyuA, irp2, and iroN), haemolysis (hlyF), resistance to bactericidal factors (iss and ompT), and toxin production (lt, st, and vat) in the fluoroquinolone-resistant APEC isolates was determined by PCR as described previously (Jeong et al., Citation2012).
Biofilm formation assay
Isolates were assayed for biofilm formation in microtitre plate wells following previously described procedures (Coffey & Anderson, Citation2014). Briefly, an overnight culture of each isolate was transferred as a 1:10 dilution into LB broth (Life Technologies, Carlsbad, CA, USA). The culture diluted in LB broth was then aliquoted into eight replicate wells (100 µl each) in a 96-well polystyrene microtitre plate (Costar, Corning, NY, USA). Eight wells with LB broth only were included as a control. After inoculation, plates were sealed and incubated statically at 30°C for 48 h. Liquid was then removed from each well, and the plates were washed three times with 200 µl of sterile distilled water to remove loosely adherent bacteria. Plates were air-dried at room temperature for 15 min, and then attached cells were stained with 150 µl of a 1% solution of crystal violet (CV) per well for 15 min. Plates were washed again three times with sterile distilled water to remove unbound dye. The remaining CV was dissolved in 200 µl of 95% ethanol per well. The relative amount of CV from each well was measured at 600 nm with a microtitre reader (Molecular Devices, Sunnyvale, CA, USA).
Intramacrophage survival assay
Isolates were tested for their intraphagocytic survival abilities with the chicken macrophage cell line (HD11) as described previously with some modifications (Chadfield et al., Citation2003; Zhuge et al., Citation2019). For the survival assay, HD11 cells were seeded in a 24-well tissue culture tray at 105 cells/well with Iscove’s Modified Dulbecco’s Medium (IMDM; Sigma Aldrich, MO, USA) containing 10% foetal bovine serum and 150 mM L-glutamine and grown overnight at 41°C in a 5% CO2 atmosphere. The log-phase bacterial culture was opsonized with 10% chicken serum at 37°C for 30 min. The opsonized culture was added to the HD11 cells at an MOI of 10. The bacteria were incubated with cells for 1 h, and then the medium was replaced with IMDM with 10% FBS and 150 mM L-glutamine containing amikacin (100 µg/ml) and incubated for 1 h to kill extracellular bacteria. The cells were then washed three times with antibiotic-free medium. The initial count of intracellular bacteria (1-h survival) was made by lysing cells with 0.1% Triton X-100 in PBS and then plating the lysate onto LB agar (Life Technologies). To determine the persistence of bacteria, cells were maintained in medium containing amikacin (10 μg/ml). At 4 and 24 h post-infection the monolayers were washed three times with PBS and the cells were lysed with 0.1% Triton X-100 in PBS. Released bacteria were quantified to determine the percentage recovery relative to inoculated bacteria.
Statistical analysis
Biofilm formation and intramacrophage survival assay results were compared to test differences between fluoroquinolone-resistant APEC isolates of each QRDR mutant group and the pansusceptible APEC isolate using Student’s t test. Test results with P < 0.05 were considered to be statistically significant.
Results
Antimicrobial resistance of APEC isolates
All 286 APEC isolates tested showed high resistance to multiple antimicrobials. The isolates showed the highest resistance against nalidixic acid (98.0%), followed by ampicillin (71.7%), tetracycline (69.9%), and sulfisoxazole (61.2%). They were also highly resistant to fluoroquinolones, enrofloxacin (60.1%) and ciprofloxacin (54.9%) ().
Figure 1. Antimicrobial resistance of 286 APEC isolates collected from clinical cases between 2014 and 2018. The isolates showed the highest resistance against nalidixic acid (98.0%), followed by ampicillin (71.7%), tetracycline (69.9%), sulfisoxazole (61.2%), enrofloxacin (60.1%), and ciprofloxacin (54.9%). Abbreviations: ampicillin, AMP; amoxicillin-clavulanic acid, AMC; cephalothin, CEF; cefoxitin, FOX; ceftiofur, XNL; cefepime, FEP; apramycin, APR; gentamicin, GEN; streptomycin, STR; neomycin, NEO; tetracycline, TET; nalidixic acid, NAL; enrofloxacin, ENR; ciprofloxacin, CIP; trimethoprim-sulphamethoxazole, SXT; sulfisoxazole, FIS; chloramphenicol, CHL; florfenicol, FFC.
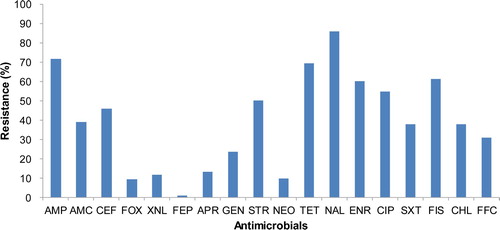
One hundred and thirty-two isolates of fluoroquinolone-resistant APEC were selected and tested for MICs of the quinolones nalidixic acid, ciprofloxacin, and enrofloxacin. The range of MICs of the quinolones classified as resistant is indicated by light grey shading in . MIC50 and MIC90 against the isolates were >512 μg/ml (range: 32–>512 μg/ml) for nalidixic acid, 16 and 64 µg/ml (range: 2–128 µg/ml) for enrofloxacin, and 16 and 32 µg/ml (range: 0.5–128 µg/ml) for ciprofloxacin, respectively ( and ).
Table 1. The MIC distribution of quinolones against 132 isolates of fluoroquinolone-resistant APEC.
Table 2. Mutations in gyrA, parC, and parE of 132 isolates of fluoroquinolone-resistant APEC.
QRDRS and PMQRS of fluoroquinolone-resistant APEC isolates
Fluoroquinolone-resistant isolates revealed eight combinations of mutations in gyrA, parC, and parE including single- or double-point mutations at their QRDRs (). One hundred and twenty-nine isolates (97.7%) showed a substitution of serine to leucine at codon 83 (S83L) in gyrA, and 115 (81.7%) had a substitution of aspartic acid to asparagine at codon 87 (D87N) in gyrA (). At QRDRs in parC, a substitution of serine to isoleucine at codon 80 (S80I) was the most predominant (89.4%) in the isolates, and only 20 isolates (15.2%) showed a substitution at codons 458, 460, or 464 in parE (). Eighty-five isolates (64.4%) had two substitutions of S83L and D87N in gyrA and a substitution of S80I in parC and comprised the most prevalent mutant group (D) (). The isolates that had double mutations at codons 83 and 87 in gyrA and additional mutations in parC and parE were highly resistant to fluoroquinolones, showing ciprofloxacin MICs ranging from 16 to 128 µg/ml and enrofloxacin MICs from 32 to 128 µg/ml ().
The PMQR gene qnrS1 was detected in nine (6.8%) of the isolates tested, while other genes including qnrA, qnrB, qnrC, qnrD, qepA, aac(6´)-1b-cr, and oqxAB were all PCR-negative (). PMRQ-positive isolates showed ciprofloxacin MICs ranging from 2 to 64 µg/ml and enrofloxacin MICs from 4 to 128 µg/ml, and they had similar or slightly (approximately twofold) increased levels of fluoroquinolone resistance compared with qniS1-negative isolates of the same mutant group (). Six qnrS1-positive isolates detected were also resistant to β-lactamase inhibitor, amoxicillin-clavulanic acid, or a third-generation cephalosporin, ceftiofur ().
Table 3. The distribution of quinolone MICs and mutations in gyrA, parC, and parE of nine APEC isolates harbouring the PMQR gene qnrS1.
Phylogenetic groups, sequence types, and virulence-associated gene content of fluoroquinolone-resistant APEC isolates
All 132 fluoroquinolone-resistant APEC isolates were divided into four phylogenetic groups A, B1, B2, and D. Groups A and B1 were the most predominant, reaching 35.6% (47/132) and 36.4% (48/132), respectively. The most prevalent mutant group D included all four phylogenetic groups A (37.6%), B1 (36.5%), B2 (2.4%), and D (23.5%). The other isolates were grouped into group B2 (6.8%, 9/132) or D (21.2%, 28/132). Sequence types of nine isolates belonging to group B2 included ST95 (3/9, 33.3%), ST69 (2/9, 22.2%), ST57 (3/9, 33.3%), and ST770 (1/9, 11.1%). The virulence-associated genes, which were highly prevalent in the isolates, included hlyF (97.0%), iucD (95.5%), iss (94.7%), fimC (93.9%), ompT (80.3%), and iroN (78.0%). The frequencies of the genes irp2, tsh, fyuA, vat, st, and lt were 56.1%, 53.8%, 33.3%, 13.6%, 3.0%, and 0%, respectively. The isolates showed 48 different combinations of the genes with the most common types fimC-iroN-irp2-iucD-fyuA-tsh-hlyF-ompT-iss (11.4%, 15/132), fimC-iroN-irp2-iucD-tsh-hlyF-ompT-iss (10.6%, 14/132), and fimC-iroN-iucD-tsh-hlyF-ompT-iss (9.8%, 13/132). These three common types were found in all four groups A, B1, B2, and D. The phylogenetic groups and virulence-associated gene contents of representative fluoroquinolone-resistant isolates of APEC are presented in .
Table 4. Antimicrobial resistance patterns and virulence-associated gene contents of representative fluoroquinolone-resistant isolates of APEC used in biofilm formation and intramacrophage survival assays.
Biofilm formation and intramacrophage survival of fluoroquinolone-resistant APEC isolates
Twenty-two isolates of fluoroquinolone-resistant APEC representing eight mutant groups (A to H) with different combinations of mutations in the QRDRs and including one wild type (I) were tested for their biofilm formation and intramacrophage survival abilities. One pansusceptible APEC isolate (1582) was included in the assays as a control. Assay data of isolates of each mutant group were averaged for comparison. Although isolates of mutant groups C and F formed relatively robust biofilms in polystyrene plates 24 h post-incubation compared with the pansusceptible isolate (P < 0.05), there were no significant differences between other groups and the pansusceptible isolate (). The isolates were able to persist in the HD11 cells with variation, but there was no significant difference in survival rates examined periodically between the isolates of each mutant group and the pansusceptible isolate ().
Figure 2. Biofilm formation of fluoroquinolone-resistant and pansusceptible isolates of APEC. Each isolate was tested by a crystal violet assay using polystyrene microtitre plates with LB broth. Bars show averaged biofilm levels from two independent experiments and error bars represent standard errors. Although isolates of mutant groups C and F formed relatively robust biofilms 24 h post-incubation compared with the pansusceptible isolate (*P < 0.05), overall, their biofilm levels were low, and there was no significant difference between fluoroquinolone-resistant and pansusceptible isolates.
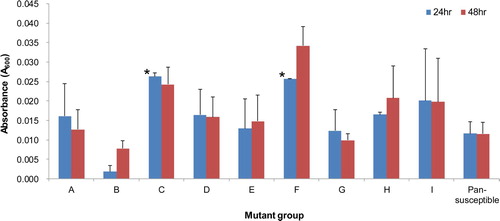
Figure 3. Intramacrophage survival of fluoroquinolone-resistant and pansusceptible isolates of APEC in the chicken macrophage cell line HD11. Bars represent the ratio (%) of intracellular bacteria to inoculated bacteria that was determined based on three independent experiments, and error bars indicate standard errors. The isolates were able to survive in the HD11 cells with variation, but there was no significant difference in intracellular survival between fluoroquinolone-resistant and pansusceptible isolates.
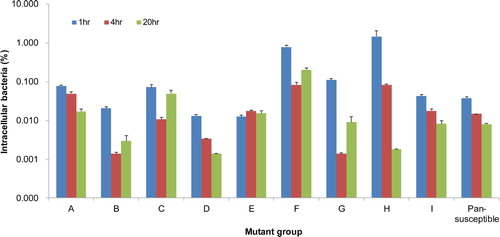
Discussion
Fluoroquinolone resistance of pathogenic bacteria in poultry has significant implications for public health as well as poultry production. Inappropriate use of fluoroquinolones on poultry farms may have facilitated the development of the resistant bacteria, and the discontinued or prudent use of fluoroquinolones in poultry production has been highly recommended to manage the levels of fluoroquinolone-resistant bacteria worldwide. APEC isolates tested in the present study showed high resistance to fluoroquinolones, enrofloxacin (60.1%) and ciprofloxacin (54.9%). They also had broad ranges of MIC reaching 128 µg/ml for both enrofloxacin and ciprofloxacin. These fluoroquinolone resistance rates of APEC were lower than the 92.2% between 2000 and 2005 reported previously (Kim et al., Citation2007), but they remain high in Korea even though a veterinarian’s prescription has been required for using the drug since 2013.
Fluoroquinolone resistance-associated genetic changes were identified in QRDRs in gyrA, parC, and parE in this study, revealing eight combinations of mutations in the three genes including single- or double-point mutations at their QRDRs. The mutants harbouring two substitutions of S83L and D87N in gyrA and a substitution of S80I in parC (mutant group D) were the most prevalent, accounting for 64.4% of fluoroquinolone-resistant APEC isolates tested. Additional mutations, E84G and S458A in parC and parE, respectively, were associated with a further increase in fluoroquinolone resistance based on MIC ranges. The PMQR gene qnrS1 was detected in nine (6.8%) of the fluoroquinolone-resistant isolates tested, and the isolates harbouring the gene had similar or slightly greater fluoroquinolone resistance than the qnrS1-negative isolates of the same mutant group, similar to previous findings (Rodriguez-Martinez et al., Citation2016; Nishikawa et al., Citation2019). Most qnrS1-positive isolates detected were also resistant to β-lactamase inhibitor (clavulanic acid) or a third-generation cephalosporin (ceftiofur). Previous studies showed that PMQR genes might be co-located on extended-spectrum β-lactamase (ESBL) plasmids, resulting in fluoroquinolone and ESBL co-resistance in bacteria (Rodriguez-Martinez et al., Citation2016; Davies & Wales, Citation2019). Although the frequency of this co-resistance in APEC is low, this potential genetic linkage should be considered to control fluoroquinolone resistance in poultry.
Researchers suggested there was a close association between increased virulence in APEC and the possession of ColV plasmids carrying virulence genes such as iutA, hlyF, iss, iroN, and ompT (Johnson et al., Citation2008; Cummins et al., Citation2019). Furthermore, a clone of fluoroquinolone-resistant APEC such as ST354 was identified in Australia, indicating the clonal spread of fluoroquinolone resistance-related mutations (Cummins et al., Citation2019). Also, clonal lineages of fluoroquinolone-resistant ExPEC (ST131 and ST1193) belonging to phylogenetic group B2 have been reported in many parts of the world (Doumith et al., Citation2012; Cummins et al., Citation2019; Matsui et al., Citation2020). In this study, we tested fluoroquinolone-resistant APEC isolates for the carriage of 12 virulence-associated genes. The genes hlyf, iucD, iss, and fimC were most prevalent in fluoroquinolone-resistant APEC isolates, accounting for more than 90%, and the isolates were highly variable in the carriage of all genes tested. Phylogenetic analysis revealed that groups A (35.6%) and B1 (36.4%) were the most predominant in the isolates, similarly to previous reports with APEC isolates, regardless of fluoroquinolone resistance (Johnson et al., Citation2008; Jeong et al., Citation2012). Group B2, which is the most frequent in ExPEC, was rarely found, accounting for 6.8%, while group D was 21.2%. Nevertheless, more than half of the isolates belonging to group B2 were ST95 and ST69 that were included in major lineages associated with bloodstream infections and urinary tract infections in humans (Ewers et al., Citation2009; Riley, Citation2014; Vincent et al., Citation2010). The most prevalent mutant group D included all four phylogenetic groups at rates similar to those in all isolates tested. There was no clear relationship between virulence-associated gene contents and phylogenetic background, and they were not significant contributors to the prevalence of fluoroquinolone resistance-related mutation types.
In this study, the isolates representing each mutant group and a pansusceptible isolate were tested for their biofilm forming and intramacrophage survival abilities, that is, allowing resistance in hostile environments within the host. In those assays, the isolates of each mutant group showed various levels of biofilm-forming and intramacrophage survival abilities, and the resistant isolates did not have better survival fitness than the pansusceptible isolate. In previous studies, the biofilm-forming ability appeared to influence the environmental persistence and phagocytic survival of fluoroquinolone-resistant E. coli (Oliveira et al., Citation2014; Nesse et al., Citation2020). Researchers suspect that biofilm production is associated with the persistence and dissemination of fluoroquinolone-resistant E. coli in the poultry production chain even if fluoroquinolones are not administered to poultry (Nesse et al., Citation2020). However, fluoroquinolone-resistant APEC isolates tested in this study did not produce any meaningful data on their biofilm formation and intramacrophage survival distinguishing specific mutant or phylogenetic groups.
Previous researchers showed that introducing the S80I mutation in parC into the E. coli mutant harbouring S83L and D87N in gyrA increased its resistance to fluoroquinolones and its competitive fitness versus the wild type in the absence of the drug (Marcusson et al., Citation2009). Similarly, mutations including S83L and D87N in gyrA and S80I in parC were the most prevalent in this study, suggesting they could be selected for improved fitness and reduced susceptibility to fluoroquinolones. Although fitness traits of the fluoroquinolone-resistant APEC isolates varied, the major mutant group may be persistent in poultry even without use of the drug.
In the present study, high levels of fluoroquinolone resistance in APEC were mediated by mutations in gyrA, parC, and parE and the acquisition of PMQR, at least qnrS1. Also, major QRDR mutant groups and clonal lineages of ExPEC were identified in the APEC isolates. However, the virulence-associated gene content, phylogenetic background, and biological fitness of the isolates were not major factors contributing to the high prevalence of the fluoroquinolone-resistance phenotype. Possible co-resistance, such as to fluoroquinolone and ESBL, should be considered together to control fluoroquinolone-resistant APEC in poultry. Overall, the findings of this study indicate that fluoroquinolone-resistant APEC in poultry may increase the potential risk to public health, and its high genetic and fitness diversity may complicate the control of the resistance despite strict restrictions on use of the drug.
Acknowledgement
We thank Su-Jeong Kim and Yu-Jin Lee for technical assistance.
Disclosure statement
No potential conflict of interest was reported by the authors.
Additional information
Funding
References
- Agunos, A., Leger, D. & Carson, C. (2012). Review of antimicrobial therapy of selected bacterial diseases in broiler chickens in Canada. The Canadian Veterinary Journal, 53, 1289–1300.
- Altschul, S.F., Madden, T.L., Schaffer, A.A., Zhang, J., Zhang, Z., Miller, W. & Lipman, D.J. (1997). Gapped BLAST and PSI-BLAST: a new generation of protein database search programs. Nucleic Acids Research, 25, 3389–3402.
- Bergeron, C.R., Prussing, C., Boerlin, P., Daignault, D., Dutil, L., Reid-Smith, R.J., Zhanel, G.G. & Manges, A.R. (2012). Chicken as reservoir for extraintestinal pathogenic Escherichia coli in humans, Canada. Emerging Infectious Diseases, 18, 415–421.
- Bhatnagar, K. & Wong, A. (2019). The mutational landscape of quinolone resistance in Escherichia coli. PLoS One, 14, e0224650.
- Chadfield, M.S., Brown, D.J., Aabo, S., Christensen, J.P. & Olsen, J.E. (2003). Comparison of intestinal invasion and macrophage response of Salmonella Gallinarum and other host-adapted Salmonella enterica serovars in the avian host. Veterinary Microbiology, 92, 49–64.
- Cheng, A.C., Turnidge, J., Collignon, P., Looke, D., Barton, M. & Gottlieb, T. (2012). Control of fluoroquinolone resistance through successful regulation, Australia. Emerging Infectious Diseases, 18, 1453–1460.
- Ciesielczuk, H., Hornsey, M., Choi, V., Woodford, N. & Wareham, D.W. (2013). Development and evaluation of a multiplex PCR for eight plasmid-mediated quinolone-resistance determinants. Journal of Medical Microbiology, 62, 1823–1827.
- CLSI (Clinical and Laboratory Standards Institute). (2015). Performance standards for antimicrobial disk and dilution susceptibility tests for bacteria isolated from animals. 3rd ed. CLSI supplement VET01S. In. Wayne, PA: CLSI.
- CLSI (Clinical and Laboratory Standards Institute). (2016). Performance standards for antimicrobial susceptibility testing, 26th ed. Approved standard, CLSI publication M100-S26. In. Wayne, PA: CLSI.
- Coffey, B.M. & Anderson, G.G. (2014). Biofilm formation in the 96-well microtiter plate. Methods in Molecular Biology, 1149, 631–641.
- Cummins, M.L., Reid, C.J., Chowdhury, P.R., Bushell, R.N., Esbert, N., Tivendale, K.A., Noormohammadi, A.H., Islam, S., Marenda, M.S., Browning, G.F., Markham, P.F. & Djordjevic, S.P. (2019). Whole genome sequence analysis of Australian avian pathogenic Escherichia coli that carry the class 1 integrase gene. Microbial Genomics, 5, e000250.
- Davies, R.D. & Wales, A. (2019). Antimicrobial resistance on farms: a review including biosecurity and the potential role of disinfectants in resistance selection. Comprehensive Reviews in Food Science and Food Safety, 18, 753–774.
- Doumith, M., Day, M.J., Hope, R., Wain, J. & Woodford, N. (2012). Improved multiplex PCR strategy for rapid assignment of the four major Escherichia coli phylogenetic groups. Journal of Clinical Microbiology, 50, 3108–3110.
- Dziva, F. & Stevens, M.P. (2008). Colibacillosis in poultry: unravelling the molecular basis of virulence of avian pathogenic Escherichia coli in their natural hosts. Avian Pathology, 37, 355–366.
- Everett, M.J., Jin, Y.F., Ricci, V. & Piddock, L.J. (1996). Contributions of individual mechanisms to fluoroquinolone resistance in 36 Escherichia coli strains isolated from humans and animals. Antimicrobial Agents and Chemotherapy, 40, 2380–2386.
- Ewers, C., Antao, E.M., Diehl, I., Philipp, H.C. & Wieler, L.H. (2009). Intestine and environment of the chicken as reservoirs for extraintestinal pathogenic Escherichia coli strains with zoonotic potential. Applied and Environmental Microbiology, 75, 184–192.
- Ewing, W.H. (1986). Edwards and Ewing's identification of Enterobacteriaceae. New York, N.Y.: Elsevier Science Publishing Co. Inc.
- Griggs, D.J., Johnson, M.M., Frost, J.A., Humphrey, T., Jorgensen, F. & Piddock, L.J. (2005). Incidence and mechanism of ciprofloxacin resistance in Campylobacter spp. isolated from commercial poultry flocks in the United Kingdom before, during, and after fluoroquinolone treatment. Antimicrobial Agents and Chemotherapy, 49, 699–707.
- Jeong, Y.W., Kim, T.E., Kim, J.H. & Kwon, H.J. (2012). Pathotyping avian pathogenic Escherichia coli strains in Korea. Journal of Veterinary Science, 13, 145–152.
- Johnson, T.J., Wannemuehler, Y., Doetkott, C., Johnson, S.J., Rosenberger, S.C. & Nolan, L.K. (2008). Identification of minimal predictors of avian pathogenic Escherichia coli virulence for use as a rapid diagnostic tool. Journal of Clinical Microbiology, 46, 3987–3996.
- Kim, T.E., Jeong, Y.W., Cho, S.H., Kim, S.J. & Kwon, H.J. (2007). Chronological study of antibiotic resistances and their relevant genes in Korean avian pathogenic Escherichia coli isolates. Journal of Clinical Microbiology, 45, 3309–3315.
- Literak, I., Reitschmied, T., Bujnakova, D., Dolejska, M., Cizek, A., Bardon, J., Pokludova, L., Alexa, P., Halova, D. & Jamborova, I. (2013). Broilers as a source of quinolone-resistant and extraintestinal pathogenic Escherichia coli in the Czech Republic. Microbial Drug Resistance, 19, 57–63.
- Luo, N., Pereira, S., Sahin, O., Lin, J., Huang, S., Michel, L. & Zhang, Q. (2005). Enhanced in vivo fitness of fluoroquinolone-resistant Campylobacter jejuni in the absence of antibiotic selection pressure. Proceedings of the National Academy of Sciences, 102, 541–546.
- Lutful Kabir, S.M. (2010). Avian colibacillosis and salmonellosis: a closer look at epidemiology, pathogenesis, diagnosis, control and public health concerns. International Journal of Environmental Research and Public Health, 7, 89–114.
- Marcusson, L.L., Frimodt-Moller, N. & Hughes, D. (2009). Interplay in the selection of fluoroquinolone resistance and bacterial fitness. PLoS Pathogens, 5, e1000541.
- Matsui, Y., Hu, Y., Rubin, J., de Assis, R.S., Suh, J. & Riley, L.W. (2020). Multilocus sequence typing of Escherichia coli isolates from urinary tract infection patients and from fecal samples of healthy subjects in a college community. MicrobiologyOpen, 9, e1032.
- Melnyk, A.H., Wong, A. & Kassen, R. (2015). The fitness costs of antibiotic resistance mutations. Evolutionary Applications, 8, 273–283.
- Miskinyte, M. & Gordo, I. (2013). Increased survival of antibiotic-resistant Escherichia coli inside macrophages. Antimicrobial Agents and Chemotherapy, 57, 189–195.
- Nesse, L.L., Osland, A.M., Mo, S.S., Sekse, C., Slettemeas, J.S., Bruvoll, A.E.E., Urdahl, A.M. & Vestby, L.K. (2020). Biofilm forming properties of quinolone resistant Escherichia coli from the broiler production chain and their dynamics in mixed biofilms. BMC Microbiology, 20, 46.
- Nhung, N.T., Chansiripornchai, N. & Carrique-Mas, J.J. (2017). Antimicrobial resistance in bacterial poultry pathogens: a review. Frontiers in Veterinary Science, 4, 126.
- Nishikawa, R., Murase, T. & Ozaki, H. (2019). Plasmid-mediated quinolone resistance in Escherichia coli isolates from commercial broiler chickens and selection of fluoroquinolone-resistant mutants. Poultry Science, 98, 5900–5907.
- Oliveira, M., Dias, F.R. & Pomba, C. (2014). Biofilm and fluoroquinolone resistance of canine Escherichia coli uropathogenic isolates. BMC Research Notes, 7, 499.
- Olkkola, S., Nykasenoja, S., Raulo, S., Llarena, A.K., Kovanen, S., Kivisto, R., Myllyniemi, A.L. & Hanninen, M.L. (2016). Antimicrobial resistance and multilocus sequence types of Finnish Campylobacter jejuni isolates from multiple sources. Zoonoses and Public Health, 63, 10–19.
- Redgrave, L.S., Sutton, S.B., Webber, M.A. & Piddock, L.J. (2014). Fluoroquinolone resistance: mechanisms, impact on bacteria, and role in evolutionary success. Trends in Microbiology, 22, 438–445.
- Riley, L.W. (2014). Pandemic lineages of extraintestinal pathogenic Escherichia coli. Clinical Microbiology and Infection, 20, 380–390.
- Rodriguez-Martinez, J.M., Machuca, J., Cano, M.E., Calvo, J., Martinez-Martinez, L. & Pascual, A. (2016). Plasmid-mediated quinolone resistance: two decades on. Drug Resistance Updates, 29, 13–29.
- Sarowska, J., Futoma-Koloch, B., Jama-Kmiecik, A., Frej-Madrzak, M., Ksiazczyk, M., Bugla-Ploskonska, G. & Choroszy-Krol, I. (2019). Virulence factors, prevalence and potential transmission of extraintestinal pathogenic Escherichia coli isolated from different sources: recent reports. Gut Pathogens, 11, 10.
- Vincent, C., Boerlin, P., Daignault, D., Dozois, C.M., Dutil, L., Galanakis, C., Reid-Smith R.J., Tellier, P.P., Tellis, P.A., Ziebell, K. & Manges, A.R. (2010). Food reservoir for Escherichia coli causing urinary tract infections. Emerging Infectious Diseases, 16, 88–95.
- Wirth, T., Falush, D., Lan, R., Colles, F., Mensa, P., Wieler, L.H., Karch, H., Reeves, P.R., Maiden, M.C., Ochman, H. & Achtman, M. (2006). Sex and virulence in Escherichia coli: an evolutionary perspective. Molecular microbiology, 60(5), 1136–1151.
- Zhuge, X., Sun, Y., Jiang, M., Wang, J., Tang, F., Xue, F., Ren, J., Zhu, W. & Dai, J. (2019). Acetate metabolic requirement of avian pathogenic Escherichia coli promotes its intracellular proliferation within macrophage. Veterinary Research, 50, 31.